ABSTRACT
Asparagus (Asparagus officinalis) is a crop with medicinal, horticultural, and nutritional uses. The basic leucine zipper (bZIP) family is a transcription factor family distributed throughout eukaryotes, including plants, and whose members participate in various biological processes, including plant growth, development, flowering, and stress responses. However, a few genome-wide studies of the bZIP family members have been reported. Here, 46 Asparagus bZIP members, named AobZIP01–AobZIP46, were identified from the reference genome via PFAM search and could be divided into 14 subgroups which were shown to be similar via motif and gene structure analysis. The molecular evolution, motif, and gene structure comparative analysis between A. officinalis and Arabidopsis indicated the accuracy of AobZIP member identification. Additionally, the cis-acting elements of the AobZIP members revealed that they might be associated with plant hormones and responses to abiotic stress. The collinear analysis predicted that the function of AobZIP members might be comparable to that of other species. Kyoto Encyclopedia of Genes and Genomes (KEGG) enrichment analysis showed that AobZIP genes were enriched in the abscisic acid (ABA) pathway. Furthermore, the tissue-specific expression at the seedling stage revealed that root tissue could be used as a target tissue because of its high expression level. The expression of AobZIP genes determined by quantitative real-time PCR under abiotic stress at the seeding stage revealed that some AobZIP members could be candidate genes for plant breeding. This study offers insights for future research in improving the abiotic stress resistance of asparagus utilizing the AobZIP genes.
Introduction
Transcription factors are proteins that regulate the transcription of other genes and comprise approximately 8% of the protein-coding genes in eukaryotic genomes (Pruneda-Paz et al. Citation2014). TFs have highly specific sequences (Pan et al. Citation2010), and the structure of most plant TFs includes an oligomerization site, a DNA-binding region, a transcription-regulation domain, and a nuclear localization signal (Liu et al. Citation1999). The binding of TFs to their DNA binding sites is a key step of gene expression regulation (Turner et al. Citation2012). Therefore, functional characterization of TFs is critical for understanding the transcriptional regulatory networks (Liu et al. Citation2014). The basic leucine zipper (bZIP) TF family is among the largest and most diverse TF families, and its members participate in plant growth, development, and abiotic stress responses (Pérez-Rodríguez et al. Citation2010). The bZIP TF members have a highly conserved 40–80 amino acid sequence called the bZIP domain, consisting of two structural features located on a contiguous α-helix (Jakoby et al. Citation2002). These two features comprise a basic region of 16 amino acid residues with a nuclear signal followed by an N-x7-R/K motif and a heptad repeat of leucines or other bulky hydrophobic amino acids that are located nine residues from the C-terminus (Izawa et al. Citation1993). These two subunits adhere through interactions between the hydrophobic sides of their helices and create a superimposing coiled-coil structure (Pérez-Rodríguez et al. Citation2010). Besides their bZIP domain, some domains of the bZIP members also bind the DNA (Liao et al. Citation2008) to form structures (Ellenberger et al. Citation1992).
Some bZIP TF members have been reported to participate in plant growth and developmental processes, including light signaling, seed maturation, flower development, resisting abiotic stress, and ensuring pathogen defense during plant growth (Jakoby et al. Citation2002; Toh et al. Citation2012). The bZIP genes were involved in explant browning in walnut (Wang et al. Citation2023). AtbZIP11, a bZIP member in Arabidopsis, regulates root development (Weiste et al. Citation2017), and its overexpression in transgenic Arabidopsis significantly affected amino acid contents (Thalor et al. Citation2012; Sagor et al. Citation2016). SlbZIP1 is a Solanum lycopersicum bZIP member, and its overexpression up-regulated the expression of sucrose phosphate synthase genes and increased the sugar content in tomato plants (Thalor et al. Citation2012). Furthermore, GbbZIP08 regulates flavonoid biosynthesis in Ginkgo biloba (Han et al. Citation2023), while CAREB1 regulates somatic embryogenesis in Daucus carota (Guan et al. Citation2009). ZmbZIP4 is a bZIP gene in Zea mays, and its overexpression in transgenic maize plants promoted abscisic acid (ABA) synthesis and the formation of more lateral roots and longer primary roots under abiotic stress (Ma et al. Citation2018). SlAREB1, a bZIP transcription factor in Solanum lycopersicum, responds to low temperatures to regulate anthocyanin biosynthesis (Xu et al. Citation2023). Moreover, ELONGATED HYPOCOTYL5 (HY5) is a bZIP transcription factor family member involved in biological processes such as hormone signaling, nutrient uptake, and abiotic stress responses (salt and cold) and promotes pigment accumulation in Arabidopsis (Ma et al. Citation2018). The bZIP transcription factor PERIANTHIA is highly expressed in developing flowers, where it regulates the formation of perianth organs (Maier et al. Citation2011). Overexpressing bZIP transcription factor GmbZIP19 in transgenic Arabidopsis affected the tolerance of plants to pathogens, drought, and salt stress (He et al. Citation2020). Another soybean bZIP member, GmbZIP15, has been shown to negatively regulate salt and drought stresses (Zhang et al. Citation2020a). Some bZIP members function in protein degradation, protein–protein interactions and intracellular partitioning (Hu et al. Citation2002; Schütze et al. Citation2008). S1-bZIPs were found to directly regulate the expression of PROLINE DEHYDROGENASE (ProDH) and ASPARAGINE SYNTHETASE1 (ASN1), thus affecting amino acid metabolism (Dietrich et al. Citation2011). AtbZIP53, a bZIP member in Arabidopsis, has been shown to target ProDH2 to alleviate carbon, nitrogen, and energy deficiencies (Satoh et al. Citation2004; Hartmann et al. Citation2015). OsABF1 is a bZIP member in rice (Oryza sativa) that activates COR413-TM1 transcription to improve drought tolerance (Zhang et al. Citation2017).
Several plant reference genomes have been obtained with advancing research technologies, enabling the analysis of bZIP family members. Thus, more bZIP members have been identified, including those in Arabidopsis (Jakoby et al. Citation2002), maize (Zea Mays) (Wei et al. Citation2012), soybean (Glycine max) (Yang et al. Citation2020), Chinese jujube (Ziziphus jujuba) (Zhang et al. Citation2020b), olive (Olea europaea) (Rong et al. Citation2020), potato (Solanum tuberosum) (Herath and Verchot Citation2020), common bean (Phaseolus vulgaris) (Zhang et al. Citation2022b), and adzuki bean (Vigna Angularis) (Yin et al. Citation2022). All these results had showed that although bZIP members had a variable numbers while some characteristics had been found in bZIP members such as responding to abiotic stresses (Baillo et al. Citation2019; Liu et al. Citation2023). However, the bZIP members in asparagus (Asparagus officinalis) have not been explored. Asparagus is a vegetable belonging to the Asparagaceae family and has abundant phytochemicals with important bioactivities. With the advance of sequencing technology in recent years, genomic information on asparagus, including the mitochondrial genome (Sheng Citation2020; Sheng et al. Citation2023), chloroplast genome (Kuang and Sheng Citation2022; Sheng Citation2022), and the whole-genome (Tsugama and Fujino Citation2019), have been published, which provides signatures of evolutionary complexity and informativity in sex chromosome evolution, gives detailed information of asparagus and acceleratees the breeding process of asparagus (Kuhl et al. Citation2005; Li et al. Citation2019a; Plath et al. Citation2022). Here, a genome-wide identification was conducted to identify the bZIP members, and the bZIP members were comprehensively analyzed, providing a foundation for future research and breeding of Asparagus officinalis.
Materials and methods
Identification and evolutionary analysis of bZIP members
The reference genome (including DNA, RNA, cDNA, and protein sequences) of asparagus (Asparagus officinalis) was obtained from the National Center for Biotechnology Information (NCBI) database. A hidden Markov model (PF00170) of the bZIP domain was used to search the bZIP genes in the PFAM database, with the filter conditions set to PFAM 35.0 (Finn et al. Citation2015). SMART (Letunic et al. Citation2015) and InterPro (Finn et al. Citation2017) tools were used to screen the identified members, and the members obtained after filtering were identified as the AobZIP proteins and were numbered according to chromosomal location. The locations of AobZIP members were mapped using TBtools (Chen et al. Citation2020).
The gene tree of the bZIP members of asparagus and Arabidopsis protein sequences was constructed via the maximum likelihood method using MEGA X with the LG + G model and 1000 bootstrap replicates (Kumar et al. Citation2018). The evolutionary tree was generated using TBtools.
The analysis of AobZIP proteins
The motifs of putative AobZIP proteins were analyzed using MEME (Bailey et al. Citation2009) with a motif length of 10–50 amino acids and an e-value of less than 1e−20. The Gene Structure Display Server (GSDS) was used to analyze the gene structure of the AobZIP members (Hu et al. Citation2015), including their untranslated region (UTR), coding sequences (CDS), and introns. PlantCARE was used to analyze the cis-acting elements of the AobZIP members to determine the abiotic stress-related and hormone-related elements for subsequent analysis (Lescot et al. Citation2002). MCScanX was used to analyze collinearity and gene duplication events (Krzywinski et al. Citation2009; Wang et al. Citation2013). The Kyoto Encyclopedia of Genes and Genomes (KEGG) database was used for the functional enrichment analysis.
Plant material, RNA-Seq, and qRT-PCR analysis of the 16 selected members
The ‘Emerald Pearl’ asparagus, a local variety cultivated in Jilin province, was obtained from the Economic Botany Institute of the Jilin Academy of Agricultural Sciences (Gongzhuling, Jilin, China) for gene expression experiments. Four-month-old seedlings with consistent growth rates were selected as plant materials. The plant materials were divided into different parts, including root, stem, leaf, and crown tissues, for tissue-specific expression analysis. The root tissue was also used as the target sample for analyzing gene expression under abiotic stress. The plants were subjected to five treatments; the control (water, CK), cold stress (4°C) (Zhang et al. Citation2022a), salt stress (S) (70 mmol/L NaCl) (Zhao et al. Citation2021b; Zhang et al., Citation2022b), heavy metal stress (H) (60 mg/L of HgCl2) (Zhang et al. Citation2022a), and drought stress (D) (15% PEG) (Mu et al. Citation2022). Sampling was conducted after 48 h of the treatments. The ABA content of samples was determined by HPLC-MS/MS (Shimadzu Lc-20AD; Shimadzu, Kyoto, Japan; AB Sciex ABAB5500; AB Sciex, Framingham, MA, USA) according to the method by Xiong et al. (Citation2014). The RNA of each sample was extracted using the MolPure® Plant RNA Kit (19291, YEASEN, Shanghai, China) and assessed by 1% agarose gel electrophoresis and a NanoDrop instrument (OneC, Thermo Fisher, Waltham, MA, USA). The validated RNA samples were sent to Majorbio (Shanghai, China) for RNA-Seq analysis. The reference genome was Asparagus officinalis with the version number GCF_001876935. Quantitative analysis was conducted to determine the expression levels of transcripts using the expression quantification software RSEM (Li and Dewey Citation2011). After obtaining the read counts of the transcripts, DESeq2 was used to analyze the differential expression of transcripts between samples. The RNA of each sample was also used to reverse transcribe cDNA using the Hifair® II 1st Strand cDNA Synthesis SuperMix kit for qPCR (11123, YEASEN). Primer Premier 5 was used to design the primers for AobZIP genes, and Aoactin-1 was set as the internal reference gene (Table S1) (Yi et al. Citation2019). The Light Cycler 480II system (Roche, Roche Diagnostics, Basel, Switzerland) was used to perform qRT-PCR with three biological and technical replicates. The 2-ΔΔCT method was used to calculate the relative expression levels (Livak and Schmittgen Citation2001).
Results
Identification of bZIP members in asparagus (Asparagus officinalis)
Using the A. officinalis from the NCBI database as the reference genome, 62 proteins were identified to have a bZIP domain by HMM profile analysis. After InterPro and SMART analyses and the removal of duplicate sequences, 46 proteins were determined to have a bZIP domain, making them members of the bZIP family in asparagus. The proteins were named AobZIP1–46 based on the order of their chromosomal location. All of these bZIP members were distributed across ten chromosomes, with NC_33794 having the most members (eight) and NC_33799 having only one member. AobZIP45 and AobZIP46 were located on NW_017972411 and NW_017972478. Detailed information on AobZIP genes is shown in Table S2. The putative protein lengths of AobZIP members ranged from 82 to 648, while the instability index of the AobZIP proteins was between 40.62 and 83.04 ().
Evolutionary analysis of AobZIP genes
The protein sequences of AobZIP members were used to analyze the evolutionary relationship based on a gene tree constructed by the maximum likelihood method with the LG + G model implemented in MEGA X. AobZIP members were divided into 14 subgroups based on the evolutionary analysis, in which subgroups VIII, X and XIII had only one AobZIP member each, while subgroup XII had the most AobZIP members (nine) ().
Gene structure and motif analysis
The GSDS was used to analyze the gene structure of AobZIP members, which largely corresponded to their evolutionary relationship (A). The bZIP domain was also used to validate the accuracy of each AobZIP member. All AobZIP proteins contained a conserved bZIP domain, and the AobZIP members within the same subgroup had similar structures (B). Additionally, the motifs of putative AobZIP proteins were analyzed using MEME, and the detailed results presented in Table S3 indicate that AobZIP members in the same subgroup had similar motifs. Motif 10 only occurred in subgroup III, while only subgroup IV had motifs 3 and 4. Subgroup VII contained motif 5, while motif 6 only occurred in subgroup XII (C).
Figure 3. The gene structure and motif analysis of AobZIP members. (A) The evolutionary relationship of AobZIP members. (B) The gene structure of AobZIP members. The blue, pink, and orange rectangles represent untranslated regions (UTRs), coding sequences (CDSs), and bZIP domains, respectively. (C) The motifs occurring in the AobZIP members, and each of the ten motifs is represented by a different color.
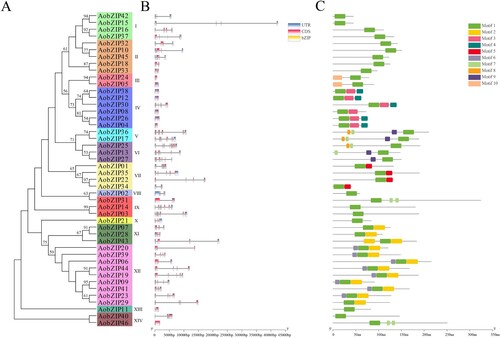
Evolutionary analysis of bZIP members in Asparagus officinalis and Arabidopsis
The identified bZIP members in asparagus and Arabidopsis were used to analyze the evolutionary relationship among gene family members. The LG + G model was the most suitable, as predicted by MEGA X. Although the order of subgroups had changed, the number of subgroups remained unchanged, and the bZIP members in these two species were divided into 14 subgroups, each containing members from both species. Similar to the gene structure and motif analysis, the gene structure and motifs of bZIP members were similar in each subgroup ().
Figure 4. The evolutionary, gene structure, and motifs analysis of bZIP members in asparagus (Asparagus officinalis) and Arabidopsis. The outer ring represents the gene structure of bZIP members, while the green, yellow, and pink rectangles represent untranslated regions (UTRs), coding sequences (CDSs), and bZIP domains, respectively; The middle ring shows the evolution of bZIP members while the red dots represent the AobZIP members. The inner ring shows the motifs of the bZIP members, and each of the ten motifs is in a different color. Different subgroups of AobZIP members (I–XIV) are shown with different background colors.
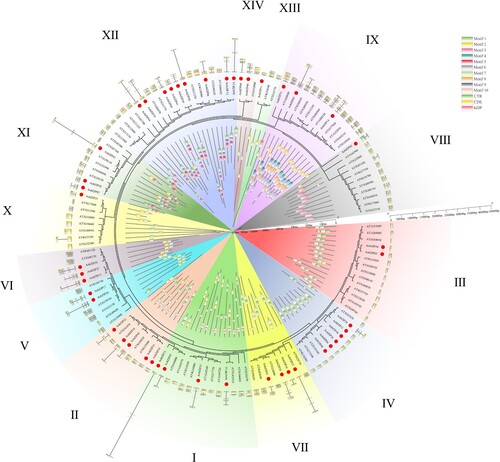
Cis-acting element analysis
PlantCARE was used to analyze the cis-acting elements of AobZIP members, and their functions, as predicted by PlantCARE (Table S4), largely corresponded to their evolutionary relationships (A). AobZIP members had cis-acting elements associated with hormones (green elements in ) and abiotic stress (red elements in ). The hormone-related elements, such as ARBE, AuxRR-core, TATC-box, and TGA-box elements, indicated that AobZIP proteins interact with hormones. Similarly, the stress-related elements, such as LTR, MBS, ARE, and MBSI, indicated that AobZIP proteins are involved in abiotic stress responses. Additionally, members in the same subgroup had similar cis-acting elements (B).
Collinearity analysis
TBtools was used to analyze the collinearity of AobZIP members. Only one pair of AobZIP members had perfect collinearity (AobZIP12 and AobZIP38), while four AobZIP members showed perfect collinearity with their homologs in Arabidopsis, which was higher than that observed between asparagus and soybean, rice or maize (two each) ().
Figure 6. The collinearity analysis of AobZIP genes. (A) A visualization of the collinearity analysis of AobZIP genes, in which the two inner circles represent the density of the genome, the red line represents the single intraspecific collinear relationship, and the gray background represents the collinear relationship in the genome. (B) A visualization of the collinearity analysis of AobZIP genes from asparagus and four other species (rice, maize, soybean, and Arabidopsis), in which the red rectangles represent asparagus chromosomes, while the yellow, green, and pink rectangles represent collinear soybean, rice, and maize chromosomes, respectively. The lines of different colors connecting the chromosomes indicate intraspecific collinearity relationships, while the gray background represents the collinear relationship in the genome.
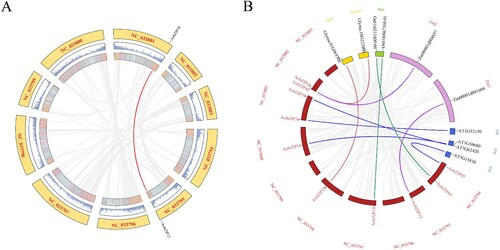
KEGG enrichment analysis of AobZIP genes
The KEGG enrichment analysis of AobZIP genes indicated that the genes were mostly enriched in the ‘plant hormone signal transduction’ pathway (map04075) (P = 7.93 × e−19). These results revealed that AobZIPs interact with plant hormones (A). In map04075, 16 AobZIP members (detailed information is shown in Table S5) were enriched in the ABA-responsive element binding factor (ABF) module in the ABA pathway (B), indicating that AobZIP proteins interact with ABA.
Figure 7. The Kyoto Encyclopedia of Genes and Genomes (KEEG) analysis of AobZIP genes. (A) A visualization of the KEGG enrichment analysis of AobZIP genes, in which the circle size represents the degree of enrichment of AobZIP members. The color variation from blue to red represents the P-value range from low to high. (B) The enriched carotenoid biosynthesis pathway, in which the AobZIP proteins are enriched the red module.
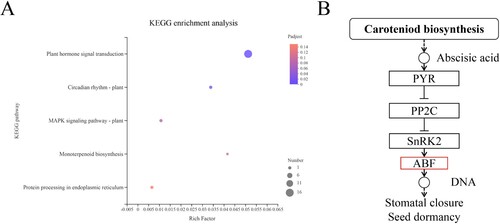
Tissue-specific expression of AobZIP genes in RNA-Seq data
The expression pattern of AobZIP genes at the seeding stage was assayed by RNA-Seq, and the raw data have been uploaded to the Zenodo database (10.5281/zenodo.7112175). The expression levels of 16 AobZIP genes with a higher expression are shown in . AobZIP genes had tissue-specific expression patterns in root, stem, leaf, and crown tissues, but different members had different patterns. For example, most AobZIP members, such as AobZIP01, AobZIP04, AobZIP11, AobZIP14, AobZIP25, AobZIP26, AobZIP43, and AobZIP45, had higher expression in root tissues, while some AobZIP members, e.g. AobZIP23, had lower expression in roots than in other tissues. The RNA-Seq results suggested that roots could be used as target tissues for studying AobZIP gene expression at the seeding stage.
Figure 8. The AobZIP expression patterns in different tissues at the seeding stage. The expression levels of AobZIP were analyzed in four different tissues (including root, stem, leaf, and crown) at the seeding stage. (A) Diagram of different asparagus tissues at the seeding stage. (B) The expression levels of AobZIP genes at the seeding stage. The color variation of each tissue, from blue to red, represents the variation of the relative expression level from low to high.
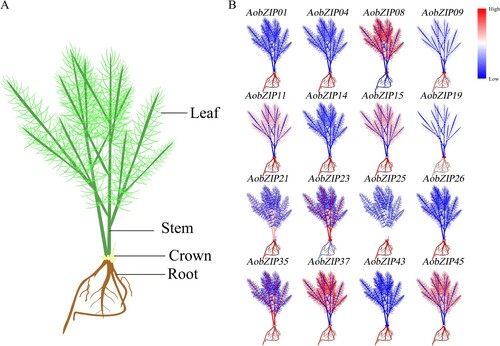
Abiotic stress-induced expression of AobZIP genes and ABA content
The expression of AobZIP genes under abiotic stress (including control, cold, drought, salt, and heavy metal treatments) was analyzed by qRT-PCR to evaluate the roles of the gene family members in abiotic stress responses, and the ABA content was determined by HPLC-MS/MS. The ABA content responded significantly (P < 0.05) to abiotic stress (A), and the expression level of AobZIP genes also changed significantly under abiotic stress (B): Some AobZIP genes (such as AobZIP01, AobZIP08, AobZIP11, AobZIP25, AobZIP37, and AobZIP45) were significantly up-regulated (P < 0.05), while some (including AobZIP09 and AobZIP14) were down-regulated under abiotic stress. This suggested that some AobZIP proteins are involved with ABA responses under abiotic stress at the seeding stage.
Figure 9. The qRT-PCR analysis of the gene expression levels of the 16 AobZIP members in the asparagus seedling roots under abiotic stress. The green bars represent the control (CK) conditions, while the blue, brown, purple, and red bars represent cold (C), drought (D), salt (S), and heavy metal (H) stress conditions, respectively. Different letter(s) above the bars indicate significant differences obtained via a least significant difference test following an ANOVA (α = 0.05). (A) ABA content. (B) AobZIP gene expression levels.
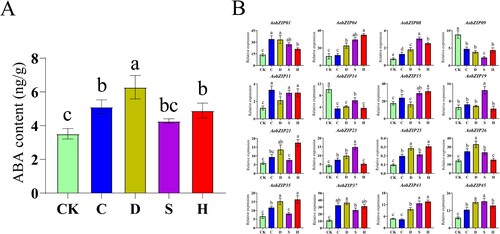
Discussion
The number of bZIP members has been found to vary among species. A previous study identified 78 bZIP members in Arabidopsis (Dröge-Laser et al. Citation2018), while 89 bZIP members were found in Oryza sativa (Nijhawan et al. Citation2008). Additionally, 96 members were found in Fagopyum talaricum (Fan et al. Citation2019), while 80 and 69 members were identified in Solanum tuberosum and Solanum lycopersicum, respectively (Li et al. Citation2015; Herath and Verchot Citation2020). The number of bZIP members also varies among legumes; for example, there are 160, 84, 75, and 72 bZIP members in Glycine max, Phaseolus vulgaris, Vigna radiata, and Vigna angularis, respectively (Zhang et al. Citation2018; Zhao et al. Citation2021b; Yin et al. Citation2022; Zhang et al. Citation2022b). In this study, 46 bZIP members were identified in the genome of Asparagus officinalis, fewer than in some species. This may be due to the smaller genome size of Asparagus officinalis or the less numerous genome replication events in its ancestral lineage.
In our evolutionary analyses, AobZIP members were divided into 14 subgroups in both the single species and multispecies trees ( and ). Similarly, the bZIP members in Vigna angularis, Triticum aestivum, and Ziziphus jujuba were divided into 14 subgroups in a previous evolutionary analysis (Li et al. Citation2015; Zhang et al. Citation2020b; Yin et al. Citation2022), suggesting that these subgroups are evolutionarily conserved. In our motif analysis, AobZIP genes in the same subgroup had similar motifs, suggesting that subgroup members have similar functions. Also, some of the identified motifs were found in bZIP members of other species, such as motif 1 (NRESA), which was found in VabZIP genes (Yin et al. Citation2022), and motif 2, which was also contained in poplar bZIP members (Zhao et al., Citation2021b). The cis-acting element analysis identified two categories of cis-acting elements (the hormone-related elements, such as ARBE, AuxRR-core, TATC-box, and TGA-box elements, and the stress-related elements, such as LTR, MBS, ARE, and MBSI elements) in AobZIP sequences. Both hormone-related and stress-related elements have been found in the bZIP members of other species (Dröge-Laser et al. Citation2018), indicating that AobZIP proteins are also involved in hormone and stress responses. Additionally, KEGG enrichment analysis showed that AobZIP genes were enriched in the ABA pathway (). As a pathway responding to abiotic stresses, ABA pathway was seemed as to mitigate drought and salinity stress enriched by bZIP members (Hussain et al. Citation2021), which the result was similar to the findings on PvbZIPs and VrbZIPs genes enriched in ABA pathway (Zhao et al. Citation2021b; Zhang et al. Citation2022b). Collinearity analysis demonstrated that AobZIP36 collineated with AT1G32150, which regulates stress tolerance in Arabidopsis (Li et al. Citation2019b). Similarly, AT3G26420 had collinearity with AobZIP38, which responds to abiotic stress (Kim et al. Citation2005; Kim and Kang Citation2006). The cis-acting element, collinearity, and KEGG enrichment analyses suggest that AobZIP proteins are involved in hormone and stress responses.
The expression pattern of AobZIP genes at the seeding stage exhibited tissue specificity (), similar to the results of PvbZIP, VabZIP, and RsbZIP genes (Fan et al. Citation2019; Zhao et al. Citation2021b; Yin et al. Citation2022), from which the root tissue was considered a target tissue for studying bZIP members. The changes in the expression of these genes under abiotic stress suggested that these members respond to abiotic stress, making them candidate bZIP members for further research (Zhang et al. Citation2021a). In the present study, the expression level of AobZIP genes and the ABA content of the different tissues changed significantly under abiotic stresses (including cold, drought, salt, and heavy metal). This indicated that AobZIP genes (such as AobZIP01, AobZIP04, AobZIP11, AobZIP14, AobZIP25, AobZIP26, AobZIP43, and AobZIP45) might function in abiotic stress and ABA responses, similar to the function in other plant species bZIP members (Ng et al. Citation2018), such as StbZIP25 (Wang et al. Citation2021), TabZIP96 (Liang et al. Citation2022) and CabZIP25 (Gai et al. Citation2020). These bZIP members could thus be used for further research, especially in breeding resistance to abiotic stress.
Conclusion
In the present study, 46 AobZIP members were identified based on the Asparagus officinalis reference genome and comprehensively analyzed (via evolutionary, motif, gene structure, cis-acting elements, collinearity, KEGG enrichment, and expression pattern analyses). AobZIP proteins were found to be likely involved in abiotic stress and hormone responses, especially ABA responses. Moreover, some AobZIP genes (such as AobZIP01, AobZIP04, AobZIP11, AobZIP14, AobZIP25, AobZIP26, AobZIP43, and AobZIP45) could be used as candidate genes for improving stress resistance of asparagus. These findings provide valuable insights into AobZIP genes and establish a foundation for future research and breeding of Asparagus officinalis.
Authors’ contributions
Yu-Huan Li, Cai-Hua Li, Yan Cheng and Luo-Ming Zhang: Writing original draft; Yu-Huan Li, Haiyu Xu and Cai-Hua Li: Data curation; Jun-Jie Xu, Ji-Xing Zhu, Lei Wang: Conceptualization and methodology; Chang-Jian Chen and Yi Zheng: Software and Formal data analysis and preparation of materials; Cai-Hua Li and Zhong-Sheng Mu: Methodology; Zhong-Sheng Mu and Wlodzimierz Zygmunt Krzesnski: Conceptualization, data curation and funding acquisition. All authors reviewed manuscript.
Ethics approval and consent to participate
Not applicable.
Consent for publication
Not applicable.
Availability of data and materials
All data generated or analyzed in this study are included and uploaded to Zenodo database in this manuscript.
Supplemental Material
Download MS Word (11.5 KB)Supplemental Material
Download MS Word (12.2 KB)Supplemental Material
Download MS Word (325.1 KB)Supplemental Material
Download MS Word (27.5 KB)Supplemental Material
Download MS Word (12.3 KB)Disclosure statement
No potential conflict of interest was reported by the author(s).
Additional information
Funding
Notes on contributors
Yu-Huan Li
Yu-Huan Li, Research Interests: Economic crop breeding. Economic Botany Institute, Jilin Academy of Agricultural Sciences.
Luo-Ming Zhang
Luo-Ming Zhang, Research Interests: Bioinformatics analysis. Economic Botany Institute, Jilin Academy of Agricultural Sciences. University of Melbourne, VIC 3010, Australia.
Jun-Jie Xu
Jun-Jie Xu, Research Interests: Economic crop breeding. Heilongjiang Bayi Agricultural Universitys.
Ji-Xing Zhu
Ji-Xing Zhu, Research Interests: Economic crop breeding. Heilongjiang Bayi Agricultural University.
Lei Wang
Lei Wang, Research Interests: Economic crop breeding. Heilongjiang Bayi Agricultural University.
Chang-Jian Chen
Chang-Jian Chen, Research Interests: Economic crop physiology. Economic Botany Institute, Jilin Academy of Agricultural Sciences.
Haiyu Xu
Haiyu Xu, Research Interests: Economic crop physiology. Economic Botany Institute, Jilin Academy of Agricultural Sciences.
Yi Zheng
Yi Zheng, Research Interests: Economic crop physiology. Economic Botany Institute, Jilin Academy of Agricultural Sciences.
Cai-Hua Li
Cai-Hua Li, Research Interests: Economic crop breeding. Economic Botany Institute, Jilin Academy of Agricultural Sciences.
Zhong-Sheng Mu
Zhong-Sheng Mu, Research Interests: Economic crop breeding. Economic Botany Institute, Jilin Academy of Agricultural Sciences.
Wlodzimierz Zygmunt Krzesnski
Wlodzimierz Zygmunt Krzesnski, Research Interests: Economic crop physiology. Department of Vegetable Crops,Poznan University of Life Science, Poland.
References
- Bailey TL, Boden M, Buske FA, Frith M, Grant CE, Clementi L, Ren J, Li WW, Noble WS. 2009. MEME SUITE: tools for motif discovery and searching. Nucleic Acids Res. 37:W202–W208. doi:10.1093/nar/gkp335.
- Baillo EH, Kimotho RN, Zhang Z, Xu P. 2019. Transcription factors associated with abiotic and biotic stress tolerance and their potential for crops improvement. Genes (Basel). 10(10):771. doi:10.3390/genes10100771. PMID: 31575043; PMCID: PMC6827364.
- Chen C, Chen H, Zhang Y, Thomas HR, Frank MH, He Y, Xia R. 2020. Tbtools: an integrative toolkit developed for interactive analyses of big biological data. Mol Plant. 13:1194–1202. doi:10.1016/j.molp.2020.06.009.
- Dietrich K, Weltmeier F, Ehlert A, Weiste C, Stahl M, Harter K, Dröge-Laser W. 2011. Heterodimers of the Arabidopsis transcription factors bZIP1 and bZIP53 reprogram amino acid metabolism during low energy stress. Plant Cell. 23:381–395. doi:10.1105/tpc.110.075390.
- Dröge-Laser W, Snoek BL, Snel B, Weiste C. 2018. The Arabidopsis bZIP transcription factor family-an update. Curr Opin Plant Biol. 45:36–49. doi:10.1016/j.pbi.2018.05.001.
- Ellenberger TE, Brandl CJ, Struhl K, Harrison SC. 1992. The GCN4 basic region leucine zipper binds DNA as a dimer of uninterrupted alpha helices: crystal structure of the protein-DNA complex. Cell. 71:1223–1237. doi:10.1016/S0092-8674(05)80070-4.
- Fan L, Xu L, Wang Y, Tang M, Liu L. 2019. Genome- and transcriptome-wide characterization of bZIP gene family identifies potential members involved in abiotic stress response and anthocyanin biosynthesis in radish (Raphanus sativus L.). Int J Mol Sci. 20. doi:10.3390/ijms20246334.
- Finn RD, Attwood TK, Babbitt PC, Bateman A, Bork P, Bridge AJ, Chang HY, Dosztányi Z, El-Gebali S, Fraser M, et al. 2017. InterPro in 2017-beyond protein family and domain annotations. Nucl Acids Res. 45:D190–D199. doi:10.1093/nar/gkw1107.
- Finn RD, Clements J, Arndt W, Miller BL, Wheeler TJ, Schreiber F, Bateman A, Eddy SR. 2015. HMMER web server: 2015 update. Nucl Acids Res. 43:W30–W38. doi:10.1093/nar/gkv397.
- Gai WX, Ma X, Qiao YM, Shi BH, Ul Haq S, Li QH, Wei AM, Liu KK, Gong ZH. 2020. Characterization of the bZIP transcription factor family in pepper (Capsicum annuum L.): CabZIP25 positively modulates the salt tolerance. Front Plant Sci. 11:139. doi:10.3389/fpls.2020.00139.
- Guan Y, Ren H, Xie H, Ma Z, Chen F. 2009. Identification and characterization of bZIP-type transcription factors involved in carrot (Daucus carota L.) somatic embryogenesis. Plant J. 60:207–217. doi:10.1111/j.1365-313X.2009.03948.x.
- Han H, Dong L, Zhang W, Liao Y, Wang L, Wang Q, Ye J, Xu F. 2023. Ginkgo biloba GbbZIP08 transcription factor is involved in the regulation of flavonoid biosynthesis. J Plant Physiol. 287:154054. doi:10.1016/j.jplph.2023.154054. Epub ahead of print. PMID: 37487356.
- Hartmann L, Pedrotti L, Weiste C, Fekete A, Schierstaedt J, Göttler J, Kempa S, Krischke M, Dietrich K, Mueller MJ, et al. 2015. Crosstalk between Two bZIP signaling pathways orchestrates salt-induced metabolic reprogramming in Arabidopsis roots. Plant Cell. 27:2244–2260. doi:10.1105/tpc.15.00163.
- He Q, Cai H, Bai M, Zhang M, Chen F, Huang Y, Priyadarshani S, Chai M, Liu L, Liu Y, et al. 2020. A soybean bZIP transcription factor GmbZIP19 confers multiple biotic and abiotic stress responses in plant. Int J Mol Sci. 21. doi:10.3390/ijms21134701.
- Herath V, Verchot J. 2020. Insight into the bZIP gene family in Solanum tuberosum: genome and transcriptome analysis to understand the roles of gene diversification in spatiotemporal gene expression and function. Int J Mol Sci. 22. doi:10.3390/ijms22010253.
- Hu B, Jin J, Guo AY, Zhang H, Luo J, Gao G. 2015. GSDS 2.0: an upgraded gene feature visualization server. Bioinformatics. 31:1296–1297. doi:10.1093/bioinformatics/btu817.
- Hu CD, Chinenov Y, Kerppola TK. 2002. Visualization of interactions among bZIP and Rel family proteins in living cells using bimolecular fluorescence complementation. Mol Cell. 9:789–798. doi:10.1016/S1097-2765(02)00496-3.
- Hussain Q, Asim M, Zhang R, Khan R, Farooq S, Wu J. 2021. Transcription factors interact with ABA through gene expression and signaling pathways to mitigate drought and salinity stress. Biomolecules. 11(8):1159. doi:10.3390/biom11081159. PMID: 34439825; PMCID: PMC8393639.
- Izawa T, Foster R, Chua NH. 1993. Plant bZIP protein DNA binding specificity. J Mol Biol. 230:1131–1144. doi:10.1006/jmbi.1993.1230.
- Jakoby M, Weisshaar B, Dröge-Laser W, Vicente-Carbajosa J, Tiedemann J, Kroj T, Parcy F. 2002. bZIP transcription factors in Arabidopsis. Trends Plant Sci. 7:106–111. doi:10.1016/S1360-1385(01)02223-3.
- Kim YO, Kang H. 2006. The role of a zinc finger-containing glycine-rich RNA-binding protein during the cold adaptation process in Arabidopsis thaliana. Plant Cell Physiol. 47:793–798. doi:10.1093/pcp/pcj047.
- Kim YO, Kim JS, Kang H. 2005. Cold-inducible zinc finger-containing glycine-rich RNA-binding protein contributes to the enhancement of freezing tolerance in Arabidopsis thaliana. Plant J. 42:890–900. doi:10.1111/j.1365-313X.2005.02420.x.
- Krzywinski M, Schein J, Birol I, Connors J, Gascoyne R, Horsman D, Jones SJ, Marra MA. 2009. Circos: an information aesthetic for comparative genomics. Genome Res. 19:1639–1645. doi:10.1101/gr.092759.109.
- Kuang Q, Sheng W. 2022. The entire chloroplast genome sequence of Asparagus setaceus (Kunth) Jessop: genome structure, gene composition, and phylogenetic analysis in Asparagaceae. Open Life Sci. 17(1):1541–1554. doi:10.1515/biol-2022-0497. PMID: 36474705; PMCID: PMC9684740.
- Kuhl JC, Havey MJ, Martin WJ, Cheung F, Yuan Q, Landherr L, Hu Y, Leebens-Mack J, Town CD, Sink KC. 2005. Comparative genomic analyses in Asparagus. Genome. 48(6):1052–1060. doi:10.1139/g05-073. PMID: 16391674.
- Kumar S, Stecher G, Li M, Knyaz C, Tamura K. 2018. Mega X: molecular evolutionary genetics analysis across computing platforms. Mol Biol Evol. 35:1547–1549. doi:10.1093/molbev/msy096.
- Lescot M, Déhais P, Thijs G, Marchal K, Moreau Y, Van De Peer Y, Rouzé P, Rombauts S. 2002. PlantCARE, a database of plant cis-acting regulatory elements and a portal to tools for in silico analysis of promoter sequences. Nucleic Acids Res. 30:325–327. doi:10.1093/nar/30.1.325.
- Letunic I, Doerks T, Bork P. 2015. SMART: recent updates, new developments and status in 2015. Nucleic Acids Res. 43:D257–D260. doi:10.1093/nar/gku949.
- Li B, Dewey CN. 2011. RSEM: accurate transcript quantification from RNA-Seq data with or without a reference genome. BMC Bioinformatics. 12:323. doi:10.1186/1471-2105-12-323. PMID: 21816040; PMCID: PMC3163565.
- Li D, Fu F, Zhang H, Song F. 2015. Genome-wide systematic characterization of the bZIP transcriptional factor family in tomato (Solanum lycopersicum L.). BMC Genomics. 16:771. doi:10.1186/s12864-015-1990-6.
- Li SF, Li JR, Wang J, Dong R, Jia KL, Zhu HW, Li N, Yuan JH, Deng CL, Gao WJ. 2019a. Cytogenetic and genomic organization analyses of chloroplast DNA invasions in the nuclear genome of Asparagus officinalis L. provides signatures of evolutionary complexity and informativity in sex chromosome evolution. BMC Plant Biol. 19(1):361. doi:10.1186/s12870-019-1975-8. PMID: 31419941; PMCID: PMC6698032.
- Li Y, Liu W, Zhong H, Zhang HL, Xia Y. 2019b. Redox-sensitive bZIP68 plays a role in balancing stress tolerance with growth in Arabidopsis. Plant J. 100:768–783. doi:10.1111/tpj.14476.
- Liang Y, Xia J, Jiang Y, Bao Y, Chen H, Wang D, Zhang D, Yu J, Cang J. 2022. Genome-wide identification and analysis of bZIP gene family and resistance of TaABI5 (TabZIP96) under freezing stress in wheat (Triticum aestivum). Int J Mol Sci. 23. doi:10.3390/ijms23042351.
- Liao Y, Zou HF, Wei W, Hao YJ, Tian AG, Huang J, Liu YF, Zhang JS, Chen SY. 2008. Soybean GmbZIP44, GmbZIP62 and GmbZIP78 genes function as negative regulator of ABA signaling and confer salt and freezing tolerance in transgenic Arabidopsis. Planta. 228:225–240. doi:10.1007/s00425-008-0731-3.
- Liu H, Tang X, Zhang N, Li S, Si H. 2023. Role of bZIP transcription factors in plant salt stress. Int J Mol Sci. 24(9):7893. doi:10.3390/ijms24097893. PMID: 37175598; PMCID: PMC10177800.
- Liu J, Chen N, Chen F, Cai B, Dal Santo S, Tornielli GB, Pezzotti M, Cheng ZM. 2014. Genome-wide analysis and expression profile of the bZIP transcription factor gene family in grapevine (Vitis vinifera). BMC Genomics. 15:281. doi:10.1186/1471-2164-15-281.
- Liu L, White MJ, Macrae TH. 1999. Transcription factors and their genes in higher plants functional domains, evolution and regulation. Eur J Biochem. 262:247–257. doi:10.1046/j.1432-1327.1999.00349.x.
- Livak KJ, Schmittgen TD. 2001. Analysis of relative gene expression data using real-time quantitative PCR and the 2(-Delta Delta C(T)). Methods. 25:402–408. doi:10.1006/meth.2001.1262.
- Ma H, Liu C, Li Z, Ran Q, Xie G, Wang B, Fang S, Chu J, Zhang J. 2018. Zmbzip4 contributes to stress resistance in maize by regulating ABA synthesis and root development. Plant Physiol. 178:753–770. doi:10.1104/pp.18.00436.
- Maier AT, Stehling-Sun S, Offenburger SL, Lohmann JU. 2011. The bZIP transcription factor PERIANTHIA: a multifunctional hub for meristem control. Front Plant Sci. 2:79. doi:10.3389/fpls.2011.00079.
- Mu Z, Wei Z, Liu J, Cheng Y, Song Y, Yao H, Yuan X, Wang S, Gu Y, Zhong J, et al. 2022. RNA-Seq analysis demonstrates different strategies employed by tiger nuts (Cyperus esculentus L.) in response to drought stress. Life (Basel). 12. doi:10.3390/life12071051.
- Ng DW, Abeysinghe JK, Kamali M. 2018. Regulating the regulators: the control of transcription factors in plant defense signaling. Int J Mol Sci. 19(12):3737. doi:10.3390/ijms19123737. PMID: 30477211; PMCID: PMC6321093.
- Nijhawan A, Jain M, Tyagi AK, Khurana JP. 2008. Genomic survey and gene expression analysis of the basic leucine zipper transcription factor family in rice. Plant Physiol. 146:333–350. doi:10.1104/pp.107.112821.
- Pan Y, Tsai CJ, Ma B, Nussinov R. 2010. Mechanisms of transcription factor selectivity. Trends Genet. 26:75–83. doi:10.1016/j.tig.2009.12.003.
- Pérez-Rodríguez P, Riaño-Pachón DM, Corrêa LG, Rensing SA, Kersten B, Mueller-Roeber B. 2010. PlnTFDB: updated content and new features of the plant transcription factor database. Nucleic Acids Res. 38:D822–D827. doi:10.1093/nar/gkp805.
- Plath S, Klocke E, Nothnagel T. 2022. Karyological and nuclear DNA content variation of the genus Asparagus. PLoS One. 17(3):e0265405. doi:10.1371/journal.pone.0265405. PMID: 35294505; PMCID: PMC8926174.
- Pruneda-Paz JL, Breton G, Nagel DH, Kang SE, Bonaldi K, Doherty CJ, Ravelo S, Galli M, Ecker JR, Kay SA. 2014. A genome-scale resource for the functional characterization of Arabidopsis transcription factors. Cell Rep. 8:622–632. doi:10.1016/j.celrep.2014.06.033.
- Rong S, Wu Z, Cheng Z, Zhang S, Liu H, Huang Q. 2020. Genome-wide identification, evolutionary patterns, and expression analysis of bZIP gene family in olive (Olea europaea L.). Genes (Basel). 11. doi:10.3390/genes11050510.
- Sagor GH, Berberich T, Tanaka S, Nishiyama M, Kanayama Y, Kojima S, Muramoto K, Kusano T. 2016. A novel strategy to produce sweeter tomato fruits with high sugar contents by fruit-specific expression of a single bZIP transcription factor gene. Plant Biotechnol J. 14:1116–1126. doi:10.1111/pbi.12480.
- Satoh R, Fujita Y, Nakashima K, Shinozaki K, Yamaguchi-Shinozaki K. 2004. A novel subgroup of bZIP proteins functions as transcriptional activators in hypoosmolarity-responsive expression of the ProDH gene in Arabidopsis. Plant Cell Physiol. 45:309–317. doi:10.1093/pcp/pch036.
- Schütze K, Harter K, Chaban C. 2008. Post-translational regulation of plant bZIP factors. Trends Plant Sci. 13:247–255. doi:10.1016/j.tplants.2008.03.002.
- Sheng W. 2020. The complete mitochondrial genome of Asparagus officinalis L. Mitochondrial DNA B Resour. 5(3):2627–2628. doi:10.1080/23802359.2020.1780986. PMID: 33457885; PMCID: PMC7782815.
- Sheng W. 2022. The entire chloroplast genome sequence of Asparagus cochinchinensis and genetic comparison to Asparagus species. Open Life Sci. 17(1):893–906. doi:10.1515/biol-2022-0098. PMID: 36045717; PMCID: PMC9372710.
- Sheng W, Deng J, Wang C, Kuang Q. 2023. The garden Asparagus (Asparagus officinalis L.) mitochondrial genome revealed rich sequence variation throughout whole sequencing data. Front Plant Sci. 14:1140043. doi:10.3389/fpls.2023.1140043. PMID: 37051082; PMCID: PMC10084930.
- Thalor SK, Berberich T, Lee SS, Yang SH, Zhu X, Imai R, Takahashi Y, Kusano T. 2012. Deregulation of sucrose-controlled translation of a bZIP-type transcription factor results in sucrose accumulation in leaves. PLoS One. 7:e33111. doi:10.1371/journal.pone.0033111.
- Toh S, Mccourt P, Tsuchiya Y. 2012. HY5 is involved in strigolactone-dependent seed germination in Arabidopsis. Plant Signal Behav. 7:556–558. doi:10.4161/psb.19839.
- Tsugama D, Fujino K. 2019. Data of whole genome sequencing of five garden asparagus (Asparagus officinalis) individuals with the MinION nanopore sequencer. Data Brief. 28:104838. doi:10.1016/j.dib.2019.104838. PMID: 31832531; PMCID: PMC6889792.
- Turner D, Kim R, Guo JT. 2012. TFinDit: transcription factor-DNA interaction data depository. BMC Bioinformatics. 13:220. doi:10.1186/1471-2105-13-220.
- Wang H, Peng J, Li Y, Xu L, Dai W, Zhao S. 2023. The role of walnut bZIP genes in explant browning. BMC Genomics. 24(1):377. doi:10.1186/s12864-023-09492-1. PMID: 37407925; PMCID: PMC10324250.
- Wang Q, Guo C, Li Z, Sun J, Wang D, Xu L, Li X, Guo Y. 2021. Identification and analysis of bZIP family genes in potato and their potential roles in stress responses. Front Plant Sci. 12:637343. doi:10.3389/fpls.2021.637343.
- Wang Y, Li J, Paterson AH. 2013. MCScanX-transposed: detecting transposed gene duplications based on multiple colinearity scans. Bioinformatics. 29:1458–1460. doi:10.1093/bioinformatics/btt150.
- Wei K, Chen J, Wang Y, Chen Y, Chen S, Lin Y, Pan S, Zhong X, Xie D. 2012. Genome-wide analysis of bZIP-encoding genes in maize. DNA Res. 19:463–476. doi:10.1093/dnares/dss026.
- Weiste C, Pedrotti L, Selvanayagam J, Muralidhara P, Fröschel C, Novák O, Ljung K, Hanson J, Dröge-Laser W. 2017. The Arabidopsis bZIP11 transcription factor links low-energy signalling to auxin-mediated control of primary root growth. PLoS Genet. 13:e1006607. doi:10.1371/journal.pgen.1006607.
- Xiong DM, Liu Z, Chen H, Xue JT, Yang Y, Chen C, Ye LM. 2014. Profiling the dynamics of abscisic acid and ABA-glucose ester after using the glucosyltransferase UGT71C5 to mediate abscisic acid homeostasis in Arabidopsis thaliana by HPLC-ESI-MS/MS. J Pharm Anal. 4:190–196. doi:10.1016/j.jpha.2014.01.004.
- Xu Z, Wang J, Ma Y, Wang F, Wang J, Zhang Y, Hu X. 2023. The bZIP transcription factor SlAREB1 regulates anthocyanin biosynthesis in response to low temperature in tomato. Plant J. 115(1):205–219. doi:10.1111/tpj.16224. Epub 2023 Apr 13. PMID: 36999610.
- Yang Y, Yu TF, Ma J, Chen J, Zhou YB, Chen M, Ma YZ, Wei WL, Xu ZS. 2020. The soybean bZIP transcription factor gene GmbZIP2 confers drought and salt resistances in transgenic plants. Int J Mol Sci. 21. doi:10.3390/ijms21020670.
- Yi TG, Yeoung YR, Choi IY, Park NI. 2019. Transcriptome analysis of Asparagus officinalis reveals genes involved in the biosynthesis of rutin and protodioscin. PLoS One. 14:e0219973. doi:10.1371/journal.pone.0219973.
- Yin Z, Meng X, Guo Y, Wei S, Lai Y, Wang Q. 2022. The bZIP transcription factor family in adzuki bean (Vigna Angularis): genome-wide identification, evolution, and expression under abiotic stress during the Bud stage. Front Genet. 13:847612. doi:10.3389/fgene.2022.847612.
- Zhang C, Li C, Liu J, Lv Y, Yu C, Li H, Zhao T, Liu B. 2017. The OsABF1 transcription factor improves drought tolerance by activating the transcription of COR413-TM1 in rice. J Exp Bot. 68:4695–4707. doi:10.1093/jxb/erx260.
- Zhang M, Liu Y, Cai H, Guo M, Chai M, She Z, Ye L, Cheng Y, Wang B, Qin Y. 2020a. The bZIP transcription factor GmbZIP15 negatively regulates salt- and drought-stress responses in soybean. Int J Mol Sci. 21. doi:10.3390/ijms21207778.
- Zhang M, Liu Y, Shi H, Guo M, Chai M, He Q, Yan M, Cao D, Zhao L, Cai H, Qin Y. 2018. Evolutionary and expression analyses of soybean basic Leucine zipper transcription factor family. BMC Genomics. 19:159. doi:10.1186/s12864-018-4511-6.
- Zhang Q, Geng J, Du Y, Zhao Q, Zhang W, Fang Q, Yin Z, Li J, Yuan X, Fan Y, et al. 2022a. Heat shock transcription factor (Hsf) gene family in common bean (Phaseolus vulgaris): genome-wide identification, phylogeny, evolutionary expansion and expression analyses at the sprout stage under abiotic stress. BMC Plant Biol. 22:33. doi:10.1186/s12870-021-03417-4.
- Zhang Q, Li M, Xia CY, Zhang WJ, Yin ZG, Zhang YL, Fang QX, Liu YC, Zhang MY, Zhang WHJB, Equipment B. 2021a. Transcriptome-based analysis of salt-related genes during the sprout stage of common bean (Phaseolus vulgaris) under salt stress conditions. Biotechnol Biotechnol Equip. 35:1086–1098. doi:10.1080/13102818.2021.1954091.
- Zhang Q, Zhang W-J, Yin Z-G, Li W-J, Xia C-Y, Sun H-Y, Yang Y-M, Wu H-B, Zhang S, Zhao H-HJ. 2022b. Genome-wide identification reveals the potential functions of the bZIP gene family in common bean (Phaseolus vulgaris) in response to salt stress during the sprouting stage. J Plant Growth Regul. 1–16.
- Zhang Y, Gao W, Li H, Wang Y, Li D, Xue C, Liu Z, Liu M, Zhao J. 2020b. Genome-wide analysis of the bZIP gene family in Chinese jujube (Ziziphus jujuba Mill.). BMC Genomics. 21:483. doi:10.1186/s12864-020-06890-7.
- Zhao K, Chen S, Yao W, Cheng Z, Zhou B, Jiang T. 2021b, Mar 1. Genome-wide analysis and expression profile of the bZIP gene family in poplar. BMC Plant Biol. 21(1):122. doi:10.1186/s12870-021-02879-w. PMID: 33648455; PMCID: PMC7919096.