ABSTRACT
Potato is an extremely important food crop due to its high nutritional and economic value; however, this crop suffers losses due to water-deficient conditions. The aim of the study was to analyze the expression profiles of the StDREB30 gene and to study the enzymatic antioxidant system of transgenic potatoes under water-deficient situations. The results depicted that following drought and 20% polyethylene glycol 6000 stresses, transgenic plants showed vigorous growth, increased antioxidant activities, low malondialdehyde, and enhanced differential expression of StDREB30 in transgenics compared to control plants. Results suggest that StDREB30 overexpression mitigated the negative effects of water-deficit treatments by reducing oxidative damage in plants. Hence, the StDREB30 gene retains extensive potential for crop bioengineering to generate highly tolerant crop plants.
KEY POLICY HIGHLIGHTS
Transgenic potatoes overexpressing StDREB30 showed enhanced tolerance to water-deficient conditions.
StDREB30 expression was almost 30, 5, and 3 folds higher in transgenic roots, shoots, and leaves respectively compared to non-transgenic plants after drought stress.
StDREB30 expression was 10, 4, and 3 folds higher in transgenic roots, shoots, and leaves, respectively after 20% PEG stress.
Transgenic plants showed vigorous growth, high antioxidant activities, and low lipid peroxidation activities in drought conditions.
The StDREB30 gene is a promising candidate for developing elite cultivars of crop plants.
1. Introduction
Potato (Solanum tuberosum L.) is one of the most vital nutritious noncereal crops globally (Pino et al. Citation2007) and its consumption is limited to not only feed and food but also fuels and production of commercial materials (Ren et al. Citation2009). Potato is grown in over 100 countries feeding over a billion people globally. It is a rich source of carbohydrates, proteins, dietary fibers, and antioxidants. Potato has gained importance due to its extraordinarily high nutritional content with high yielding potential. More than half of harvested potatoes come from developing countries where potatoes are a source of nutrition , employment, and income. A total of 376 million tons of potatoes were produced globally and have emerged as the third largest food crop due to their high yield providing global food security (FAOSTAT Citation2022).
Drought stress has been considered one of the most crucial abiotic stresses that limit plant development and growth. Extreme drought can disrupt the osmotic balance of cells, causing the closing of stomata, a decrease in photosynthetic activity, stunted growth, low nutrient access, and eventually death of plants, subsequently causing damage to crop productivity (Tang et al. Citation2019). Over the years drought stress has negatively impacted the yield and growth of potatoes resulting in large losses of yield and tuber quality as the growth and tuber formation in potatoes are heavily dependent on rainfall (Dahal et al. Citation2019). Furthermore, drought stress causes the downregulation of the expression of chlorophyll a-b binding proteins, sucrose biosynthetic enzymes, and key regulatory photosynthetic enzymes and enhances the expression of sucrose-breaking enzymes (Hwang et al. Citation2011). Thus, the adverse effects of drought on potatoes make them unsuitable for consumption and industrial processing. Plants show response and adapt to various environmental stresses at cellular, molecular, and physiological levels through defense mechanisms, such as signal perception, transcription regulation, gene editing, and signal transduction (Majeed et al. Citation2022). At the molecular level, several genes and transcription factors get activated in response to stress to provide tolerance to plants (Kizis et al. Citation2001). Activation and transcription of stress-responsive genes is an extremely important step in establishing abiotic stress tolerance mechanism in plants. Stress-responsive genes are actively involved in plant responses against biotic and abiotic stresses (Baoxiang et al. Citation2023). These drought-induced stress-responsive genes encode proteins that include enzymes and osmoprotectant proteins that actively provide stress tolerance. Thus, the key factor to reduce agriculture losses and to meet the growing food demands of the increasing global population is to uncover the mechanisms of potato tolerance to abiotic stresses (Shinozaki and Yamaguchi-Shinozaki Citation2006).
Drought-responsive genes encode products that are critical in providing initial stress response as well as in developing cellular stress response. Over the last two decades, significant work has been done to identify and characterize stress-responsive genes and their encoded proteins that protect plants against biotic and abiotic stresses. Stress-responsive genes are regulated by transcription factors that enhance the ability of plants to tolerate abiotic stresses by controlling the expression of downstream genes. Among these transcription factors, Dehydration Responsive Element Binding (DREB), is the most widely studied and highly recognized transcription factor family of APETALA2 (AP2)/ethylene-responsive factor (ERF) superfamily that regulate several abiotic stress-responsive genes (Sazegari and Niazi Citation2012).
DREB genes have been widely considered as one of the key regulators of various plant abiotic and biotic stress-responsive genes and have been overexpressed in a variety of plants to obtain genetically engineered crops that are tolerant to multiple abiotic stresses (Onele et al. Citation2023). Further studies have explored the role of DREB genes in development, hormone signaling, and light regulation (Mushtaq et al. Citation2021). Our previous works on in silico analysis of DREB gene promoters in potatoes have identified 104 cis-regulatory elements which were involved in various biotic and abiotic stress responses (Ain-Ali et al. Citation2021). DREB proteins contain a highly conserved DNA binding domain called the AP2 domain, which regulates the expression of target genes related to drought, salt, or other abiotic stress by binding to the Dehydration Responsive Element (DRE)/C-repeat (CRT) elements present in the promoter regions of target genes (Srivastava and Kumar Citation2019). DREB family is divided into 6 subgroups 1–6 based on their similarity to AP2:A (1–6) and AP2:B domains (Akhtar et al. Citation2012). Among the DREB subgroups, the functions of A-1 and A-2 subgroups genes are the most extensively studied, genes of both these subgroups are involved in providing tolerance to plants against drought, salinity, cold, and high temperature while DREBs belonging to A3-A4 subgroups induce abiotic stress tolerance in plants as well as involved in impairing cell division and growth regulation (Li et al. Citation2018). Extensive studies on A5 and A6 subgroups have not been reported; however, the limited findings show that they are also stress-inducible proteins and improve plants’ tolerance against drought and salt stress (Sun et al. Citation2015). Comprehensive transcriptome analysis of DREB gene families A1–A6 in tomatoes showed 29 DREB gene functions against drought and heat stress establishing the DREB gene family as multi-stress-tolerant gene family (Maqsood et al. Citation2022).
DREB transcription factors enhance the tolerance ability of plants to abiotic stress in different ways. For instance, SlDREB4 increases the ability of transgenic tomatoes to withstand heat stress by altering the content of osmoprotectant molecules, stress hormones, upregulating the antioxidant activities of enzymes at the physiological level, and regulating the expression of many downstream heat shock proteins at the molecular level (Mao et al. Citation2020). Further studies showed that GmDREB1 forms a heterodimer with GmERF008 or GmERF106 transcription factors to regulate the expression of downstream stress-responsive genes and enhance the drought tolerance of transgenic soyabean (Chen et al. Citation2023). DREB binds to DRE/C-repeat responsive cis-elements (CRT) present in the promoter region and initiates the stress response process. The DRE elements contain a core sequence of 6-bp, A/GCCGAC, which was discovered in responsive to desiccation 29A (RD29A) promoters. The expression level of RD29A increases under drought, salinity, and low-temperature stress (Lata et al. Citation2011).
DREBs of A-6 members have been identified in various plant species and their functions against abiotic stresses mainly heat, drought, salt, and cold have been characterized. For instance, CmDREB6 overexpression improves chrysanthemum tolerance to multiple abiotic stresses (Du et al. Citation2018). Overexpression of SsDREB enhances tolerance in transgenic tobacco to drought and salt stress (Zhang et al. Citation2015). Tese findings suggest that genes of the A-6 group of DREBs are good candidates in plant breeding against stress tolerance. However, limited data are available on the detailed characterization of A-6 DREB genes in transgenic crop plants against abiotic stresses.
In the current study, the StDREB30 gene was identified and overexpressed in potato var. Kuroda to develop transgenic potato plants (OE), Furthermore, OE and non-transgenic (NT) plants were exposed to osmotic stress in vitro and drought stress in soil, to investigate the potential role of StDREB30 in protecting potato plants against drought stress. Physiological attributes, gene expression analysis, and biochemical responses of OE and NTpotato plants were studied to evaluate transgenic performance in response to drought stress. In drought stress, transgenic potato plants demonstrated improved growth, increased leaf production, and resistance to wilting in addition to an activated antioxidant system. Thus, current research is a comprehensive study on the role of the StDREB30 gene in providing tolerance to potato plants against drought and osmotic stress.
2. Materials and methods
2.1. Plant material and growth conditions
In vitro, Solanum tuberosum var. Kuroda plants were propagated in culture tubes using single nodal segments on Murashige and Skoog (MS) basal medium containing 3% (w/v) sucrose and 0.8% (w/v) agar and 1.5 mg/L gibberellic acid (GA3) (Munir et al. Citation2011). Regular subculturing of in vitro plants was performed on fresh MS media after every 3 weeks to maintain healthy potato lines. Potato cultures were maintained under 16-hr light/8-hr dark cycle, 25 ± 2 °C temp, and light intensity of 40 µmol m−2 s–1 in growth chambers.
2.2. Identification of StDREB30
The StDREB30 gene sequence was retrieved from previous studies by Mushtaq et al. (Citation2021). Primers were designed from the StDREB30 mRNA sequence manually and restriction sites were selected and added accordingly to the binary vector pCAMBIA 1301 driven by Cauliflower Mosaic Virus (CaMV) 35S promoter. Nco1 and BglII restriction sites were added in forward and reverse primer, respectively forming overexpression fragments primers. The sequences of primers are as follows: StDREB30- F: 5′- GCCCATGGATTCCCAAATCTTTTCAAC -3′ and StDREB30 -R: 5′- GCAGATCTTATAGAGAGGCCCAATCAATTTC -3′, the underline sequence show the restriction enzyme site.
2.3. Cloning of the StDREB30 gene in binary vector pCAMBIA 1301
The coding sequences of the StDREB30 gene flanking Nco1 and BglII sites at 5’ and 3’ end, respectively were amplified and purified. The purified amplicon was ligated in TA cloning vector PCR 2.1 to make a fusion construct following the Invitrogen TA cloning kit protocol. The ligated mixture was used to transform electrocompetent E.coli DH5 α cells through electroporation. The positive transformants were selected on an antibiotic selection plate. E.coli DH5α transformation was followed by the plasmid isolation through the Invitrogen Purelink Miniprep kit. The positive transformation of DH5 α cells with the StDREB30 gene was further confirmed by performing colony PCR with gene-specific primers.
The fusion construct was inserted in the Nco1 and BglII sites of the plant expression vector. pCAMBIA 1301 to form pCAMBIA 1301::DREB30 construct. The resulting pCAMBIA 1301::DREB30 construct was introduced into Agrobacterium tumeficians strain LBA4404 through electroporation. The presence of the desired gene in the antibiotic-resistant bacterial colonies was confirmed by PCR using StDREB30-specific primers.
3. DNA/Protein sequences and phylogenetic analysis
Protein sequences of DREBs of A1–A6 subgroups were retrieved from NCBI (National Centre for Biotechnology Information) database. Multiple sequence alignment of amino acid sequences of DREBs from different plant species was performed using Clustal-W in MEGA X. A phylogenetic tree was constructed by the neighbor joining method with 1000 bootstrap replicates using the MEGA X software. The MEME software was used for motif prediction of StDREB30 and other classic DREB proteins.
3.1. Generation of StDREB30 transgenic potato plants
3-week-old healthy potato plants were used for agrobacterium-mediated transformation. Two separate protocols were optimized for leaf disk and internode transformation (). Glycerol stocks of binary vector construct were streaked on agar plates containing two selection antibiotics i.e. rifampicin (25 mg/L) and kanamycin (50 mg/L), and kept in an incubator at 28 °C for 48 hr. Random bacterial colonies were picked, inoculated in bacterial broth using the same antibiotics, and left overnight in a shaking incubator at 28 °C. 400 µl of the bacterial suspension was transferred to potato infection medium (PIM) and adjusted to give an O.D650 of 0.3. For leaf disk transformation, leaves were cut in the disk shape and were submerged in a bacterial suspension containing the StDREB30:: pCAMBIA 1301 construct for 30 min with gentle agitation in a shaker. The excised leaves were then blotted briefly on sterile filter paper and kept in the dark on Petri plates containing cultivation media (PCM) for 48 hr. Untransformed explants inoculated on MS media plates containing hygromycin were used as negative controls. After co-cultivation, the explants were washed with liquid MS medium containing cefotaxime (250 mg/L) twice and sterile deionized water 3–4 times to remove excess Agrobacterium. After washing with cefotaxime to remove excess agrobacterium the explants were transferred and kept on callus-inducing media (CIM1) for 7 days. After a short callus phase, explants were transferred to a shooting medium (SIM1) and were regularly subculture every 8–10 days for weeks. Regenerated shoots with 3–4 cm length and 2–3 leaves were excised and subcultured on the rooting medium (RIM1). Small shoots were inoculated on a rooting medium for 2–3 weeks until they developed into mature plants with thick shoots, expanded leaves, and proper roots. Plant transformations were carried out through internodes as explants were highly efficient. Several stable transgenic lines were produced using internodes as explants for transformation. Only the first 4 internodes 2–3 cm from the top of the potato plant were excised and cut longitudinally. The excised internodes were infected with liquid MS media containing overnight grown bacterial suspension with 1:20 volume for 30 min and placed on co-cultivation media (PCM2) in the dark for 48 hr.
Table 1. Culturing medium composition at different potato transformation stages. PCM (Potato co-culture media), CIM (Callus induction medium), SIM (Shoot induction medium), and RIM (Root induction medium).
After 48 hrs incubation period internodes were washed 2–3 times with 250 mg/L cefotaxime and 3–4 times with deionized water. Internodes from the callus induction medium were transferred to a shooting medium and were regularly subcultured for weeks (SIM2). The shoots were excised carefully from the mother explants and shifted to the rooting medium (RIM2) in test tubes. Excised shoots developed mature leaves and roots in around 3–4 weeks. Only healthy plantlets with proper roots were transferred to pots for further biochemical and molecular analysis.
3.2. Molecular analysis of transformants
Transgenic potato plants were confirmed via genomic PCR using hygromycin primers. DNA extracted from 100 to –200 mg leaves of 4-week-old OE plants and wild-type plants using the CTAB method was used as a template for the amplification of hygromycin gene. Forward (Hygromycin-F:GCTCCATACAAGCCAACCAC) and reverse (Hygromycin-R: CGAAAAGTTCGACAGCGTCTC) primers were designed from the hygromycin sequences present in the backbone of pCAMBIA 1301 vector.
3.3. β-glucuronidase (GUS) histochemical analysis
GUS histochemical analysis was performed according to the protocol described by Jefferson (Citation1989). OE and NT explants were used in performing the assay Solanum tuberosum var. Kuroda plants that were not carrying pCAMBIA1301:: DREB30 construct were taken as NT plants and were used as controls in the GUS assay . GUS analysis was performed by preparing a fixation solution of GUS buffer (50 mM sodium dihydrogen phosphate, 0.01% triton X-100 and 10 mM EDTA, final pH 7.0), and 2 Mm X-Gluc. Plant samples were incubated in GUS solution at 37 °C overnight in dark. After incubation, explants were washed with methanol to remove the chlorophyll pigmentation and observations were recorded.
3.4. Drought and osmotic stress treatments
After the confirmation of transgenics, plants were further propagated from nodal segments on MS media and the expression level of the StDREB30 gene in different parts of the potato i.e. leaves, stem, and roots under drought stress and normal conditions was observed. To perform drought stress assays in soil in vitro OE, plants were shifted to pots containing peat moss in 23 °C, 16 hr light/8 hr dark photoperiod cycle, 50–60% humidity in greenhouse chambers. OE and NT plants with the same health and height were selected for drought assay. Drought stress was imposed by withholding water for 14 days followed by rewatering for another 3 days. All the experiments were performed in 3 biological replicates.
To impose osmotic stress potato plants were grown in MS liquid media supplemented with 3% sucrose without kanamycin under 16 hr light/8 hr dark cycle, 25 ± 2 °C temperature and white, fluorescent light. After 3 weeks, MS liquid media was poured out and replaced with 50 ml fresh MS media supplemented with 20% PEG (polyethylene glycol) 6000. NT plants used as controls were also given the same treatment. 5 time periods (0, 3, 6, 12, and 24 hr) were selected for sampling of the plant samples under PEG stress. Roots, stems, and leaves were collected after each time point and immediately frozen in liquid nitrogen for a short time and ultimately stored at −80 °C for RNA extraction.
3.5. Tissue-specific expression profiling of the StDRE30 gene in potato plants
To analyze the changes in OE plants against drought stress at the gene level, qRT-PCR analysis was performed for the determination of relative expression of the StDREB30 gene under drought conditions. After the acclimatization of plants in soil drought stress was given to plants over 14 days followed by rewatering for 3 days. 2-week-old in vitro potato plants from growth chambers were first shifted to soil in thermophore cups for acclimatization. Plants hardened and gradually acclimatized in glass house conditions were subsequently shifted to pots for drought stress. Samples were collected on the first day of drought stress i.e. Day 0, Day 14, and after 3 days of rewatering the Rehydration point. Total RNA was extracted from the above-mentioned preserved samples of transgenic and non-transgenic plants collected at specified time points through the Trizol Reagent technique. The integrity of RNA was checked by Nanodrop TM 2000/2000c Spectrophotometer. Quantitative real-time PCR analysis was performed with a Thermoscientific Syber green amplification kit. The 7500 Fast Real-time PCR System (Applied Biosystem, USA) was used for real-time PCR analysis. 1 µg of total RNA was converted to synthesize cDNA through a Thermoscientific Revert Aid First Strand cDNA synthesis kit. Potato β-actin was used as an internal control gene to normalize the data. The relative expression level of the StDREB30 gene (Primers listed in Supplementary Table S1) was evaluated using the Livak method 2(−ΔΔCt). All the reactions were carried out with 3 biological replicates.
3.6. Determination of antioxidant activities
0.5 g plant tissue samples of OE and NT plants were homogenized in 0.5 mM EDTA and 0.1 M phosphate buffer (pH 7.5). The plant extract was centrifuged at 13,000 g at 4 °C for 12 min. The supernatant was collected and used for antioxidant enzyme analysis. Superoxide dismutase (SOD) activity was determined according to Zhang (Citation1992). Catalase activity was measured by a procedure described by Cakmak and Marschner (Citation1992) and absorbance was recorded at 240 nm. Ascorbate peroxidase (APX) activity was determined as reported by Yoshimura et al. (Citation2000). Lipid peroxidation in tissue samples was calculated in terms of malondialdehyde (MDA) content according to the protocol as described by Mellacheruvu et al. (Citation2016).
3.7. In silico transcriptome analysis
The in-silico transcriptome analysis of StDREB30 was performed through the Potato eFP browser (https://bar.utoronto.ca/efp_potato/cgi-bin/efpWeb.cgi). A simple heat map was generated by the eFP browser to analyze the potential role of StDREB30 against certain stressesStatistical Analysis.
All treatments were performed with 3 biological replicates and each experiment was conducted 3 times. The data were analyzed using GraphPad Prism 8.0 using two-way ANOVA (analysis of variance) at p ≤ 0.05.
4. Results
4.1. Multiple sequence alignment and phylogenetic analysis of StDREB30
StDREB30 shared the highest sequence similarity with LeDREB3 in tomatoes with full-length predicted protein. Phylogenetic analysis showed that StDREB30 clustered with the A-6 group of DREB proteins. Multiple sequence alignment showed that StDREB30 shared 3 β-sheets and α -helix with conserved AP2 domain. The AP2 domain analysis showed that out of 59 amino acids 18 amino acids were conserved in 21 DREB sequences. Valine at position 14th was conserved in all amino acid sequences while Glutamic acid (E) at position 19th was replaced by Leucine (L) in A-6 group members including StDREB30 and Isoleucine (I) and Alanine (A) in some other DREBs family members. Motif analysis of StDREB30 showed 3 β-sheets and 1 α-sheets in the AP2 domain ().
Figure 1. Sequence alignment, phylogenetic analysis, and motif prediction of StDREB30 (A) Multiple sequence alignment of StDREB30 with other plant species ScDREB5 (AMT92113.1), AtDREB1A (BAA33791.1), AtDREB2A (BAA33794.1), AtB14 (ABE65896.1),AtRAP2.1 (OAP00017.1), At1g7760 (ABD57508.1), OsDREB2A (AFB77198.1), OsAB14 (C7J2Z1.1), Os02g43940.1 (XP_015626317.1), ZmDBF1 (AAM80486.1), MsDREB5(AFM84627.1), GhDBP1 (AAO43165.1), GmDREB2 (ABB36645.1), StDREB30 (PGSC0003DMG400009475) LeDREB3 (AAO13360.1), GmDREB3 (AAZ03388.1), DRFL2C (ABC74511.1), AtTINY (NP_197953.1) and ZmDBF2 (AAM80485.1). Identical amino acid residues in all aligned sequences are highlighted by an asterisk (B) A phylogenetic tree was constructed using the neighbor-joining method with Poisson correction (pairwise deletion) and 1000 bootstrap value of replicates used to assess the robustness of the tree (C) Motif prediction of StDREB30 with other DREB proteins.
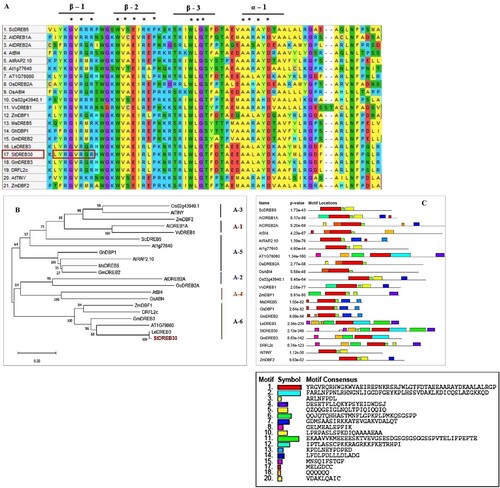
4.2. Cloning and transformation of the DREB gene in potato
The StDREB30 gene cloned in plant expression vector pCAMBIA 1301 was further transformed into Agrobacterium strain LBA 4404. The pCAMBIA 1301 vector was restricted through Nco1 and BglII enzymes as these sites were chosen as cloning sites for the StDREB30 gene. The newly designed binary construct is shown in ((A)). Colony PCR confirmed the presence of the 795 bp StDREB30 gene in pCAMBIA 1301 ((B)) (Supplementary Figure S1). The resulting transformed plate was further used for potato plant transformation.
Figure 2. Schematic representation and Colony PCR confirmation of the StDREB30 gene (A) Schematic representation of pCAMBIA 1301::StDREB30 used for Agrobacterium-mediated potato transformation. LB: Left T-DNA border, RB: Right T-DNA border, HPT II: Hygromycin transferase gene, CaMV: Cauliflower mosaic 35 S promoter. (B) 795 bp StDREB30 gene was confirmed in binary vector pCAMBIA 1301 through colony PCR. Lane M contains a 1 kb ladder, Lane 1 contains negative control and Lane 2–7 contains positive clones containing the 795 bp StDREB30 gene.
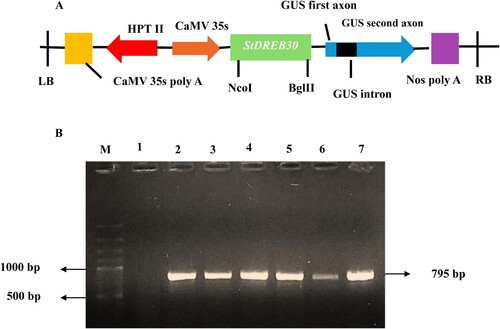
4.3. Confirmation of transgenic potato plants
Plants were regularly subcultured from internodal and nodal sections to maintain healthy young plants. Leaf disks and internodes-mediated potato transformation led to the successful generation of transformed potato plants (). The OE potato plants, containing pCAMBIA 1301-StDREB30 construct, were verified through the GUS assay and the amplification of the hygromycin gene through standard PCR. The plant materials were immersed in a GUS histochemical solution. Visible blue coloration was seen on the roots and shoots of the plantlets while no blue coloration was seen in the NTplants ((A,B)).
Figure 3. Stages of potato transformation (A) Inoculation of leaf disks on MS media (B) Internode inoculation on co-cultivation media (C) Callus generation from edges of intermodal explants after 9 days of inoculation on callus medium (D) Shoot regeneration from callus cultures (E) Propagation of transformed shoots on shooting medium (F) Shoots multiplication and elongation after 4 weeks in culture (G) Culturing of excised shoots on rooting medium (H) Fully grown transformed plants.
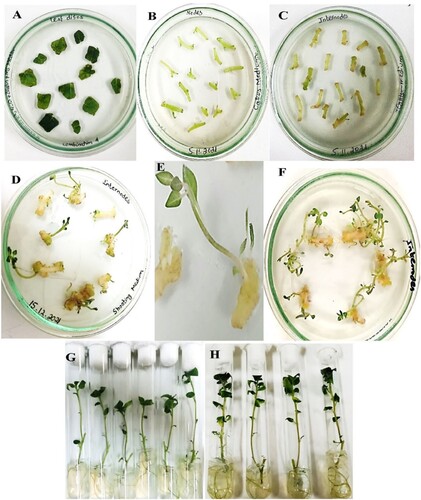
Figure 4. Confirmation of OEpotato plants GUS histochemical assay of (A1) Roots (A2) Nodes and internodes stem parts of NT potato plants as controls (B1) Roots (B 2) Nodal and internodal sections of OE potato plants. NTexplants showed no color after incubation with X-Gluc for 16 hr at 37 °C while OE explants developed a deep blue color that depicts successful potato transformation. (C) Verification of OEpotato plants by PCR amplification from genomic DNA with hygromycin (hptII) gene-specific primers. The amplified fragment corresponds to the hptII gene of 686 bp. Lane M denotes 1 kb Marker, and Lane1–L 4 contains positive OE plants.
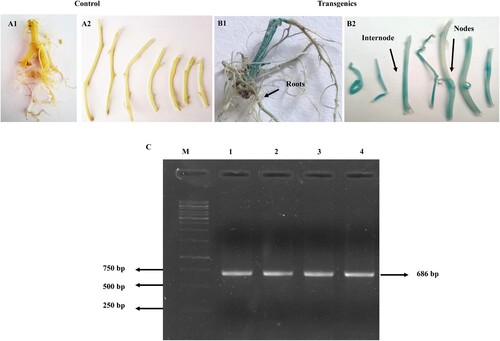
The generation of OE potato plants was further confirmed by hygromycin PCR with hygromycin-specific primers. An amplicon of 686 bp seen in the Gel Documentation System (GelDoc) confirmed the presence of the hygromycin gene in transgenic potato plants ((C)) (Supplementary Figure S2).
4.4. Drought stress induces the expression of the StDREB30 gene
The overall trends of expression analysis of StDREB30 showed that expression analysis of gene enhanced in overexpressing OE plants under drought conditions compared to NTplants. The expression was particularly high in roots compared to shoots and leaves in OE plants. The quantitative expression analysis of the StDREB30 gene in shoots ((A)) under drought and normal conditions of OEand NTplants was evaluated through qRT-PCR. On Day 0 i.e. under normal water conditions, there was no obvious difference in the expression of the StDREB30 gene in OE and NT plants. On Day 14 the expression of the StDREB30 gene in OE potato elevated and reached a maximum point almost 5 times more than the expression on Day 0. Nevertheless, after rehydration for 3 days, the expression of the StDREB30 gene drops almost 2.5 times from 4.9 folds to 1.4 folds. However, the expression of the OE plants after the recovery period was still around 1.39 folds higher than the NT plants. The expression of the StDREB30 gene was also analyzed in the leaves of OE and NT plants ((B)). The comparison between the OE and NT plants shows a clear difference in the expression level of the StDREB30 gene at specific time points during drought stress. On Day 0 there was no noticeable difference in the expression of the gene in the leaves of OE and NT plants, the expression level in both plants was 1-fold. However, a clear upregulation was seen in the transcript of the StDREB30 gene after the water withholding for 2 weeks. The OE plants showed an exponential increase in the expression of almost 3 folds higher in comparison to the expression level measured in NT plants on Day 14. The expression level in NT plants after the drought period was also 5.5 folds higher than the expression recorded on the first day. The recovery period of 3 days again causes a decrease of almost 4 fold in the expression level of StDREB30 in OE and NT plants. However, after the drought period and the recovery period, the expression of the StDREB30 gene in OE plants was more pronounced than the normal plants.
Figure 5. Overexpression of the StDREB30 gene confers drought tolerance in transgenic potato plants. Tissue-specific expression analysis of StDREB30 by qRT-PCR in response to drought stress. (A) Indicates tissue-specific expression analysis of StDREB30 in shoots (B) leaves (C) roots of OE potato plants overexpressing StDREB30 and NT plants under 14 days of drought stress followed by 3 days of rewatering. Each value is expressed as a means of 3 biological replicates. Different asterisk (*) represents significant differences (p ≤ 0.05). Statistical differences were calculated using ANOVA *P < 0.05, **P < 0.01, ***P < 0.001, ****P < 0.0001. Phenotypes of potato plants under drought stress treatment. (D) Phenotypes of controls (NT) D1-D2 and D3-D4 OE plants (E) Comparison of growth condition in controls (NT) E1-E3 and OE plants E4-E6 on the 14th day of drought stress (F) Wilting symptoms observed in F1 control (NT) in comparison to healthy F2 OE plant on the 14th day of stress.
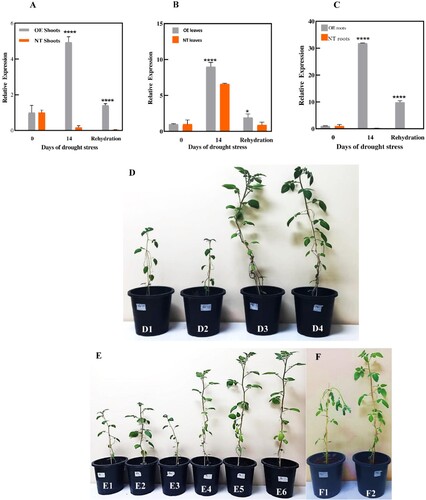
Among the differential expression of the StDREB30 gene performed in the roots, shoots and leaves of OE and NT plants, roots showed the most profound results of reaching 31 folds after 14 days of drought stress ((C)). In harmony with the previous trends, both expressions of the StDREB30 gene in the OE and NT under normal watering conditions showed an expression level of 1fold. The expression of the StDREB30 gene multiplied exponentially and reached a peak of almost 30 times higher than the expression recorded in the roots of NT plants. After rehydration for 3 days, the expression was downregulated in the roots of OE and NT plants; however, the expression was 9.8 folds more significant than that observed under watering conditions. Furthermore, we also observed the difference in the growth of OE and NT plants during drought stress ((D–F)) as overexpression of DREB has resulted in undesirable phenotypes i.e. dwarfism in many plants. Remarkable differences in phenotypes were observed between OE and NT plants after the drought interval. OE plants grew well and produced a good number of leaves under the stress period. In contrast to OE plants, the NT plants showed either wilting or stunted growth and did not recover after the three days of rehydration intervals.
4.5. Overexpression of the StDREB30 gene enhances osmotic tolerance under 20% PEG stress
The results of the expression analysis of the plant samples treated with 20% PEG 6000 showed that the OE plants tolerated the PEG stress better than non-transgenic plants. No significant phenotypic differences were observed in normal and transgenic in vitro potato plants under normal growth conditions. However, when MS liquid media was supplemented with 20% PEG 6000 for 24 hr, then clear differences were seen. In the early hours of osmotic stress, no negative effects of osmotic stress were observed on the OE and NT plants but as the stress prolonged clear signs began to show. At the end of the osmotic stress the NT plants began to wilt, their shoots started to bend, and defoliation of leaves was observed. No such symptoms were observed in OE plants, their shoots remained upright with their leaves remaining fresh and the plants showed no wilting ((A–F)). These results showed that OE plants can bear osmotic stress better than NT plants.
Figure 6. Potato plants under 20% PEG6000 osmotic stress treatment. Phenotypes of NT and OE potato plants under 20% PEG6000 stress for 24 hr. Figures (A) and (D) shows normal phenotypes of NT and OE plants at the start of PEG stress. Figures (B) and (C) shows clear wilting and defoliation of leaves in NT plants as the osmotic stress reaches 24 hr. (E) and (F) shows OE plants withstand PEG stress for 24 hr with no wilting and defoliation. qRT-PCR analysis of the StDREB30 gene in OE plants and NT plants treated with 20% PEG6000 was performed to analyze relative expression levels of the StDREB30 gene (G) shoots (H) leaves (I) roots of OE and NT plants treated with osmotic stress for 0, 3, 6,12, and 24 hr. Each value is expressed as a means of 3 biological replicates. Different asterisk (*) represents significant differences (p ≤ 0.05).
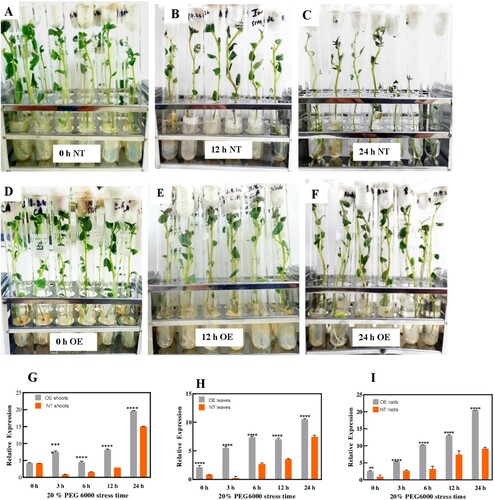
The expression level reaches a peak after 24 hr of PEG stress. The expression profile of StDREB30 in roots, shoots and leaves of OE plants showed more profound expression as compared to NT plants ((G–I)). This establishes StDREB30 as a drought stress-responsive gene. The results of the differential tissue expression analysis of the StDREB30 gene demonstrated that both OE and NT plant roots showed the highest and most steady increment in the expression of the StDREB30 gene ((I)). In roots, at 0 hr the difference in expression of the StDREB30 gene between NT and OE plants was not significant, which depicts the constitutive expression of the StDREB30 gene under normal watering conditions. At 3 hr there was a positive incline in the expression of the StDREB30 gene; however, the expression of the StDREB30 gene in OE plants was almost 2.5 higher than that of NT plants. At the 6 and 12 hr the expression of StDREB30 was 6 and 7 folds higher in OE roots than NT roots, respectively. At the final time point of 24 hr a 10-fold difference in StDREB30 expression was recorded between OE and NT plants.
The results of the expression analysis experiments obtained from the osmotic stress treatments and drought-stress treatments are parallel. At the end of stress, the expression of the StDREB30 gene in shoots of OE plants was almost folded higher than the NT plants ((G)). Similarly, a 3-fold higher expression was recorded in OE leaves ((H)) than NT while a profound 10-fold higher expression was observed in OE roots. As stated above, the expression of the StDREB30 gene relies on the length of the drought period in soil conditions. Similarly in this case the expression of genes enhanced with the extension of time of osmotic stress.
4.6. Measurement of oxidative stress parameters under water deficit conditions
To compare the antioxidant activities of OE and NT plants we measured SOD, CAT, APX, and MDA activities in potato plants during drought stress and after rehydration.
4.6.1. Increase in SOD activity under drought stress
The results ((A–C)) showed that SOD activity increased with dehydration stress with a peak on the 14th day of drought stress which indicates the SOD response against drought stress. At the start of drought stress on day 0 d the SOD activity in shoots of OE plants was 133 folds, after 14 days of drought stress it increased 143 folds and after 3 days of rewatering it dropped again to 134 folds. In NT plants the SOD activity was almost 65 folds it increased to 94 folds on Day 14 and after rewatering it falls to almost 86 folds. This result clearly shows the higher SOD activity of OE plants than that of NT plants.
Figure 7. Changes in biochemical activities in OE potato plants and NT plants during drought-stress treatment. Potato plants were exposed to drought stress treatment for 14 days followed by 3 days of rewatering for recovery. SOD activity (A, B, and C), CAT activity (D, E, and F), MDA (G, H, and I), and APX activity (J, K, and L) were measured in shoots, leaves and roots of OE and NT plants, respectively. Each value is expressed as a means of 3 biological replicates. Different asterisk (*) represents significant differences (p ≤ 0.05). Statistical differences was calculated using ANOVA *P < 0.05, **P < 0.01, ***P < 0.001, ****P < 0.0001.
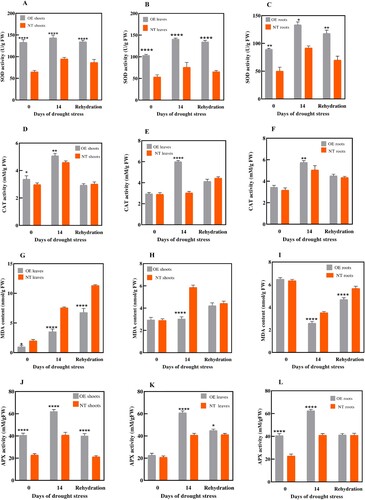
Similarly, in leaves of OE plants, the SOD activity on Day 0 was approximately 103 folds it reached its peak of 140 folds on Day 14 of drought stress and then decreased to 134 folds after rewatering. While in leaves of NT plants, the SOD activity before the drought stress was 53 folds, on Day 14 the expression was 76 folds, and after rewatering the activity recorded was 65 folds.
A similar pattern was observed in roots of OE plants, the SOD activity on Day 0, Day, 14 and after rewatering was 89, 134, and 113 folds, respectively. However, in NT plants the SOD activity at the mentioned time points was 50, 98, and 69 folds, respectively.
4.6.2. Upregulation of CAT activity under water-deficit conditions
To detect the variation in CAT levels under drought stress and normal conditions in OE StDREB30 plants and NT plants,the CAT analysis was performed. The CAT activity increased during drought stress with peak activity on the 14th day of water withholding stress. On day 0 the lowest CAT activity was recorded in both OE and NTplants. On Day 14th both the lines showed upregulation of CAT activity with OE plants showing enhanced levels as compared to NT plants, On Day 14, CAT activity measured in OE shoots, leaves and roots was 5, 5.8, and 3 folds, respectively. On Day 14, CAT activity recorded in NT shoots, leaves, and roots was 4.4, 3, and 5 folds, respectively ((D–F)). However, after 3 days of re-watering the CAT levels decreased a little in both lines. These results show that along with SOD other antioxidant enzymatic activity also upregulates to response to abiotic stresses.
4.6.3. Increase in StDREB30 overexpression lowers MDA activity in potatoes
To investigate whether StDREB30 overexpression in potatoes could scavenge toxic reactive oxygen species and alleviate the resulting damages MDA content was measured. The MDA content measured in OE leaves was 1, 4 folds and 4.5 folds lower than MDA content in NT leaves on Day 0, Day 14, and after 3 days of rewatering respectively ((G)). A similar pattern was observed in shoots, MDA content in OE shoots on Day 0, and Day 14, followed by 3 days of rewatering was almost 0.6, 14.1 and 2.24 folds lower than MDA content in NT shoots on the same time points ((H)). Correspondingly, the MDA activity measured in OE roots ((I)) was 0.1, 0.9, and 1fold lower than NT roots at Day 0, Day 14, and after 3 days of rewatering respectively. These findings depict that StDREB30 overexpression lines in potatoes reduce MDA levels, one of the causes of oxidative damage to plant cells, thus improving drought tolerance in OE potato plants.
4.6.4. StDREB30 gene overexpression increases APX activity under drought conditions
The ascorbate peroxidase activity was also measured as one of the important indicators of antioxidant activity. APX activity of OE and NT plants was measured in drought and normal conditions and compared. The results showed an elevation of enzymatic activity under stress conditions. The APX activity was highest on Day 14 of drought stress in both OE and NT plants. After 3 days of rewatering the APX activity showed downregulation as compared to the activity recorded on Day 14; however, the value was still higher than the APX activity measured on Day 0. The APX activity was around 20 times higher in OE shoots ((J)), than that of NT shoots, almost 22 folds higher in OE leaves compared to NT leaves ((K)), and almost 21 folds higher in OE roots compared to NT roots ((L)). This pattern was observed in shoots, leaves, and roots of OE and NT.
4.6.5. Transcriptional role of StDREB30 against stresses
The in silico transcriptome analysis of StDREB30 shows the upregulation or downregulation in response to various abiotic stresses The in silico expression analysis of StDREB30 was carried out against heat stress, mannitol, salt stress, 6-Benzylaminopurine (BAP), Abscisic acid (ABA), Indole-3-acetic acid (IAA), and Gibberellic acid (GA3) using eFP Browser (). The expression profiles of StDREB30 in potato group Phureja Clone DM obtained from Potato eFP Browser using default Threshold of 210 showed that upon exposure to 260 µM mannitol stress for 24 hr, StDRE30 showed an upregulation of expression of almost 114.5 folds. Similarly, StDREB30 showed an upregulation of approximately 60.23 folds upon exposure to 150 mM salt (NaCl) stress for 24 hr. The results also showed that StDREB30 show a profound response against hormonal stresses as expression of the StDREB30 gene showed an upregulation of 54.55, 29.05 and 69.75 folds when potato plants were exposed to 24 hr treatment of 10 µM BAP, 10 µM IAA, and 50 µM GA-3. However, the StDREB30 gene showed a downregulation of 64 folds in expression against 35 °C heat stress and a downregulation of 10 folds in response to 50 µM ABA stress. These findings demonstrate the diverse potential of StDREB30 in providing tolerance to plants against various major abiotic and hormonal stresses.
5. Discussion
Drought is one of the critical abiotic stresses that decrease potato productivity by limiting its growth and development (Ambrosone et al. Citation2011). Plants being sessile organisms have developed multiple strategies to cope with drought stress that involves activation of various transcription factors and changes in metabolic profile (Singh and Laxmi Citation2015). Adaptive stress tolerance mechanism of plants includes hormonal induction, stress protein synthesis, accumulation of stress-tolerant metabolites and activation of the antioxidant defense system. To minimize the adverse effects of drought stress on plants it is important to develop plant varieties that can tolerate the drought stress (Li et al. Citation2022). Advancements in the field of genetic engineering, tissue culture and genomics have resulted in the production of stress-tolerant potato plants (Munir et al. Citation2016). In this perspective, we developed drought-tolerant potato variety by the overexpression of the StDREB30 gene.
As one of the fundamental family of transcription factors, DREB plays a crucial role in countering abiotic stresses (Golldack et al. Citation2011). Despite being a widely studied transcription factor family very limited information is available on A 6 subgroup members. However, the available data on A-6 DREBs shows that these transcription factors are stress-responsive and play an important role in strengthening plants' defense response against abiotic stresses (El-Esawi and Alayafi Citation2019). Thus, it is important to study the A6 factors to comprehensively understand their functions. Hence, we focused on the A6 member of the DREB subfamily, StDREB30 to produce drought-tolerant potato variety. Plant stress tolerance to environmental stresses is an integration of physiological, biochemical, and molecular processes (Xu et al. Citation2022). Hence keeping these three aspects as the basis of the current study we comprehensively analyzed the role of the StDREB30 gene in providing drought tolerance to potato plants.
The phylogenetic analysis showed that StDREB30 showed the highest sequence (96% identity) similarity with LeDREB3, a member of the A-6 group of DREBs (). The multiple sequence alignment showed that StDREB30 consists of 1 α-helix and 3 β-sheets that are present on the major groove of DNA and help in the interaction of DREB genes with other proteins. Moreover, alignment shows the conservation of 18 amino acids out of 59 amino acids in the AP2 domain of all 6 classes of DREBs. Furthermore, a total of 20 motifs were discovered from motif analysis, out of 20 motifs only 1 motif was conserved in all DREB sequences that are found in the AP2 domain. Motif 8 seems to be unique to A-6 DREB genes while StDREB30 showed the presence of a maximum number of motifs i.e. 10. Different types of motifs help in binding to diverse proteins and forming molecular interactions that ultimately play a crucial role in gene regulation.
To achieve the objective of the generation of StDREB30 overexpressing transgenic plants, the StDREB30 gene was cloned in plant expression vector pCAMBIA 1301. The colony PCR results show that the gene was cloned in the binary vector and pCAMBIA 1301:: StDREB30 construct was successfully generated (). Two separate protocols were optimized to transfer pCAMBIA 1301:: StDREB30 construct in potato plants Leaf discs, nodes and internodes were used as explants for the agrobacterium-mediated transformation of potato plants (). As the StDREB30::pCAMBIA 1301 construct contains the GUS gene, so GUS histochemical assay was carried out to confirm the presence of binary construct in transgenic plants. The positive transformed potato plants showed blue coloration in different parts of the plant depicting positive GUS activity. Furthermore, the generation of transgenic plants was also confirmed through hygromycin PCR. Both GUS assay and hygromycin PCR confirmed the generation of StDREB30 transgenic plants ().
After the successful generation of OE potato plants, the in vitro OE and NT plants were transferred from test tubes to soil and after acclimatization, in glass house conditions drought stress of 14 days was imposed on potato plants . Our expression analysis results clearly indicate that the expression level of the StDREB30 gene was low in NT plants as compared to OE plants in drought conditions (). Moreover, OE potato plants displayed enhanced drought tolerance while NT potato plants showed significantly reduced drought tolerance. The overall pattern observed in OE and NT plants was that in well-watered (normal) conditions at Day 0 no significant change in expression level was recorded between the OE and NT potato plants. However, as the water was withheld and drought stress was imposed for 14 days on OEand NT potato plants, expression of the StDREB30 gene upregulated almost 30 folds in roots, 5 folds in shoots and 3 folds in leaves of OE potato plants as compared to NTplants. However, a decline in StDREB30 expression was seen in OE and NT plants after the rehydration of 3 days nevertheless the expression in OE shoots, leaves, and roots was still 1.39 folds, 4 folds, and 9.8 folds higher than NTplants ((A–C)). Constitutive expression of the StDREB30 gene suggests that this gene may have a major role in potato plants throughout their life cycle. The higher expression of StDREB30 in roots suggests the crucial role of the DREB gene in drought adaptation. Roots are considered model organs for the study of drought genetics due to their role as primary organs for perceiving water deficiency signals and direct involvement (Liu and Qin Citation2021). Previous studies have shown higher expression of DREB genes such as AtDREB, ZmDREB, TaDREB, and OsDREB in root tissue compared to shoots and leaves. Apart from DREBs NAC, NHX, MYB and WRKY showed higher expression in roots (Janiak et al. Citation2016). Water shortage signals are first produced in roots and then the drought-responsive genes get activated, these signals are then passed to aerial parts via signal transduction and a complete pathway is triggered. Thus, the gene expression profiling in roots can be used for breeding and for genetic engineering to produce drought-tolerant crops.
Many of the previously reported DREB genes that have successfully enhanced the tolerance of transgenic plants to various abiotic stresses however, have compromised the growth of transgenic plants in comparison to wild-type plants (Liao et al. Citation2017). These undesirable phenotypes limit the practical utilization of economically and nutritionally important drought-tolerant transgenic crops. Thus, it is extremely important to predict and avoid the undesirable effects of DREB transcription factors in transgenic plants. In current the research, no such stunted growth in transgenic potatoes has been observed ((D–F)). In contrast, OEpotato plants overexpressing StDREB30 not only survived the 14-day drought stress but showed more vigorous growth than NTplants. They developed tall, thick stems, and green expanded leaves. No yellowing of leaves or wilting was observed in any of the OEpotato plants. On the other hand, NTpotato plants were unable to withstand drought stress of 14 days. NTplants could not grow well in drought conditions and even showed severe wilting at the end of the drought span. Hence, we successfully enhanced the drought-tolerant capacity of potato plants without compensating for their growth and development. Our results are consistent with the results of an experiment conducted by (Kudo et al. Citation2017), drought tolerance as well as plant growth was improved by overexpressing dehydration element-binding 1A (DREB 1A) and phytochrome interacting factor-like 1 (OsPIL1) in Arabidopsis. The transgenic plants grew well under 3weeks of drought stress and showed no symptoms of dwarf phenotype. Similarly, in another study drought tolerance in transgenic soyabean was enhanced by the overexpression of the GmDREB1 gene (Jia et al. Citation2023). We further explored the effects of osmotic stress on transgenic plants in in vitro conditions by exposing plants to 20% PEG 6000 for 24 hr. The phenotypic and qPCR results obtained from PEG stress were strikingly similar to the results of drought stress. OEpotato plants did not show any significant phenotypic abnormalities during or at the end of 24 hr osmotic stress (). At the end of osmotic stress, the shoots of OEpotato plants were upright, leaves were green and clearly no wilting was observed in any of the OEplants under osmotic stress. On the other hand, NTplants showed signs of shoot wilting and major defoliation after 12 hr of osmotic stress. After 24 hr of osmotic stress, NT had either wilted or defoliated. These results further validate that the StDREB30 gene had enhanced the osmotic stress of OEplants.
The tissue-specific expression analysis results of the StDREB30 gene from the roots, shoots and leaves of OE and NT plants at 0, 3, 6, 12, and 24 hr of PEG stress showed the upregulation of the StDREB30 gene with the increase in time of osmotic stress ((G–I)). In shoots the expression of the StDREB30 gene was almost equal in OE and NT plants, however, OE leaves and roots showed a higher expression of the StDREB30 gene at the start of PEG stress. After 3 hr of PEG stress, NTroots showed upregulation in gene expression while shoots and leaves showed a reduction in the expression of the StDREB30 gene. However, after 6 hr shoots, roots, and leaves exhibit an upregulation of the StDREB30 gene with its expression reaching a maximum at 24 hr. In the case of OE plants, a prolific upregulation in the expression of the StDREB30 gene was observed in roots, shoots and leaves with stress time except in OEleaves. At 3 hr of 20% PEG 6000 stress, the expression of StDREB30 in OE leaves was 7.4 folds, at 6 hr of stress the expression of gene downregulated to 4.4 folds; however, at 12 hr of stress, the expression of StDREB30 gene once again shows an upregulation and reaches to 5.4 folds. In OE shoots, at 0 hr of osmotic stress, StDREB30 showed expression of 2.1 folds, at 6 hr of stress an increase in the expression of the gene was observed and the expression recorded was 5.4 folds. Nevertheless, in OE shoots the expression of the gene remains almost constant from 6 to 12 hr with the expression of almost 7 folds at both time points, however, gene expression increased after 24 hr of PEG stress and reached 10 folds. OEroots showed the highest expression of the StDREB30 gene with its expression increased from 2.5 folds at 0 hr of osmotic stress to 20 folds at 24 hr of PEG stress. Thus, expression analysis results demonstrate that StDREB30 expression also depends on the period of osmotic stress.
Such results were obtained in experiments conducted to investigate the drought tolerance of transgenic Arabidopsis overexpressing the DREB10 gene (Li et al. Citation2019). They performed osmotic stress by supplementing MS media with 250 Mm Mannitol for 7 days. The plants overexpressing DREB10 showed better germination and growth rate than normal in vitro Arabidopsis plants. Similarly, the RT–PCR analysis showed the upregulation of the DREB10 gene in OE plants under osmotic stress than the normal plants. Similar results were obtained in studies performed to promote drought tolerance in tobacco plants through the overexpression of the StDREB1 gene (Hezema et al. Citation2021). Transgenic tobacco exhibited better osmotic tolerance to 20% PEG 6000 stress than control plants and the expression analysis results showed higher expression of the StDREB1 gene in transgenics than control plants after 24 hr of stress. Plants respond to environmental stresses by increasing their cellular metabolism which leads to cause the production of reactive oxygen species (ROS) which includes hydrogen peroxide (H2O2), superoxide (O2−) and hydroxyl ions (OH) (Saed-Moucheshi et al. Citation2014). High concentration of these active molecules causes damage to nucleic acids, proteins, lipids, and cell membranes causing metabolic disorders. Antioxidants play a crucial role in scavenging the harmful radical oxygen (ROS) species produced during drought stress. Thus, plants have developed a complete antioxidant system as a defense mechanism to minimize or eliminate the harm caused to cells by reactive oxygen species under stress conditions like drought (Yusuf et al. Citation2012). The highly developed antioxidant system of plants consists of enzymes such as superoxide dismutase (SOD), ascorbate peroxidase (APX), catalase (CAT), peroxidase (POD), and non-enzymatic molecules such as β-carotene, vitamin C, and vitamin E (Gill et al. Citation2015).
Under drought-stress plant varieties with better drought tolerance showed high activity of SOD, APX, and CAT compared to those varieties which have weak tolerance to drought stress as reported in wheat (Chen et al. Citation2008) and sweet sorghum (Lu and Guo Citation2010). The enzymatic activity i.e. SOD showed a higher trend in both OE and NT plants under drought stress. SOD is an important antioxidant enzyme found in various organisms such as plants, animals, and microorganisms. The SOD level in plants is an indicator of ageing and death thus, it plays an important role in the plant antioxidant system which help plants to scavenge ROS against various stresses. A recent study revealed DaSOD1 gene increased SOD levels in transgenic tobacco to detoxify harmful radicals and provide protection to plants against cadmium stress (Hua et al. Citation2024). To investigate the effect of overexpression of StDREB30 on enzymatic activity under drought conditions, the SOD activity of OE plants and NT plants were compared. The results ((A–C)) showed that SOD activity increased with dehydration stress with a peak on the 14th day of drought stress which indicates the SOD response against drought stress.
CAT is an enzyme that catalyzes the decomposition reaction of hydrogen peroxide into oxygen and water. It is found in the peroxisome of cells and accounts for 40% of the total peroxidase enzyme (Guan et al. Citation2009). Catalase results were in harmony with SOD activity results, both OE and NT plants showed the highest catalase activity at the end of drought stress however the catalase activity of OE plants was higher than NT plants ((D–F)). The high catalase activity of transgenic plants shows the high free radical scavenging ability of OEplants as compared to NTplants.
Drought stress triggers the increased production of harmful free radicals, these radicals ultimately attack cellular macromolecules like membrane lipids causing oxidative damage to the cellular structures. The reactions of free radicals with macromolecules results in the production of MDA which is extremely toxic to plant cells (Wang et al. Citation2023). Accumulation of MDA causes cell and membrane damage, and the level of MDA positively correlates with the degree of membrane lipid peroxidation ultimately reflecting the extent of damage occurred in plants under stress conditions. Drought-tolerant tobacco varieties accumulated less MDA content in leaves while drought-sensitive tobacco varieties accumulated high MDA content, and tobacco accumulated more MDA in drought conditions than in normal watering conditions (Wu et al. Citation2007). DREB1B overexpression significantly enhanced the tolerance of cotton to low temperatures while silencing made cotton sensitive to cold stress. Overexpression of DREB1B activated cold-responsive downstream genes, enhanced antioxidant activities and decreased MDA accumulation in cells (Wang et al. Citation2021). The results of the current study showed less accumulation of MDA in OEplants than in NTplants under drought and normal conditions, indicating the high susceptibility of non-transgenic plants to damage caused by MDA.
APX is another important antioxidant enzyme of the plant defense system that plays a major role in protecting plants against adverse effects of superoxide radicals produced during drought stress. APX catalyzes the conversion of superoxide radicals to hydrogen peroxide and ultimately to water and oxygen thus protecting plants from detrimental cytotoxic effects (Asada Citation1999). At normal conditions, both OE and NT plants showed almost the same APX activity; however, after drought stress the APX activity in OE potato plants was enhanced as compared to NT plants which provided plants with the extra radical scavenging ability and better adaptation to potato plants in drought stress. These results strongly support the fact that StDREB30 overexpression in potato plants also improves the activity of antioxidant enzymes during drought periods, which ultimately improves drought tolerance. These differential drought responses lead to better drought tolerance of transgenic potato overexpressing the StDREB30 gene, indicative through the degree of wilting. The in-silico expression analysis () shows the response of StDREB30 against various other abiotic stresses. The results show higher expression of StDREB30 against mannitol and salt stress, which are the other major abiotic factors limiting plant growth. The results also showed the high response of StDREB30 against hormonal stresses mainly ABA, GA3, and BAP which suggests a crosstalk between StDREB30 and hormonal pathways and the role of StDREB30 in various signaling pathways indicating its diverse regulatory mechanism. Moreover, these results suggest the generation of multi-stress tolerant potato plants through the overexpression of a single gene.
6. Conclusion
The current research comprehensively covers the comprehensive characterization of StDREB30, a member of the A-6 subgroup of the DREB gene family in potatoes. Our research findings demonstrated that transgenic plants overexpressing the StDREB30 gene exhibited better growth patterns and appeared to be able to resist the oxidative stress brought on by drought stress through the induction of stress-related genes, the activation of antioxidant machinery to scavenge dangerous ROS and the accumulation of osmoprotectant molecules. In contrast to NT lines, which exhibited severe stunted growth and wilting, OE potato plants displayed tolerance to a 14-day drought period. The expression analysis revealed that StDREB30 expression was high in OE lines under drought stress, compared to NT lines. The transgenic roots showed particularly high StDREB30 expression both in soil and in vitro conditions under PEG 6000 stress for a period of 24 hr. The biochemical analysis revealed high antioxidant activities in plants, such as increased SOD, CAT, and APX activity and decreased MDA activity, which helped the plants withstand drought stress by increasing antioxidant enzyme activity and reducing lipid peroxidation brought on by ROS. The current study suggests that StDREB30 is a potential candidate for the development of multi-stress-tolerant crops. Furthermore, extensive field trials of transgenic potatoes are required to determine the threshold level of drought stress that the transgenic potato can tolerate in actual field conditions and show better growth and yield than its wild-type counterparts in harsh environmental conditions. Successful field trials can form the basis for the commercialization of transgenic potatoes overexpressing the StDREB30 gene.
Author contributions
F.M. planned and designed the research project; Q.A. . performed the experiments; Q.A. and F.M. characterized, and analyzed the data; Q.A. and F.M. prepared the manuscript. F.M., Q.A., M.T., A.G,. and R.A reviewed and edited the manuscript. All authors read and approved this manuscript.
Supplemental Material
Download MS Word (391.9 KB)Acknowledgements
We acknowledge the National University of Sciences and Technology (NUST), Pakistan, for providing research facilities. We are thankful to the Higher Education Commission (HEC) of Pakistan. This work was supported by the National Research Program for Universities by HEC Pakistan.
Disclosure statement
No potential conflict of interest was reported by the author(s).
Data availability statement
All data supporting the findings of this study are available within the paper.
Additional information
Funding
Notes on contributors
Qurat- Ul Ain-Ali
Qurat-Ul Ain-Ali is a PhD student; she is the part of Plant Molecular Biology Research Group led by Dr. Faiza Munir..
Faiza Munir
Dr. Faiza Munir is currently working as a Tenured Associate Professor at Atta ur Rahman School of Applied Biosciences, NUST, Pakistan. She is the principal investigator of this research project. She is the recipient of the NRPU grant No. 10055; awarded by the Higher Education Commission of Pakistan. Her research focus is on the development of stress tolerant overexpressive crop varieties mainly Solanaceae members and elucidation of transcriptional activation analysis of stress regulatory genes in transgenic lines. She utilizes interdisciplinary techniques, including biochemistry, genetic engineering, genomics to elucidate the regulatory mechanism governing stress regulatory patterns in plants.
Muhammad Tahir
Dr. Muhammad Tahir is currently working as a Professor at Atta ur Rahman School of Applied Biosciences, NUST, Pakistan.
Rabia Amir
Dr. Rabia Amir is currently working as Associate Professor at Atta ur Rahman School of Applied Biosciences, NUST, Pakistan.
Alvina Gul
Dr. Alvina Gul is currently working as Associate Professor at Atta ur Rahman School of Applied Biosciences, NUST, Pakistan.
References
- Ain-Ali Q-U, Mushtaq N, Amir R, Gul A, Tahir M, Munir F. 2021. Genome-wide promoter analysis, homology modeling and protein interaction network of Dehydration Responsive Element Binding (DREB) gene family in solanum tuberosum. PLoS One. 16:e0261215. https://doi.org/10.1371/journal.pone.0261215.
- Akhtar M, Jaiswal A, Taj G, Jaiswal JP, Qureshi MI, Singh NK. 2012. DREB1/CBF transcription factors: their structure, function and role in abiotic stress tolerance in plants. J Genet. 91:385–395. doi:10.1007/s12041-012-0201-3.
- Ambrosone A, Costa A, Martinelli R, Massarelli I, De Simone V, Grillo S, Leone A. 2011. Differential gene regulation in potato cells and plants upon abrupt or gradual exposure to water stress. Acta Physiol Plant. 33:1157–1171. doi:10.1007/s11738-010-0644-1.
- Asada K. 1999. The water-water cycle in chloroplasts: scavenging of active oxygens and dissipation of excess photons. Annu Rev Plant Physiol Plant Mol Biol 50:601–639. doi:10.1146/annurev.arplant.50.1.601.
- Baoxiang W, Zhiguang S, Yan L, Bo X, Jingfang L, Ming C, Yungao X, Bo Y, Jian L, Jinbo L. 2023. A pervasive phosphorylation cascade modulation of plant transcription factors in response to abiotic stress. Planta. 258:73. doi:10.1007/s00425-023-04232-x.
- Cakmak I, Marschner HJP. 1992. Magnesium deficiency and high light intensity enhance activities of superoxide dismutase, ascorbate peroxidase, and glutathione reductase in bean leaves. Plant Physiol. 98:1222–1227.
- Chen J-Q, Meng X-P, Zhang Y, Xia M, Wang X-P. 2008. Over-expression of OsDREB genes lead to enhanced drought tolerance in rice. Biotechnol Lett. 30:2191–2198. doi:10.1007/s10529-008-9811-5.
- Chen Y, Huang Q, Hua X, Zhang Q, Pan W, Liu G, Yu C, Zhong F, Lian B, Zhang J. 2023. A homolog of AtCBFs, SmDREB A1-4, positively regulates salt stress tolerance in Arabidopsis thaliana and Salix matsudana. Plant Physiol Biochem. 202:107963. doi:10.1016/j.plaphy.2023.107963.
- Dahal K, Li X-Q, Tai H, Creelman A, Bizimungu B. 2019. Improving potato stress tolerance and tuber yield under a climate change scenario–a current overview. Front Plant Sci. 10:563. doi:10.3389/fpls.2019.00563.
- Du X, Li W, Sheng L, Deng Y, Wang Y, Zhang W, Yu K, Jiang J, Fang W, Guan Z. 2018. Over-expression of chrysanthemum CmDREB6 enhanced tolerance of chrysanthemum to heat stress. BMC Plant Biol. 18:1–10.
- El-Esawi M, Alayafi A. 2019. Overexpression of StDREB2 transcription factor enhances drought stress tolerance in cotton (Gossypium barbadense L.). Genes (Basel). 10:142. doi:10.3390/genes10020142.
- FAOSTAT. 2022. https://www.fao.org/faostat/en/#data/QCL/visualize.
- Gill SS, Anjum NA, Gill R, Yadav S, Hasanuzzaman M, Fujita M, Mishra P, Sabat SC, Tuteja N. 2015. Superoxide dismutase—mentor of abiotic stress tolerance in crop plants. Environ Sci Pollut Res. 22:10375–10394. doi:10.1007/s11356-015-4532-5.
- Golldack D, Lüking I, Yang O. 2011. Plant tolerance to drought and salinity: stress regulating transcription factors and their functional significance in the cellular transcriptional network. Plant Cell Rep. 30:1383–1391. doi:10.1007/s00299-011-1068-0.
- Guan Z, Chai TY, Zhang YX, Xu J, Wei W. 2009. Enhancement of Cd tolerance in transgenic tobacco plants overexpressing a Cd-induced catalase cDNA. Chemosphere. 76:623–630. doi:10.1016/j.chemosphere.2009.04.047.
- Hezema YS, Shukla MR, Ayyanath MM, Sherif SM, Saxena PK. 2021. Physiological and molecular responses of six apple rootstocks to osmotic stress. Int J Mol Sci. 22:8263. doi:10.3390/ijms22158263.
- Hua S, Lin H-H, Huang Z, Wang M, Li Q, Chen S-P. 2024. Cloning and overexpression of the DaSOD1 gene from Dioscorea alata improves cadmium resistance in transgenic tobacco plants. J Plant Interact. 19:2300513. doi:10.1080/17429145.2023.2300513.
- Hwang E-W, Shin S-J, Yu B-K, Byun M-O, Kwon H-B. 2011. Mir171 family members are involved in drought response in Solanum tuberosum. J Plant Biol. 54:43–48. doi:10.1007/s12374-010-9141-8.
- Janiak A, Kwaśniewski M, Szarejko I. 2016. Gene expression regulation in roots under drought. J Exp Bot. 67:1003–1014. doi:10.1093/jxb/erv512.
- Jefferson RA. 1989. The GUS reporter gene system. Nature. 342:837–838. doi:10.1038/342837a0.
- Jia X, Qi E, Liu S, Ma S, Wen G, Zhang X, Lv H, Huang W, Zhang X. 2023. Physiological and transcriptomic analysis reveals drought-stress responses of arabidopsis DREB1A in transgenic potato. Potato Res. 66(4):1143–1164.
- Kizis D, Lumbreras V, Pagès M. 2001. Role of AP2/EREBP transcription factors in gene regulation during abiotic stress. FEBS Lett. 498:187–189. doi:10.1016/S0014-5793(01)02460-7.
- Kudo M, Kidokoro S, Yoshida T, Mizoi J, Todaka D, Fernie AR, Shinozaki K, Yamaguchi-Shinozaki K. 2017. Double overexpression of DREB and PIF transcription factors improves drought stress tolerance and cell elongation in transgenic plants. Plant Biotechnol J. 15:458–471. doi:10.1111/pbi.12644.
- Lata C, Bhutty S, Bahadur RP, Majee M, Prasad M. 2011. Association of an SNP in a novel DREB2-like gene SiDREB2 with stress tolerance in foxtail millet [Setaria italica (L.)]. J Exp Bot. 62:3387–3401. doi:10.1093/jxb/err016.
- Li Q, Huang Z, Deng C, Lin K-H, Hua S, Chen S-P. 2022. Endophytic Klebsiella sp. San01 promotes growth performance and induces salinity and drought tolerance in sweet potato (Ipomoea batatas L.). J Plant Interact. 17:608–619. doi:10.1080/17429145.2022.2077464.
- Li S, Zhao Q, Zhu D, Yu J. 2018. A DREB-like transcription factor from maize (Zea mays), ZmDREB4. 1, plays a negative role in plant growth and development. Front Plant Sci. 9:395. doi:10.3389/fpls.2018.00395.
- Li X, Liang Y, Gao B, Mijiti M, Bozorov TA, Yang H, Zhang D, Wood AJ. 2019. ScDREB10, an A-5c type of DREB gene of the desert moss Syntrichia caninervis, confers osmotic and salt tolerances to Arabidopsis. Genes (Basel). 10:146. doi:10.3390/genes10020146.
- Liao X, Guo X, Wang Q, Wang Y, Zhao D, Yao L, Wang S, Liu G, Li T. 2017. Overexpression of Ms DREB 6.2 results in cytokinin-deficient developmental phenotypes and enhances drought tolerance in transgenic apple plants. Plant J. 89:510–526. doi:10.1111/tpj.13401.
- Liu S, Qin F. 2021. Genetic dissection of maize drought tolerance for trait improvement. Mol Breed. 41:1–13.
- Lu J, Guo T. 2010. Effects of water stress on activities of protective enzyme and physiological characteristics in seedlings of two varieties of sweet sorghum. Agric Res Arid Areas. 28:89–93.
- Majeed Y, Zhu X, Zhang N, Rasheed A, Tahir MM, Si H. 2022. Functional analysis of mitogen-activated protein kinases (MAPKs) in potato under biotic and abiotic stress. Mol Breed. 42:31. doi:10.1007/s11032-022-01302-y.
- Mao L, Deng M, Jiang S, Zhu H, Yang Z, Yue Y, Zhao K. 2020. Characterization of the DREBA4-type transcription factor (SlDREBA4), which contributes to heat tolerance in tomatoes. Front Plant Sci. 11:554520. doi:10.3389/fpls.2020.554520.
- Maqsood H, Munir F, Amir R, Gul A. 2022. Genome-wide identification, comprehensive characterization of transcription factors, cis-regulatory elements, protein homology, and protein interaction network of DREB gene family in solanum lycopersicum. Front Plant Sci. 13:1031679. doi:10.3389/fpls.2022.1031679.
- Mellacheruvu S, Tamirisa S, Vudem DR, Khareedu VR. 2016. Pigeonpea hybrid-proline-rich protein (CcHyPRP) confers biotic and abiotic stress tolerance in transgenic rice. Front Plant Sci. 6:1167. doi:10.3389/fpls.2015.01167.
- Munir F, Hayashi S, Batley J, Naqvi SMS, Mahmood T. 2016. Germin-like protein 2 gene promoter from rice is responsive to fungal pathogens in transgenic potato plants. Funct Integr Genom. 16:19–27. doi:10.1007/s10142-015-0463-y.
- Munir F, Naqvi SMS, Mahmood T. 2011. In vitro culturing and assessment of somaclonal variation of Solanum tuberosum var. desiree. Turkish J Biochem. 36:296–302.
- Mushtaq N, Munir F, Gul A, Amir R, Paracha RZ. 2021. Genome-wide analysis, identification, evolution and genomic organization of dehydration responsive element-binding (DREB) gene family in solanum tuberosum. PeerJ. 9:e11647. doi:10.7717/peerj.11647.
- Onele AO, Mazina AB, Leksin IY, Minibayeva FV. 2023. DsDBF1, a type A-5 DREB gene, identified and characterized in the Moss Dicranum scoparium. Life. 13:90. doi:10.3390/life13010090.
- Pino M-T, Skinner JS, Park E-J, Jeknić Z, Hayes PM, Thomashow MF, Chen THH. 2007. Use of a stress inducible promoter to drive ectopic AtCBF expression improves potato freezing tolerance while minimizing negative effects on tuber yield. J Plant Biotechnol. 5:591–604. doi:10.1111/j.1467-7652.2007.00269.x.
- Ren Q, Chen X, Zhang S, Jia Y, Duan W, Yang T. 2009. Comparison of drought resistance characteristics of different flue-cured tobacco varieties. Acta Bot Sin. 29:2019–2025.
- Saed-Moucheshi A, Shekoofa A, Pessarakli M. 2014. Reactive oxygen species (ROS) generation and detoxifying in plants. J Plant Nutr. 37:1573–1585. doi:10.1080/01904167.2013.868483.
- Sazegari S, Niazi A. 2012. Isolation and molecular characterization of wheat (‘triticum aestivum’) dehydration responsive element binding factor (DREB) isoforms. Aust J Crop Sci. 6:1037–1044.
- Shinozaki K, Yamaguchi-Shinozaki K. 2006. Gene networks involved in drought stress response and tolerance. J Exp Bot. 58:221–227. doi:10.1093/jxb/erl164.
- Singh D, Laxmi A. 2015. Transcriptional regulation of drought response: a tortuous network of transcriptional factors. Front Plant Sci. 6:895.
- Srivastava R, Kumar R. 2019. The expanding roles of APETALA2/ethylene responsive factors and their potential applications in crop improvement. Brief Funct Genom. 18:240–254. doi:10.1093/bfgp/elz001.
- Sun X-B, Ma H-X, Jia X-P, Chen Y, Ye X-Q. 2015. Molecular cloning and characterization of two novel DREB genes encoding dehydration-responsive element binding proteins in halophyte Suaeda salsa. J Genet Genom. 37:199–212.
- Tang Y, Bao X, Zhi Y, Wu Q, Guo Y, Yin X, Zeng L, Li J, Zhang J, He W. 2019. Overexpression of a MYB family gene, OsMYB6, increases drought and salinity stress tolerance in transgenic rice. Front Plant Sci. 10:168. doi:10.3389/fpls.2019.00168.
- Wang H, Li N, Li H, Zhang S, Zhang X, Yan X, Wang Z, Yang Y, Zhang S. 2023. Overexpression of NtGCN2 improves drought tolerance in tobacco by regulating proline accumulation, ROS scavenging ability, and stomatal closure. Plant Physiol Biochem. 198:107665. doi:10.1016/j.plaphy.2023.107665.
- Wang Y, Wang Y, Meng Z, Wei Y, Du X, Liang C, Zhang R. 2021. Elevation of GhDREB1B transcription by a copy number variant significantly improves chilling tolerance in cotton. Planta. 254:1–10.
- Wu L, Chen X, Ren H, Zhang Z, Zhang H, Wang J, Wang X-C, Huang R. 2007. ERF protein JERF1 that transcriptionally modulates the expression of abscisic acid biosynthesis-related gene enhances the tolerance under salinity and cold in tobacco. Planta. 226:815–825. doi:10.1007/s00425-007-0528-9.
- Xu X, Liu W, Liu X, Cao Y, Li X, Wang G, Fu C, Fu J. 2022. Genetic manipulation of bermudagrass photosynthetic biosynthesis using Agrobacterium-mediated transformation. Physiol Plant. 174:e13710. doi:10.1111/ppl.13710.
- Yoshimura K, Yabuta Y, Ishikawa T, Shigeoka S. 2000. Expression of spinach ascorbate peroxidase isoenzymes in response to oxidative stresses. J Plant Physiol. 123:223–234. doi:10.1104/pp.123.1.223.
- Yusuf M, Fariduddin Q, Varshney P, Ahmad A. 2012. Salicylic acid minimizes nickel and/or salinity-induced toxicity in Indian mustard (Brassica juncea) through an improved antioxidant system. Environ Sci Pollut Res. 19:8–18. doi:10.1007/s11356-011-0531-3.
- Zhang X. 1992. The measurement and mechanism of lipid peroxidation and SOD, POD and CAT activities in biological system. Research methodology of crop physiology. Agriculture press, Beijing. 208–211.
- Zhang X, Liu X, Wu L, Yu G, Wang X, Ma H. 2015. The SsDREB transcription factor from the succulent halophyte Suaeda salsa enhances abiotic stress tolerance in transgenic tobacco. Int J Genom. 2015(1):875497. doi:10.1155/2015/875497.