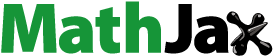
ABSTRACT
Miscanthus sinensis Andersson naturally grows at mining sites and accumulates heavy metals, suggesting heavy metal tolerance. This study aimed to elucidate the heavy metal tolerance mechanisms of M. sinensis, which naturally grew at a mine site, considering root endophytes. Elemental analysis by inductively coupled plasma-optical emission spectroscopy showed that M. sinensis highly accumulated zinc and lead in adventitious roots and dead root bark, exhibiting tolerance via these elemental removals in dead root bark. Phenolic analysis by high-performance liquid chromatography revealed chlorogenic acid production in adventitious roots. Phialocephala fortinii, which showed the highest appearance, and Talaromyces verruculosus, which produced gluconic acid as a zinc detoxicant, were isolated from adventitious roots of M. sinensis. In inoculation tests, P. fortinii and T. verruculosus significantly enhanced seedling growth by increasing potassium uptake and possibly indole-3-acetic acid production. Our results provide fundamental knowledge for using M. sinensis and root endophytes for revegetation at mining sites.
1. Introduction
Ore mining is a significant economic activity in the human society; however, it leads to the release of large amounts of heavy metals into the environment. Heavy metal is generally defined as a kind of metallic element that its density of more than 5 g/cm3 (Järup Citation2003). Recently, several researchers have been using the term ‘potentially toxic element’ to replace heavy metal when discussing environmental contamination (Pourret and Hursthouse Citation2019; Khodaverdiloo et al. Citation2020). Some heavy metals, such as copper (Cu) and zinc (Zn), are micro-essential nutrients to plants but can be detrimental in high concentrations (Arif et al. Citation2016). On the other hand, heavy metals, like cadmium (Cd) and lead (Pb), are generally toxic to plants even at low level (Kabata-Pendias Citation2011). Therefore, excessive heavy metals in the soil from mining sites pose serious environmental problems.
Generally, high concentrations of heavy metals cause a variety of toxic symptoms and are detrimental to normal plants (Kabata-Pendias Citation2011); however, some natural vegetation could be found at mining sites and serpentine soils, suggesting that plants potentially evolved diverse heavy metal tolerance (Baker Citation1981; Ernst et al. Citation1992; Larcher Citation2003) to adapt to heavy metal-enriched environment. Miscanthus sinensis Andersson, a perennial grass plant of the Gramineae family native to eastern Asia, grows naturally at several mining sites of Japan (Hiroi Citation1974, Citation1980), China (Zhao et al. Citation2013), and Korea (Babu et al. Citation2015). Several studies using element-added hydroponics or pot experiments have demonstrated that M. sinensis can resist metals and metalloids, such as aluminum (Al), chromium (Cr), Zn (Ezaki et al. Citation2008), Cd and arsenic (As) (Babu et al. Citation2015; Jiang et al. Citation2018), mercury (Hg) (Zhao et al. Citation2019), and antimony (Sb) (Xue et al. Citation2015) in the roots. Furthermore, M. sinensis, which grows naturally at mining sites, has also been found to accumulate high concentrations of metals in its roots, such as Al (Haruma et al. Citation2018), Pb, Zn (Sun et al. Citation2021), As and Sb (Xue et al. Citation2014). The detoxification of Al in M. sinensis could made by productions of root exudates, such as citric acid (Kayama Citation2001), chlorogenic acid, malic acid and trans-aconitic acid (Haruma et al. Citation2018). Several previous studies have found the heavy-metal accumulation and tolerance in M. sinensis under hydroponic experiments (Ezaki et al. Citation2008; Xue et al. Citation2015), pot experiments (Babu et al. Citation2015; Jiang et al. Citation2018; Zhao et al. Citation2019), and the habitats in the field (Xue et al. Citation2014; Haruma et al. Citation2018; Sun et al. Citation2021); however, the heavy metal tolerance mechanisms of M. sinensis, which grows naturally at mining sites, are not clearly clarified yet.
Wilson (Citation1995) showed the definition of endophytes as follows; endophytes are fungi or bacteria which, for all or part of their life cycle, invade the tissues of living plants and cause unapparent and asymptomatic infections entirely within plant tissues but cause no symptoms of disease. Recent studies have reported the benefits of endophytes on host plants under environmental stresses such as drought, salinity (Sherameti et al. Citation2008; Waqas et al. Citation2012), and heavy metals (Aly et al. Citation2011; Deng and Cao Citation2017). Dark septate endophytes (DSEs), a class of root endophytes with partially or entirely melanized septate hyphae (Stoyke and Currah Citation1991; Rodriguez et al. Citation2009), have been clarified to enhance heavy metal tolerance in plants (Li et al. Citation2011; Wang et al. Citation2016; Yamaji et al. Citation2016a; Jin et al. Citation2018; Haruma et al. Citation2021). Regarding M. sinensis, Haruma et al. (Citation2018, Citation2021) demonstrated that the root endophytic fungi Chaetomium cupreum and Phialocephala fortinii, isolated from M. sinensis, enhanced the growth and Al tolerance of the host plant in mine soil. Moreover, C. cupreum produced oosporein, a low-molecular-weight organic compound that chelated with Al, which chemically contributed to the Al tolerance of M. sinensis (Haruma et al. Citation2019). Therefore, it is worth considering the effects of root endophytes on the heavy metal tolerance of M. sinensis.
The objective of the present study was to clarify the heavy metal tolerance mechanisms of M. sinensis growing at a mine site, in association with root endophytes, via (1) evaluation of the heavy metal translocation and bio-accumulation abilities of M. sinensis, calculated by heavy metal concentrations in the root-zone soil and in each plant tissue; (2) analysis of detoxicant production in adventitious root extracts; and (3) isolation of root endophytes and examination of endophytic detoxicant production by high-appearance root endophytes. Among the isolated root endophytes, Phialocephala fortinii C. J. K. Wang & H. E. Wilcox and Talaromyces verruculosus (Peyronel) Samson, Yilmaz, Frisvad & Seifert exhibited the highest appearance and high detoxicant production, respectively; therefore, we conducted an inoculation test using sterilized M. sinensis seedlings inoculated with P. fortinii or T. verruculosus to confirm the fungal effect on heavy metal tolerance of M. sinensis. In addition, a Zn detoxicant produced by T. verruculosus was identified. Finally, we discussed the potential of using M. sinensis and root endophytes for revegetation at the mining sites.
2. Materials and methods
2.1. Sampling, and analyses of root-zone soil and plant tissues
Miscanthus sinensis grows naturally at a Zn and Pb mining site located in Honshu Island, Japan. Five individuals were randomly selected from the study site in August 2018. The root-zone soil (200 mm × 200 mm × 100 mm) together with the roots was collected. After separating from roots, the root-zone soil was air-dried and passed through a 2 mm sieve. Soil pH (H2O) was determined using a pH meter (F-22; HORIBA, Kyoto, Japan) with a water-to-soil ratio of 2.5:1 (v/w). The root-zone soil was digested using concentrated perchloric acid and nitric acid (HClO4-HNO3) at a ratio of 4:1 (v/v). Total concentrations of metals, including Al, iron (Fe), Cd, Cu, manganese (Mn), Pb, and Zn in the root-zone soil were measured using inductively coupled plasma optical emission spectroscopy (ICP-OES) (iICAP6300, Thermo Fisher Scientific, Waltham, USA).
The five individuals were carefully washed with running tap water, followed by Millipore water to remove soil particles. Each individual was separated into six parts: adventitious roots, dead root bark, rhizomes, live leaves, dead leaves, and flower spikes (flower spikes were present in three individuals due to growth differences). Each plant tissue was dried at 80°C for 48 h and ground into a powder. The dried plant powder was digested using concentrated HNO3 at 130°C. The metal concentrations (Al, Fe, Cd, Cu, Mn, Pb, and Zn) in each plant tissue were determined using ICP-OES.
2.2. Calculation of translocation and bioconcentration factors
The translocation factor (TF) and bioconcentration factor (BCF) are two parameters to evaluate plants’ abilities to transfer and accumulate heavy metals, respectively (Yoon et al. Citation2006; Zhou et al. Citation2013). The TF and BCF were calculated using the following formulas (Mendez and Maier Citation2008):
(1)
(1) where Mshoots was the metal concentration in the shoots, Madventitious roots was the metal concentration in the adventitious roots.
(2)
(2) where Msoil presented the metal concentration in the root-zone soil.
The results of the five individuals were averaged (three individuals for the flower spikes section) and standard errors (SEs) were calculated.
2.3. Analysis of phenolic compounds in adventitious roots of M. sinensis
Five individuals were used for phenolic analysis. Fresh adventitious roots were cut to pieces with scissors, placed in 100% methanol and extracted for 5 days in the dark. The methanol extract was filtered and evaporated in vacuo (REN-1000; Iwaki, Shizuoka, Japan). The concentrated sample was dissolved in 50% methanol and analyzed by high-performance liquid chromatography (HPLC; Prominence UFLC series, Shimadzu, Kyoto, Japan) using a Mightysil RP-18 GP column (75 × 4.6 mm i.d.; Kanto Chemical Co., Tokyo, Japan), and the spectral characteristics were analyzed using a diode array detector (DAD; SPD-M20A, Shimadzu, Kyoto, Japan). HPLC conditions were as described by Yamaji and Ichihara (Citation2012). According to HPLC-DAD analysis, the main phenolic compound in the adventitious roots extract was detected as chlorogenic acid according to the absorbance wavelengths at 218, 236, and 326 nm and the retention time when compared with standard chlorogenic acid (MP Biomedicals LLC., Santa Ana, CA, USA). The concentration of chlorogenic acid was calculated as 1.05 ± 0.28 mg/g fresh weight (FW; n = 5) based on the standard curve of chlorogenic acid analyzed at 320 nm using HPLC-DAD as described above.
2.4. Fungal infection observation, isolation of root endophytic fungi, and selection of functional endophytic fungi by Zn-solubilization test
2.4.1. Fungal infection observation
The adventitious roots were stained with trypan blue following the procedure of Doyama et al. (Citation2021). One hundred and fifty intersections of the stained roots were observed under a microscope (Olympus CX21; Olympus, Tokyo, Japan). Microsclerotia and endophytic hyphae (Read and Haselwandter Citation1981; Jumpponen and Trappe Citation1998) were observed. Paris-type, vesicles, and hyphae of arbuscular mycorrhizal (AM) fungi (Smith and Smith Citation1997) have also been observed. The infection rates were calculated as described by McGonigle et al. (Citation1990). The results of five individuals were averaged and the SEs were calculated.
2.4.2. Isolation of endophytic fungi and selection of functional root endophytic fungi by Zn-solubilization test
Endophytic fungi were isolated from the adventitious roots of M. sinensis. The well-washed roots by running tap water and Millipore water were sterilized with 70% ethanol for 1 min, 7.5% hydrogen peroxide for 5 min, and 70% ethanol for 1 min. The roots were washed twice with sterilized Millipore water to remove chemical reagents. The sterilized roots were cut into approximately 10 mm pieces and placed on 1% malt extract agar medium (1% MA). Fifty root segments were prepared per individual, and in total, 250 segments of five individuals were incubated at 23°C for a month. The colonies were observed microscopically and purified. The detection rates of root endophytic fungi were determined using the following formula (Haruma et al. Citation2018):
(3)
(3) where, Nfungi was the number of root segments in which the fungus was detected. Ntotal was the total number of root segments.
Zn was highly accumulated in the adventitious roots; therefore, for the selection of root endophytes, which would influence the Zn tolerance of M. sinensis chemically, the ability to detoxify Zn was evaluated. Three fungal genera, namely Phialocephala, Acephala, and Talaromyces, showing high detection rates were used in this study. The root endophytes cannot grow normally on Chrome azurol S with Zn agar media (CAS-Zn) (Doyama et al. Citation2022; modification of Alexander and Zuberer Citation1991), therefore, the Zn-solubilization test described by Martino et al. (Citation2003) was used. Fungi were grown on 1% MA amended with insoluble Zn compounds, ZnO (0.24 g/L) and Zn3(PO4)2 (0.42 g/L) (1% MA-Zn). Three isolates of each fungal genus were randomly selected, and fungal disks (5.5 mm i.d.) cut from the edge of the mycelia were placed on 1% MA-Zn. After 3 days of incubation at 23°C in the dark, the diameters of the mycelia and clear zones were measured, and the possible Zn-chelating activity was calculated by dividing the diameters of the clear zones by those of the mycelia, showing that 1.0 indicated high production. The results of three replicates were averaged and SEs were calculated. Among the fungal isolates, Talaromyces showed the highest production of possible Zn-chelating compounds. Therefore, the activities of 52 isolates of Talaromyces in total were also determined using 1% MA-Zn.
2.5. Identification of root endophytic fungi
Talaromyces isolate Ori5-2p, which showed the highest possible Zn-chelating activity on 1% MA-Zn, and Phialocephala isolate Ori2-15, which showed the highest detection rate and possible Zn-chelating activity, were identified by morphological characteristics and molecular analysis before use in the inoculation test. The primer pairs ITS5 and ITS4 were used to amplify ITS regions (White et al. Citation1990). The PCR was performed as following protocol: an initial denaturing step at 94°C for 4 min, 35 cycles at 94°C for 30 s, 52°C for 50 s, and 72°C for 50 s; and final elongation at 74°C for 6 min. The 20 µL PCR mixture incorporated 1 µL of DNA template, 10 µL of GoTaq master mix (Promega Co., Ltd, Madison, WI, USA), 0.5 µL of each primer (10 mM), and 8 µL of sterile distilled water. Amplicons were purified using the exoSAP-IT PCR Product Cleanup reagent (Applied BiosystemsTM) and used for sequencing with a BigDye Terminator Cycle Sequencing FS Ready Reaction Kit ver. 3.1, and analyzed via ABI3100 genetic analyzer (Applied Biosystems, Carlsbad, CA, USA). For fungal identification, the sequences were compared with those in public databases of the National Center for Biotechnology Information (NCBI) using the BLAST algorithm. Phialocephala isolate Ori2-15 and Talaromyces isolate Ori5-2p were identified as Phialocephala fortinii and Talaromyces verruculosus, respectively. The isolates were deposited in the National Institute of Technology and Evaluation and the author’s (XL) private culture collection: Phialocephala fortinii C. J. K. Wang & H. E. Wilcox, 1985 (NBRC115715) and Talaromyces verruculosus (Peyronel) Samson, Yilmaz, Frisvad & Seifert, 2011(NBRC115588).
2.6. Qualitative and quantitative analyses of Zn-chelating compound produced by Talaromyces verruculosus
Talaromyces verruculosus (Ori5-2p) was used in this test. Spore suspension (500 µL, 1 × 105 spores in 500 µL of sterilized Millipore water) was added into 100 mL of 1% malt extract liquid medium (1% ME) in a 300 mL Erlenmeyer flask. After 15-day incubation in the dark at 23°C under stationary condition, the fungal culture filtrate (850 mL) was extracted with ethyl acetate (EtOAc) (280 mL) three times. The EtOAc layer was washed with saturated NaCl and dried over Na2SO4. The water layer (W1) was evaporated in vacuo and the concentrated W1 (60 mL) was extracted three times with n-butanol (20 mL per time). The butanol layer was then evaporated in vacuo and dissolved in methanol (3 mL). After the butanol extraction, the water layer (W2) was concentrated in vacuo to be 15 mL.
The Zn-chelating activities of the culture filtrate, the EtOAc layer, W1, the butanol layer and W2, were examined using CAS-Zn (Doyama et al. Citation2022; modification of Alexander and Zuberer Citation1991). Culture filtrate, W1 or W2 (200 µL, equivalent to 800 µL of the culture filtrate) was sterilized by 0.22 μm syringe filter and aseptically injected into a sterile cylinder (6 mm i.d. × 10 mm, stainless steel) on CAS-Zn. For EtOAc and butanol layers (equivalent to 800 µL of the culture filtrate), the paper disk method (Yamaji et al. Citation1999) was used on CAS-Zn. After 36 h incubation at 23°C in the dark, the diameters of the clear zones were measured. As a control, 1% ME was extracted with EtOAc and n-butanol, as described above. The 1% ME, EtOAc layer, butanol layer, W1 and W2 of 1% ME (equivalent to 800 µL of 1% ME) were also examined on CAS-Zn. Three replicates were conducted for each sample of culture filtrate or 1% ME.
Zn-chelating activity was observed in W2 of the culture filtrate on CAS-Zn, and the Zn-chelating compound was isolated. Organic acids in W2 (100 µL, equivalent to 800 µL of the culture filtrate) were analyzed by means of gas chromatography/mass spectrometry (GC/MS) on a GC/MS-QP 2010 instrument equipped with a GC-2010 electro-ionization MS detector (Sinmadzu, Kyoto, Japan) after purification and trimethylsilylation following Yamaji et al. (Citation2016b). Gluconic acid was considered as a candidate Zn-chelating compound in W2; however, trimethylsilylated gluconic acid prepared by 50% D-gluconic acid solution (Wako, Osaka, Japan) showed three peaks, including two gluconolactones in equilibrium (Barbe et al. Citation2002). Therefore, the enzymatic D-gluconic acid/D-glucono-δ-lactone assay kit (K-GATE, Megazyme, Bray, Ireland) was used for quantification of gluconic acid in W2. The results of three replicates were averaged and SEs were calculated.
2.7. Inoculation test of root endophytic fungi to M. sinensis seedlings
2.7.1. Chemical properties in mine soil and sterilized mine soil
In November 2020, soil was collected from a mixed deciduous forest around the former mine, Ibaraki Prefecture, Japan, which has been reported to contain high concentrations of heavy metals, such as Cu, Pb, and Zn, compared with general forest soil (Kamiga and Tejiri Citation2003). After air-drying and sieving (< 2 mm), the fine mine soil was digested with HNO3 and HClO4; then, Al, Fe, Cd, Cu, Mn, Pb, and Zn were measured by ICP-OES. The cation exchange capacity (CEC) was determined according to the FAO method (FAO Citation2014). The carbon (C) and nitrogen (N) contents of the mine soil were determined using a CHNS elemental analyzer (UNICUBE; Elementar, Langenselbold, Germany). The measured soil chemical characteristics are shown in .
Table 1. Chemical properties of mine soil.
The mine soil used in the inoculation test was sterilized by intermittent irradiation of γ-ray (30-kGy). The pH (H2O) and the concentrations of exchangeable Al, Cd, Cu, Pb, Zn, calcium (Ca), potassium (K), magnesium (Mg) and sodium (Na) were measured to estimate the effect of γ-ray sterilization on the chemical properties of the soil. Exchangeable Al was extracted using 1 M KCl for 30 min. Exchangeable Cd, Cu, and Zn were extracted with 0.05 M of Ca(NO3)2 at 150 rpm for 1 h. Exchangeable Pb was extracted with CH3COONH4 (pH 4.5) at 150 rpm for 1 h. Exchangeable Ca, K, Mg and Na were extracted with 1 M CH3COONH4 (pH 7.0) at 150 rpm for 1 h. Exchangeable elemental concentrations were measured by ICP-OES. The measured soil chemical characteristics are shown in .
Table 2. Exchangeable elemental concentrations and soil pH (H2O) in non-sterilized and γ-ray-sterilized mine soils.
2.7.2. Root endophytic fungi used for the inoculation test
Talaromyces verruculosus (Ori5-2p), which produced Zn-chelating D-gluconic acid, and P. fortinii (Ori2-15), showed the highest detection rate and possible Zn-chelating activity, were used. Because root endophytes producing indole-3-acetic acid (IAA) are considered to improve defense against heavy metal stress in plants (Ma et al. Citation2011), the IAA production by these fungi was examined before the inoculation test, as described by Gordon and Weber (Citation1951). Three mycelial disks (5.5 mm i.d.) of T. verruculosus (Ori5-2p) or P. fortinii (Ori2-15) previously grown on 1% MA for 7 days were inoculated into 15 mL of sterilized Czapek broth in a 50 mL Erlenmeyer flask supplied with and without tryptophan (Waqas et al. Citation2012). After 15-day incubation statically at 23°C in the dark, IAA concentration in the culture filtrate was analyzed via measurement of absorbance at 530 nm with a UV-Vis spectrometer (UV-2450, Shimadzu, Kyoto, Japan). The results for three replicates were averaged and SEs were calculated.
2.7.3. Preparation of axenic seedlings and fungal suspensions
In October 2020, M. sinensis seeds were collected from the study site where M. sinensis were growing naturally. Following the procedure of Haruma et al. (Citation2018), axenic seedlings were prepared in the growth chamber (NK Systems LP-100S, Nippon Medical & Instruments Co., Osaka, Japan) for 35 days (day/night cycle: 14 h light at 25°C/10 h dark at 20°C). The photosynthetic photon flux density was determined as 107.1 ± 3.1 μmol photons/s/m2 (average ± SEs, n = 30) via an LI-1400 data logger equipped with a quantum sensor (Licor; Lincoln, NE, USA). Seedlings at the second leaf stage were used for the test. For the inoculation, T. verruculosus spore suspension (106 spores in 500 µL of sterilized Millipore water) and P. fortinii mycelial suspension [2.4 mg of mycelial dry weight (DW) in 500 µL of sterilized Millipore water] were prepared.
2.7.4. Inoculation test
On a clean bench, the sterilized mine soil (28 g) was transferred to a sterile culture tube (120 mm height × 30 mm internal diameter; AGC Techno Glass Co., Shizuoka, Japan) and covered with a polypropylene cap (AGC Techno Glass Co.) for sterilization. Fifteen mL of sterilized Millipore water was added. In each culture tube, one seedling was transplanted at the second leaf stage. Three treatments were set up: (1) seedlings with 500 µL of sterilized Millipore water as control; (2) seedlings with 500 µL of T. verruculosus spore suspension; and (3) seedlings with 500 µL of P. fortinii mycelial suspension. Six seedlings were prepared per replicate and four replicates were used for each treatment. In total, 72 seedlings (24 seedlings per treatment) were grown in a growth chamber for 40 days as described in Section 2.7.3.
2.7.5. Effects of root endophytic fungi on M. sinensis seedling growth, concentrations of elements, and phenolic production
After 40-day inoculation, 72 seedlings (24 seedlings per treatment) were confirmed to be alive or rotten by observing the color of the leaves and roots. Live seedlings were carefully washed with tap water, followed by Millipore water. The following growth parameters of all live seedlings (24 seedlings in the control, 22 seedlings inoculated with T. verruculosus, and 19 seedlings inoculated with P. fortinii) were measured in each treatment: seedling height, number of alive leaves, number of dead leaves, FW, DW of terrestrial parts, and FWs of roots. Additionally, one live seedling was randomly used to measure root length per replicate in each treatment (four seedlings in total were used per treatment). One live seedling for fungal re-isolation, one for fungal infection observation, one for phenolic analysis, and one for elemental analysis were used per replicate in each treatment (four replicates were prepared per treatment). The DW of the roots used for elemental analysis was measured.
To confirm the success of the inoculation test, fungal re-isolation and infection observations were conducted following the procedures described in Sections 2.4.2 and 2.4.1, respectively. For elemental analysis, the terrestrial parts and roots were separately digested with nitric acid, as described above, to measure the following elements: nutrients (Ca, K, Mg, Na, and P) and metals (Al, Fe, Zn, Cu, Pb, and Cd) by ICP-OES (Agilent 5100, Agilent Technologies, Santa Clara, CA, USA). For the elemental analysis of the dead leaves, only metals were measured. The roots were extracted with 100% methanol to analyze the phenolics using HPLC-DAD for identification and quantification, as described above. According to Haruma et al. (Citation2023), further identification was performed by means of liquid chromatograph-mass spectrometer (LCMS-2020; Shimadzu, Kyoto, Japan) equipping with an electrospray ionization source under following condition: column, Mightysil RP-18 MS (150 × 2.0 mm i.d.; Kanto Chemical Co., Tokyo, Japan); eluent, 0.1% formic acid in ultrapure water (solvent A) and 100% acetonitrile (solvent B); flow rate, 0.2 mL/min; injection volume, 10 µL; column temperature, 40°C. The following gradient was used for the eluent system: 0 min, 100% A; 0–30 min, 50% A and 50% B; 30–60 min, 100% B. LCMS analysis identified chlorogenic acid in the root extract owing to the detection of the [M-H]- ion at m/z 353 in the negative mode of MS condition (Lu et al. Citation2021). For each treatment, four replicates were averaged, and the SEs were calculated.
2.8. Statistical analysis
The results were analyzed using SPSS Statistics software (ver. 25.0, IBM, Armonk, NY, USA). Per each element, differences in TFs or BCFs among plant tissues were evaluated using a one-way analysis of variance (one-way ANOVA) with the non-parametric Scheffé post hoc test. Differences between live leaves/roots and dead leaves/roots TFs of Cd were evaluated using the Student’s t-test. The differences in concentrations of exchangeable elements (Al, Cu, Pb, Zn, Ca, K, Mg and Na), and pH (H2O) values between non-sterilized and γ-ray-sterilized mine soils were evaluated by Student’s t-test. For the inoculation test, differences in growth variables, elemental concentrations (nutrients: Ca, K, Mg, Na, and P; metals: Al, Fe, Zn, Cu, Pb, and Cd), and chlorogenic acid concentrations were evaluated between treatments using one-way ANOVA with the non-parametric Scheffé post hoc test. Differences were considered statistically significant at p < 0.05.
3. Results
3.1. The pH (H2O) of root-zone soil, calculation of TFs and BCFs, and phenolic compounds in roots of M. sinensis
The pH (H2O) of root-zone soil was 5.94 ± 0.02, showing a weakly acidic soil environment for M. sinensis. The TF and BCF results are shown in and , respectively. For TF results, the dead leaves/adventitious roots TFs of Al, Fe, Cu, and Pb, except Zn and Mn, were significantly higher than live leaves/adventitious roots TFs and flower spikes/adventitious roots TFs (p < 0.05). Moreover, the dead leaves/adventitious roots TF of Fe, and the live leaves/roots, dead leaves/roots, and flower spikes/roots TFs of Mn were more than 1. With respect to BCFs, except Fe and Mn, the elemental BCFs of root bark were significantly higher than other plant tissues. For Mn, root bark BCF was significantly higher than rhizomes BCF (p < 0.05); however, there were no significant differences among other plant tissues BCFs. For Fe, there were no significant differences among plant tissues BCFs. For Cd, the root bark BCF was significantly higher than rhizomes, live leaves and dead leaves BCFs (p < 0.05). The root bark BCFs of Zn, Cu, Pb, and Cd were greater than 1. Additionally, the adventitious roots BCF of Cd was higher than 1.
Table 3. Translocation factors (TFs) of metals in M. sinensis.
Table 4. Bioconcentration factors (BCFs) of metals in M. sinensis.
Based on the HPLC-DAD, main phenolic compound of root extract was detected as chlorogenic acid, and the concentration was 1.05 ± 0.28 mg/g FW (n = 5).
3.2. Fungal infection observation
Observations of fungal infection that AM and endophytic fungi infected the adventitious roots of M. sinensis (). The microsclerotia of endophytic infected characteristics ((a)) and Paris-type morphology of AM fungi ((b)) were confirmed in trypan-blue-stained roots. shows the infection rates of root-endophytic fungi (hyphae and microsclerotia) and AM fungi (hyphae, Paris-type, and vesicle).
Figure 1. Morphological characteristics of endophytic and arbuscular mycorrhizal (AM) fungi in M. sinensis trypan-blue stained adventitious roots. (a) Microsclerotia and endophytic hyphae of dark septate endophytes; (b) Paris-type structure of AM fungi.
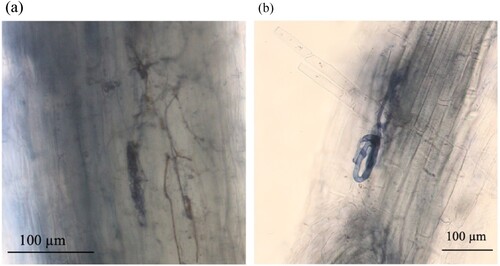
Table 5. Infection rates of root endophytic fungi and arbuscular mycorrhizal (AM) fungi.
3.3. Isolation and selection of functional root endophytic fungi by Zn-solubilization test
The three most frequently isolated genera of root endophytes were Phialocephala, Acephala, and Talaromyces, with detection rates of 25.6%, 13.2% and 9.6%, respectively. The Zn solubilization of these fungal genera (three isolates per genus) was determined using 1% MA-Zn, and Talaromyces isolates showed the highest possible Zn-chelating activities (). Thus, all isolates of Talaromyces were carefully checked via Zn-solubilization test, and the isolate Ori5-2p showed the highest possible Zn-chelating activity on 1% MA-Zn (Supplementary Figure 1). DNA analysis identified Ori5-2p as T. verruculosus.
Table 6. Zn-solubilization test of root endophytic fungi
3.4. Qualitative and quantitative analyses of Zn-chelating compounds produced by Talaromyces verruculosus
On 1% MA-Zn, a clear zone indicated that the insoluble Zn salt was dissolved by Zn-chelating compounds or proton release produced by fungi (Martino et al. Citation2003). Therefore, CAS-Zn was used to confirm the presence of the Zn-chelating compounds in the culture filtrate. Preliminary experiments showed that the unconcentrated culture filtrate did not exhibit any activity; therefore, the concentrated culture filtrate was used for the test. The concentrated culture filtrate of T. verruculosus (Ori5-2p) equivalent to 800 µL culture filtrate showed Zn-chelating activity on CAS-Zn (). After the fractionation of the culture filtrate, Zn-chelating activities in W1 and W2 equivalent to 800 µL culture filtrate were found while none was found in EtOAc and butanol layers (). As control, 1% ME, EtOAc layer, butanol layer, W1 and W2 (equivalent to 800 µL of 1% ME) did not show any activities. Therefore, we considered that the Zn-chelating compounds would move to W2 of the culture filtrate. According to the results of the GC/MS analysis, trimethylsilylated D-gluconic acid (three peaks, including two gluconolactones in equilibrium) in W2 was identified based on retention times and mass spectra when compared with standard trimethylsilylated D-gluconic acid. Based on the result of enzymatic assay kit, the concentration of gluconic acid was 42.4 ± 4.07 µg/mL volume of culture filtrate.
Table 7. Zn-chelating activities of compounds produced by Talaromyces verruculosus.
3.5. Inoculation test of T. verruculosus and P. fortinii to M. sinensis seedlings
Based on the results of fungal re-isolation, the inoculation test was considered to have been accomplished without contamination because no microorganisms were isolated from the seedling roots in the control; T. verruculosus and P. fortinii were re-isolated from the fungal-inoculated seedling roots. Moreover, microsclerotia and/or endophytic hyphae were observed in trypan blue-stained roots inoculated with T. verruculosus or P. fortinii. In trypan blue-stained roots inoculated with T. verruculosus, although microsclerotia were not observed, the endophytic hyphae were observed; the infection rate of endophytic hyphae was 75.3 ± 5.6%. In the trypan blue-stained roots of P. fortinii-inoculated seedlings, microsclerotia and endophytic hyphae were confirmed, and the infection rates were 52.8 ± 4.0% for microsclerotia and 91.5 ± 2.3% for endophytic hyphae.
After the inoculation test, all 24 seedlings in the control were alive; 22 seedlings inoculated with T. verruculosus and 19 seedlings inoculated with P. fortinii were alive. The live seedlings in the control and fungal treatments did not show symptoms of metal toxicity, such as browning of roots or chlorosis of leaves (). T. verruculosus inoculation significantly increased seedling height, the number of alive leaves, FW, and DW of terrestrial parts (p < 0.05), and significantly decreased the number of dead leaves compared with those of control seedlings (p < 0.05; ). P. fortinii significantly increased seedling height (p < 0.05); however, the number of live leaves was significantly decreased (p < 0.05), and the number of dead leaves was significantly increased compared with that of the control seedlings (p < 0.05; ). There were no significantly differences in FW and DW of terrestrial parts between control and P. fortinii-inoculated seedlings (p > 0.05). For the roots, there were no significant differences on the length, FW and DW among three treatments. Additionally, IAA productions of T. verruculosus and P. fortinii were 5.92 ± 0.50 and 4.04 ± 0.83 µg/mL (n = 3), respectively.
Figure 2. Miscanthus sinensis seedlings after inoculation test. (a) Seedlings in control, (b) seedlings inoculated with T. verruculosus, and (c) seedlings inoculated with P. fortinii. The white bar represents 1 cm.
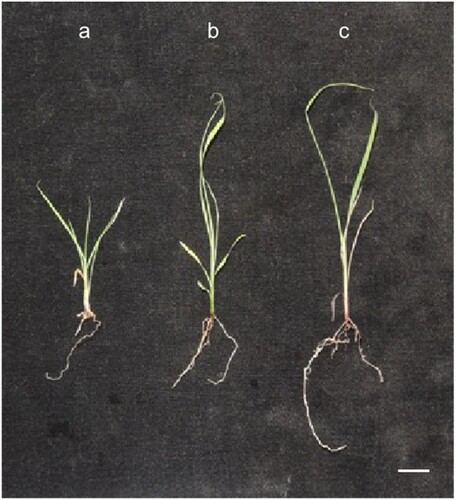
Table 8. The growth parameters of seedlings in each treatment after the inoculation test.
Nutrient elements (Ca, K, Mg, Na, and P) and metals (Al, Fe, Zn, Cu, and Pb) in the terrestrial parts and roots are shown in and , respectively. Cd concentrations of each plant tissue among treatments were not shown in because of detection limit. Inoculation with T. verruculosus and P. fortinii significantly increased K uptake in the terrestrial parts of seedlings ((a)); there were no significant differences in Ca, Mg, Na, and P among treatments ((a)). Moreover, there were no significant differences in all nutrient concentrations of the roots among treatments (p > 0.05, (b)). Additionally, there were no significant differences in metal concentrations, including Al, Fe, Zn, Cu, and Pb, of terrestrial parts ((a)), roots ((b)), and dead leaves ((c)) among treatments (p > 0.05). Seedlings in the control and fungal inoculation treatments contained higher concentrations of metals, especially Al and Fe, in the roots than in the terrestrial parts ((a,b)).
Figure 3. Concentrations of nutrient elements in M. sinensis seedlings after the inoculation test. (a) Concentrations in terrestrial parts, and (b) concentrations in roots. Nutrient concentrations of dead leaves were not examined. The results are expressed as means ± SEs (g/kg dry weight, n = 4). Different letters indicate statistically significant differences in each element between treatments by one-way ANOVA (Scheffé, p < 0.05).
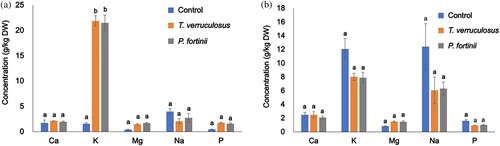
Figure 4. Concentrations of metals in M. sinensis seedlings after the inoculation test. (a) Concentrations in terrestrial parts, (b) concentrations in roots, and (c) concentrations in dead leaves. N.D. indicates the data were under the detection limit. The results are expressed as means ± SEs (g/kg, dry weight, n = 4). One-way ANOVA (Scheffé, p < 0.05). Cd concentrations of each plant tissue were not shown because of detection limit.
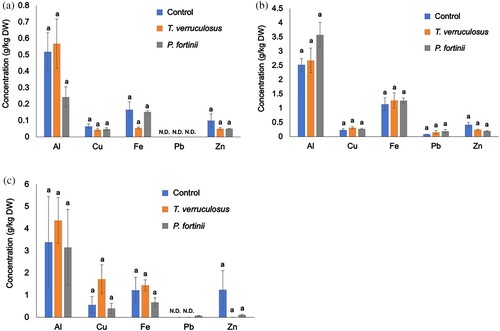
Chlorogenic acid concentrations in control seedlings, T. verruculosus-inoculated seedlings and P. fortinii-inoculated seedlings were 0.12 ± 0.03, 0.03 ± 0.01, and 0.02 ± 0.01 µg/mg FW (n = 4), respectively. The concentrations of chlorogenic acid were significantly lower in seedlings inoculated with T. verruculosus and P. fortinii than in the control seedlings (one-way ANOVA, Scheffé, p < 0.05).
4. Discussion
4.1. Metal accumulation and translocation in M. sinensis
TFs and BCFs are two important parameters to evaluate the accumulation and translocation capabilities of plants (Yoon et al. Citation2006; Zhou et al. Citation2013). In the present study, except Mn, the metals, including Al, Cu, Fe, Zn, and Pb, of live leaves/adventitious roots TFs and flower spikes/adventitious roots TFs were lower than 1 (), indicating that M. sinensis accumulated metals in its adventitious roots. The high Mn TFs of M. sinensis (above 1, ) indicated that Mn was highly transferred into aboveground part, which can be attributed to the nutrient requirement for growth because Mn is a macronutrient element. Moreover, Mn concentration in each tissue of M. sinensis was within the range of nutrient acquisition (Mn, 30–50 mg/kg, DW) (Larcher Citation2003), without showing toxicity. Moreover, the root bark BCFs of Cu, Zn, Pb and Cd were higher than 1, especially, the root bark BCF of Cd was more than 2 (), suggesting that M. sinensis would uptake heavy metals from the soil and immobilizes heavy metals in the roots. Moreover, in our inoculation test, the concentrations of Al (above 2 g/kg, (b)) and Fe (above 1 g/kg, (b)) in the roots of M. sinensis were higher compared with the acceptable concentration range in grass plants (Al, about 0.2 g/kg; Hutchinson Citation1945; Fe, less than 0.23 g/kg; Kabata-Pendias Citation2011), indicating that the roots of M. sinensis would be tolerant to high accumulations of these elements. The same phenomenon was observed in previous studies. Ezaki et al. (Citation2008) reported that M. sinensis accumulated Al, Cr, and Zn in its roots during hydroponic solution cultivation. Moreover, in metal-added hydroponic experiments, M. sinensis has been reported to contain high concentrations of Sb (Xue et al. Citation2015) and Hg (Zhao et al. Citation2019) in its roots, indicating metal tolerance in M. sinensis. Furthermore, M. sinensis, which grows at mine sites, showed a similar phenomenon of heavy metal/metalloid accumulation in the roots (Xue et al. Citation2014; Haruma et al. Citation2018; Sun et al. Citation2021).
4.2. Heavy metal tolerance in M. sinensis
Our results also showed that M. sinensis, which grew naturally at the study site, accumulated higher concentrations of Cu, Zn and Pb, in the dead root bark than other plant tissues (p < 0.05, ) and contained higher concentrations of Fe in dead leaves than live leaves and flower spikes (p < 0.05, ), indicating that M. sinensis might remove heavy metals from its living parts into dead parts to reduce heavy metal toxicity. Haruma et al. (Citation2023) also found that the Fe accumulation in dead root bark of M. sinensis, which grows naturally at a sedimentary site in a mine site, and it would be Fe exclusion mechanism of M. sinensis not to accumulate only in the living adventitious roots. According to Larcher (Citation2003), deposited mineral substances were removed through getting rid of different plant parts, such as old leaves, flower debris, and mature fruits, and that the continual shedding and renewal of leaves and bark are essential mechanisms for eliminating accumulated minerals in perennial plants. In the study of Dahmani-Muller et al. (Citation2000), Armeria maritima (Mill.) Willd. ssp. helleri (Wallr.) Löve, which was growing near a former metal smelter, contained higher Zn, Cd, Pb and Cu concentrations in old leaves than in young leaves, as a metal removal strategy. Therefore, M. sinensis might tolerates heavy metals, possibly through removing metals by the dead parts.
Production of secondary metabolites is a general defense against environmental stress in plants (Ashraf et al. Citation2018; Yang et al. Citation2018). Our results showed that M. sinensis has potential heavy metal tolerance in the living adventitious roots via the production of detoxicants, mainly chlorogenic acid. Chlorogenic acid, a well-known phenolic acid found in coffee and tea (Meng et al. Citation2013; Naveed et al. Citation2018), is the secondary metabolite in plants to against several abiotic stresses, including heavy metal (Soviguidi et al. Citation2022). With respect to metal detoxification of chlorogenic acid, the possibly contribution might be (1) chelating heavy metals, such as Al, Cu, Mn, Fe, and Zn (Améziane et al. Citation1996; Adams et al. Citation2002; Andjelković et al. Citation2006), forming complexes that are less toxic to plants compared to free metal ions (Wasay et al. Citation1998; Jung et al. Citation2003; Xu et al. Citation2012), and/or (2) acting as an antioxidant, capturing reactive oxygen radicals generated under heavy metal stress (Gill and Tuteja Citation2010). Miscanthus species, such as M. sinensis, M. sacchariflorus (Maxim.) Franch., and M. × giganteus J. M. Greef & Deuter ex Hodk. & Renvoize, which were growing in non-polluted soil, showed chlorogenic acid production (Parveen et al. Citation2013). Parveen et al. (Citation2013) clarified that M. sinensis produced chlorogenic acid in leaves, stems and flowers, and the concentrations were 0.788 mg/g FW, 0.204 mg/g FW, 0.395 mg/g FW, respectively. In contrast, in the present study, chlorogenic acid concentration in adventitious roots of M. sinensis was 1.05 ± 0.28 mg/g FW, which was much higher than concentrations shown in Parveen et al. (Citation2013), and the content was also approximately 10 times higher than that reported in a previous study of M. sinensis growing at a mine site (0.13 mg/g FW; Haruma et al. Citation2018). The increased chlorogenic acid production might be stimulated by the heavy metal stress and would contribute to heavy metal tolerance in M. sinensis growing at the study site. Other studies agree with our results. For example, Dresler et al. (Citation2017) reported that the production of chlorogenic acid by Echium vulgare L. increased significantly with increasing Zn and Pb concentrations under hydroponic conditions. An increase in chlorogenic acid has also been observed in Zea mays L. exposed to elevated Pb concentrations (Kısa et al. Citation2016) and in Gynura pseudochina (L.) DC. under elevated Zn stress (Mongkhonsin et al. Citation2016). Additionally, Mongkhonsin et al. (Citation2016) reported an increase in the concentration of phenolic compounds, including chlorogenic acid. Therefore, the relatively high chlorogenic acid production in M. sinensis indicated high heavy metal stress at the study site.
Generally, plants exhibit various responses to heavy metal stress. The production of chemicals, such as phenolic compounds and organic acids, and the reduction of heavy metals entering cells by binding metals within the cell walls are two important defenses against heavy metals in plants (Larcher Citation2003). Haruma et al. (Citation2018) found that Al, which bind to root cell walls, may be involved in Al detoxification in M. sinensis. To confirm the role of root cell walls as a barrier for heavy metals in M. sinensis, heavy metal localization in living roots should be considered in future investigation.
4.3. Root endophytes enhanced growth in M. sinensis
In the present study, P. fortinii and T. verruculosus significantly increased the seedling height and FW of alive leaves of M. sinensis (p > 0.05, ), suggesting that both endophytic fungi can enhance host plant growth under heavy metal stress. One factor in growth enhancement was IAA production by P. fortinii or T. verruculosus as shown in Section 3.5. The IAA produced by endophytic fungi has been found to increase host plant growth (Waqas et al. Citation2012; Mehmood et al. Citation2019). Under heavy metal stress, the endophytic fungi C. cupureum and P. fortinii enhanced M. sinensis growth by inducing IAA production (Haruma et al. Citation2018; Citation2021). Additionally, our results indicated that P. fortinii and T. verruculosus inoculation increased K concentrations in the shoots of M. sinensis ((a)); this increase in K uptake would also contribute to growth enhancement. K is a necessary nutrient for several plant growth processes, such as photosynthesis and metabolism (Prajapati and Modi Citation2012). Yamaji et al. (Citation2016a) also showed that P. fortinii increased K concentration in the shoots of the host plant, Clethra barbinervis Sieb. et Zucc. Therefore, P. fortinii and T. verruculosus enhanced the seedling growth of M. sinensis possibly via IAA production and nutrient K uptake promotion.
4.4. The effect of root endophytes on heavy metal tolerance in M. sinensis
Root endophytes enhanced heavy metal tolerance in host plants. Root endophytes can produce several types of compounds that enhance host plant tolerance to environmental stresses. In our study, T. verruculosus produced gluconic acid with high Zn chelation ability via the CAS-Zn assay, indicating a high potential for Zn detoxification in M. sinensis. Talaromyces, which is used to belong to Penicillium and a teleomorphic state of Penicillium, is ubiquitous in the natural environment (Heo et al. Citation2019). Several species in Talaromyces are able to produce various bioactive compounds (Suzuki et al. Citation2000; Wang et al. Citation2019). Talaromyces verruculosus, earlier known as ‘Penicillium verruculosum’ (Samson et al. Citation2011), has also been found to produce several kinds of compounds, such as pigments (Chadni et al. Citation2017), antimicrobial compounds (Miao et al. Citation2012), and oligophenalenone dimers, which show high inhibition to tumor cells (Wang et al. Citation2019). However, to the best of our knowledge, organic acid production in T. verruculosus has not yet been reported. Gluconic acid is a natural product of fungal fermentation and a highly effective chelating agent widely used for metal removal, such as Cu, Pb, and Zn, from soil (Fischer and Bipp Citation2002) and metal-cleaning operations (Anastassiadis and Morgunov Citation2007). Gluconic acid can chelate several other heavy metals such as Al, Cu, Cd, Fe, and Pb (Sawyer Citation1964; Kołodyńska Citation2011), suggesting that organic acid-metal complexes are less toxic than free metal ions (Wasay et al. Citation1998). Haruma et al. (Citation2019) reported that the root endophytic C. cupureum, which produced the Al-chelating compound oosporein, enhanced Al tolerance in M. sinensis. Therefore, endophytic T. verruculosus would enhance the heavy metal tolerance of M. sinensis via gluconic acid production. In contrast, our results indicated that P. fortinii did not produce a Zn-chelating compound, as shown in . Phialocephala fortinii, a well-known DSE, can produce black melanin, which has been found to chelate heavy metals, such as Cu, Fe, and Zn (Zunino and Martin Citation1977; Gadd Citation1993), indicating possible detoxification. In our experiment, the melanin produced by P. fortinii might have influenced the heavy metal tolerance of M. sinensis, but further studies are needed.
Additionally, root endophytes have been generally known to stimulate host plants to produce secondary metabolites (Schulz et al. Citation1999; Citation2002). Schulz et al. (Citation1999) found that Cryptosporiopsis sp. increased soluble proanthocyanidin production in the roots of Larix decidua Mill. and Fusarium sp. enhanced ferulic acid production in the roots of barley (Hordeum vulgare L.). Haruma et al. (Citation2019) reported that root-endophytic C. cupureum enhanced chlorogenic acid production in M. sinensis under hydroponic condition containing high concentration of Al. However, in the present study, P. fortinii and T. verruculosus decreased the chlorogenic acid production in M. sinensis seedlings under heavy metal stress. Similarly, in a study by Haruma et al. (Citation2021), P. fortinii inoculation decreased the concentration of chlorogenic acid in the roots of M. sinensis grown in mine soil compared to the control without fungal inoculation. The differences in their influence on secondary metabolite production might depend on the characteristics of the root endophytes, weak pathogens, or saprophytic fungi. P. fortinii and T. verruculosus have been reported as patent saprophytic fungi (Wigney et al. Citation1990; Malicka et al. Citation2022), which play an important role in the decomposition of plant litter (Kubartová et al. Citation2009), but there is no research showing the induction of secondary metabolite production in plants by these fungi. Therefore, our results suggest that P. fortinii and T. verruculosus, which are saprophytic fungi, did not increase the secondary metabolite chlorogenic acid in M. sinensis, indicating that P. fortinii and T. verruculosus did not directly enhance the chemical defense of M. sinensis in the heavy metal tolerance.
4.5. Potential of using M. sinensis and root endophytes at mining sites
Miscanthus sinensis, a pioneer species, naturally grows in several mining sites and has been extensively studied (Hiroi Citation1974, Citation1980; Zhao et al. Citation2013; Babu et al. Citation2015). The habitat is not limited to the mining site; M. sinensis can be also found in other disturbed environmental areas, such as ski slopes (Tsuyuzaki Citation1995), volcanic areas, and coastal regions with acid sulfate soils (An et al. Citation2008), indicating the adaptation to various environmental stresses. This adaptability contributes to specific characteristics of M. sinensis. Ohtsuka et al. (Citation1993) proposed that M. sinensis promoted roots deeply in order to utilize water and nutrient efficiently; therefore, M. sinensis could tolerate severe drought or low nutrient condition. The less sensitive to water stress of M. sinensis also been pointed out by Clifton-Brown et al. (Citation2002) and da Costa et al. (Citation2019). Furthermore, M. sinensis has been observed to facilitate vegetation succession. In the study of Haruma et al. (Citation2023), at a sedimentary site in a mine site, M. sinensis formed patches and promoted the establishment of tree seedlings of Pinus densiflora Sieb. et Zucc., resulting in vegetation succession. Therefore, M. sinensis would be a promising candidate for enhancing the vegetation at mining sites. Moreover, root endophytes P. fortinii and T. verruculosus enhanced growth of M. sinensis and affected heavy metal tolerance on the plant via inoculation tests; therefore, the growth enhancement facilitated by root endophytes would further accelerate vegetation development. Future research should be conducted to investigate the potential contributions of P. fortinii and/or T. verruculosus whether these fungi can facilitate the vegetation at mining sites or not.
5. Conclusion
We found that M. sinensis, which grew naturally at a mine site, accumulated high concentrations of heavy metals in the adventitious roots and tolerated heavy metals by removing them from dead parts and producing chlorogenic acid for detoxification. In inoculation tests, the root endophytes P. fortinii and T. verruculosus enhanced M. sinensis seedling growth under heavy metal stress by promoting nutrient K uptake and possible IAA production. Moreover, T. verruculosus increased the heavy metal tolerance of M. sinensis by producing gluconic acid. Further investigations are needed to identify whether P. fortinii produces melanin and examine the role of melanin in heavy metal detoxification and to clarify whether root endophytes contribute to the growth enhancement and detoxification of heavy metals in M. sinensis under the field condition. We hope that our study provides fundamental knowledge regarding the use of heavy metal-tolerating M. sinensis associated with root endophytes for revegetation at mining sites.
Supplemental Material
Download MS Word (58.4 KB)Acknowledgements
We are grateful for the support provided by the Japan Mining Industry Association. Conception and design, XL, KY and TH; investigation, XL, KY, TH, KD and HM; writing – original draft preparation, XL and KY; writing – review and editing, XL, KY, TH, KD and HM. All authors approved the final version of the manuscript for submission.
Disclosure statement
No potential conflict of interest was reported by the author(s).
Data availability statement
The data of heavy metals concentration of plant tissues is not available due to the commercial restriction.
Additional information
Funding
Notes on contributors
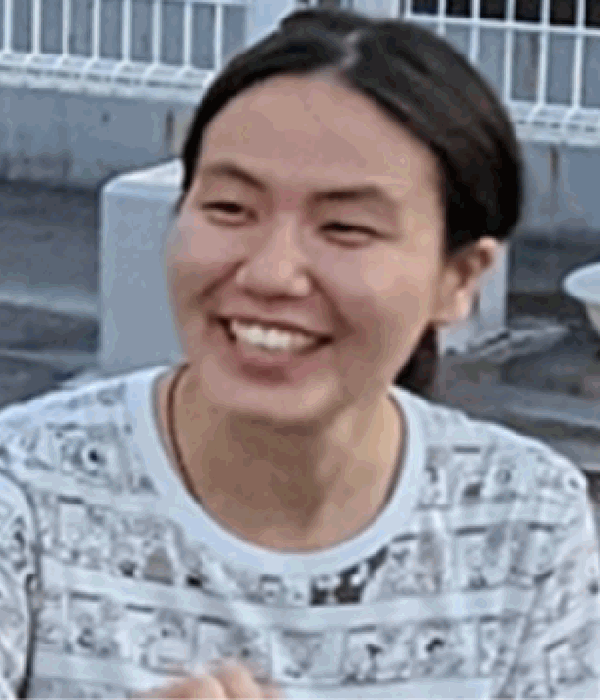
Xingyan Lu
Xingyan Lu is a postdoctoral researcher in Life and Environmental Sciences at University of Tsukuba. She obtained her Ph.D. in Environmental Studies from University of Tsukuba. Her researches are mainly related to microbiology, plant physiology and environmental sciences, especially on heavy metal tolerance of plants and the effects of endophytes on host plants.
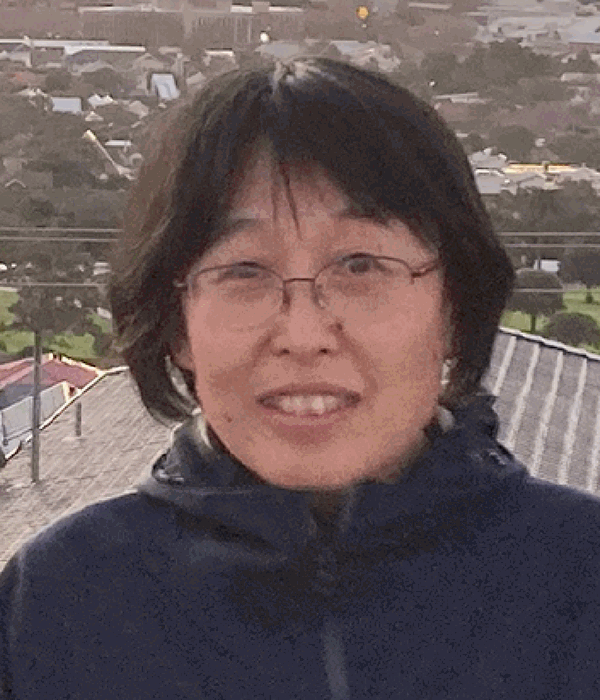
Keiko Yamaji
Keiko Yamaji is a professor in Life and Environmental Sciences, University of Tsukuba. She has received Ph.D. in Applied Natural Product Chemistry. She has been studying plant-microbe symbiosis under environmental stresses on Environmental Ecological Chemistry, which is related to field works as well as lab works including chemistry and microbiology.
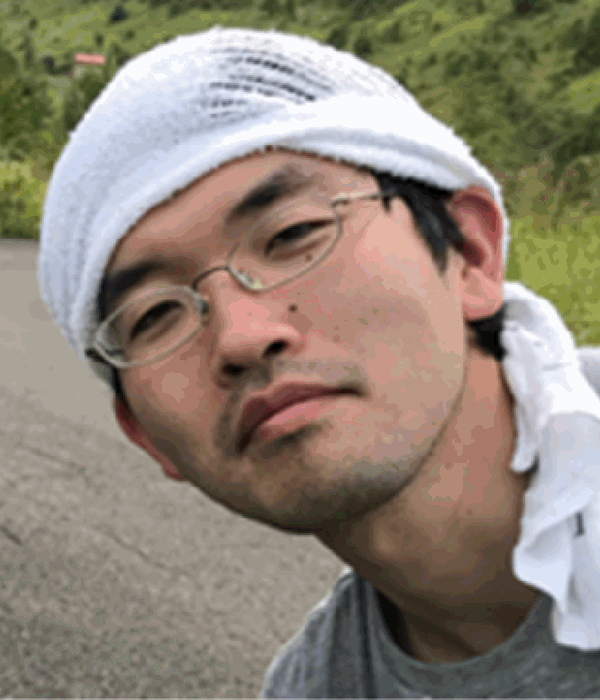
Toshikatsu Haruma
Toshikatsu Haruma is a researcher in Department of Mushroom and Forest Microbiology, Forestry and Forest Products Research Institute in Japan. His research is conducted in order to clarify interaction between plants and microbes growing at severe environmental stress including harmful metals and acid soil from the microbiological and chemical points of view.
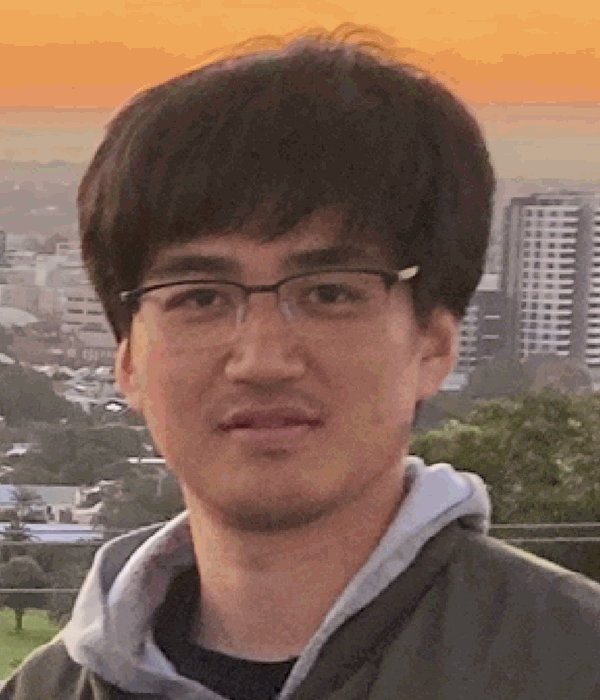
Kohei Doyama
Kohei Doyama is a post-doctoral fellow in Research Institute for Geo-Resources and Environment, National Institute of Advanced Industrial and Science Technology. He is engaged in management of legacy mines, where rehabilitation and reclamation has been required. His major is environmental sciences, especially plant-microbial interactions in the stress soils.
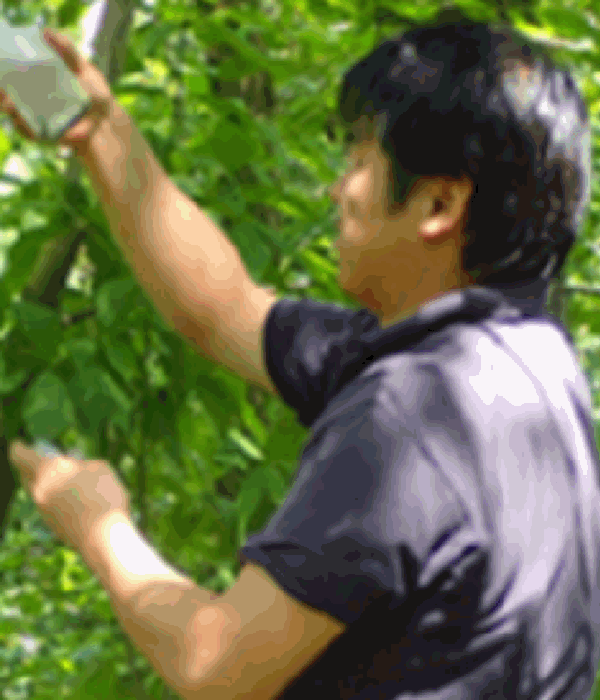
Hayato Masuya
Hayato Masuya is a chief scientist in Department of Mushroom and Forest Microbiology, Forestry and Forest Products Research Institute in Japan. His research experiences and interests are on the mycology and forest pathology, but also diversity and ecology of root endophytic fungi, particularly in the heavy metal contaminated lands.
References
- Adams ML, O'Sullivan B, Downard AJ, Powell KJ. 2002. Stability constants for aluminum (III) complexes with the 1, 2-dihydroxyaryl ligands caffeic acid, chlorogenic acid, DHB, and DASA in aqueous solution. J Chem Eng Data. 47:289–296. doi:10.1021/je010279e.
- Alexander DB, Zuberer DA. 1991. Use of chrome azurol S reagents to evaluate siderophore production by rhizosphere bacteria. Biol Fertil Soils. 12:39–45. doi:10.1007/BF00369386.
- Aly AH, Debbab A, Proksch P. 2011. Fungal endophytes: unique plant inhabitants with great promises. Appl Microbiol Biotechnol. 90:1829–1845. doi:10.1007/s00253-011-3270-y.
- Améziane J, Aplincourt M, Dupont L, Heirman F, Pierrard JC. 1996. Thermodynamic stability of copper (II), manganese (II), zinc (II) and iron (III) complexes with chlorogenic acid. Bull Soc Chim Fr. 133:243–249.
- An GH, Miyakawa S, Kawahara A, Osaki M, Ezawa T. 2008. Community structure of arbuscular mycorrhizal fungi associated with pioneer grass species Miscanthus sinensis in acid sulfate soils: habitat segregation along pH gradients. Soil Sci Plant Nutr. 54:517–528. doi:10.1111/j.1747-0765.2008.00267.x.
- Anastassiadis S, Morgunov IG. 2007. Gluconic acid production. Recent Pat Biotechnol. 1:167–180. doi:10.2174/187220807780809472.
- Andjelković M, Van Camp J, De Meulenaer B, Depaemelaere G, Socaciu C, Verloo M, Verhe R. 2006. Iron-chelation properties of phenolic acids bearing catechol and galloyl groups. Food Chem. 98:23–31. doi:10.1016/j.foodchem.2005.05.044.
- Arif N, Yadav V, Singh S, Singh S, Ahmad P, Mishra RK, Sharma S, Tripathi DK, Dubey NK, Chauhan DK. 2016. Influence of high and low levels of plant-beneficial heavy metal ions on plant growth and development. Front Environ Sci. 4:69. doi:10.3389/fenvs.2016.00069.
- Ashraf MA, Iqbal M, Rasheed R, Hussain I, Riaz M, Arif MS. 2018. Environmental stress and secondary metabolites in plants: an overview. In: Ahmad P, Ahanger MA, Singh VP, Tripathi DK, Alam P, Alyemeni MN, editor. Plant metabolites and regulation under environmental stress. Cambridge: Academic Press; p. 153–167.
- Babu AG, Shea PJ, Sudhakar D, Jung IB, Oh BT. 2015. Potential use of Pseudomonas koreensis AGB-1 in association with Miscanthus sinensis to remediate heavy metal (loid)-contaminated mining site soil. J Environ Manage. 151:160–166. doi:10.1016/j.jenvman.2014.12.045.
- Baker AJM. 1981. Accumulators and excluders-strategies in the response of plants to heavy metals. J Plant Nutr. 3:643–654. doi:10.1080/01904168109362867.
- Barbe JC, de Revel G, Bertrand A. 2002. Gluconic acid, its lactones, and SO2 binding phenomena in musts from botrytized grapes. J Agric Food Chem. 50:6408–6412. doi:10.1021/jf020412m.
- Chadni Z, Rahaman MH, Jerin I, Hoque KMF, Reza MA. 2017. Extraction and optimisation of red pigment production as secondary metabolites from Talaromyces verruculosus and its potential use in textile industries. Mycology. 8:48–57. doi:10.1080/21501203.2017.1302013.
- Clifton-Brown JC, Lewandowski I, Bangerth F, jones MB. 2002. Comparative responses to water stress in stay-green, rapid- and slow senescing genotypes of the biomass crop, Miscanthus. New Phytol. 154:335–345. doi:10.1046/j.1469-8137.2002.00381.x.
- da Costa RMF, Simister R, Roberts LA, Timms-Taravella E, Cambler AB, Corke FMK, Han J, Ward RJ, Buskeridge MS, Gomez LD, Bosch M. 2019. Nutrient and drought stress: implications for phenology and biomass quality in Miscanthus. Ann Bot. 124:553–566. doi:10.1093/aob/mcy155.
- Dahmani-Muller H, Van Oort F, Gélie B, Balabane M. 2000. Strategies of heavy metal uptake by three plant species growing near a metal smelter. Environ Pollut. 109:231–238. doi:10.1016/S0269-7491(99)00262-6.
- Deng Z, Cao L. 2017. Fungal endophytes and their interactions with plants in phytoremediation: a review. Chemosphere. 168:1100–1106. doi:10.1016/j.chemosphere.2016.10.097.
- Doyama K, Haruma T, Hishiyama S, Kato A, Masuya H, Yamaji K. 2022. Isoavenaciol and 7-hydroxyisoavenaciol: Zn-chelating metallophores produced by root-endophytic Pezicula ericae in a Zn accumulating plant, Aucuba japonica. Phytochemistry. 206:113547. doi:10.1016/j.phytochem.2022.113547.
- Doyama K, Yamaji K, Haruma T, Ishida A, Mori S, Kurosawa Y. 2021. Zn tolerance in the evergreen shrub, Aucuba japonica, naturally growing at a mine site: cell wall immobilization, aucubin production, and Zn adsorption on fungal mycelia. PLoS One. 16:e0257690. doi:10.1371/journal.pone.0257690.
- Dresler S, Wójciak-Kosior M, Sowa I, Stanisławski G, Bany I, Wójcik M. 2017. Effect of short-term Zn/Pb or long-term multi-metal stress on physiological and morphological parameters of metallicolous and nonmetallicolous Echium vulgare L. populations. Plant Physiol Biochem. 115:380–389. doi:10.1016/j.plaphy.2017.04.016.
- Ernst WHO, Verkleij JAC, Schat H. 1992. Metal tolerance in plants. Acta Bot Neerl. 41:229–248. doi:10.1111/j.1438-8677.1992.tb01332.x.
- Ezaki B, Nagao E, Yamamoto Y, Nakashima S, Enomoto T. 2008. Wild plants, Andropogon virginicus L. and Miscanthus sinensis Anders, are tolerant to multiple stresses including aluminum, heavy metals and oxidative stresses. Plant Cell Rep. 27:951–961. doi:10.1007/s00299-007-0503-8.
- FAO. 2014. World reference base for soil resources 2014: international soil classification system for naming soils and creating legends for soil maps. Rome: Food and Agriculture Organization of the United Nations.
- Fischer K, Bipp HP. 2002. Removal of heavy metals from soil components and soils by natural chelating agents. Part II. Soil extraction by sugar acids. Water Air Soil Pollut. 138:271–288. doi:10.1023/A:1015566207849.
- Gadd GM. 1993. Interactions of fungi with toxic metals. New Phytol. 124:25–60. doi:10.1111/j.1469-8137.1993.tb03796.x.
- Gill SS, Tuteja N. 2010. Reactive oxygen species and antioxidant machinery in abiotic stress tolerance in crop plants. Plant Physiol Biochem. 48:909–930. doi:10.1016/j.plaphy.2010.08.016.
- Gordon SA, Weber RP. 1951. Colorimetric estimation of indoleacetic acid. Plant Physiol. 26:192–195. doi:10.1104/pp.26.1.192.
- Haruma T, Doyama K, Lu X, Noji K, Masuya H, Arima T, Tomiyama S, Yamaji K. 2023. Miscanthus sinensis contributes to the survival of Pinus densiflora seedlings at a mining site via providing a possible functional endophyte and maintaining symbiotic relationship between P. densiflora and endophytes from high soil temperature stress. PLoS One. 18:e0286203. doi:10.1371/journal.pone.0286203.
- Haruma T, Yamaji K, Masuya H. 2021. Phialocephala fortinii increases aluminum tolerance in Miscanthus sinensis growing in acidic mine soil. Lett Appl Microbiol. 73:300–307. doi:10.1111/lam.13514.
- Haruma T, Yamaji K, Masuya H, Hanyu K. 2018. Root endophytic Chaetomium cupreum promotes plant growth and detoxifies aluminum in Miscanthus sinensis Andersson growing at the acidic mine site. Plant Species Biol. 33:109–122. doi:10.1111/1442-1984.12197.
- Haruma T, Yamaji K, Ogawa K, Masuya H, Sekine Y, Kozai N. 2019. Root-endophytic Chaetomium cupreum chemically enhances aluminium tolerance in Miscanthus sinensis via increasing the aluminium detoxicants, chlorogenic acid and oosporein. PLoS One. 14:e0212644. doi:10.1371/journal.pone.0212644.
- Heo I, Hong K, Yang H, Lee HB, Choi YJ, Hong SB. 2019. Diversity of Aspergillus, Penicillium, and Talaromyces species isolated from freshwater environments in Korea. Mycobiology. 47:12–19. doi:10.1080/12298093.2019.1572262.
- Hiroi T. 1974. Phytosociological research in copper mine vegetation, Japan. J Humanit Nat Sci. 38:177–226. In Japanese.
- Hiroi T. 1980. Vegetation in area around heavy metal mines and smelters. J Humanit Nat Sci. 55:63–98. In Japanese.
- Hutchinson GE. 1945. Aluminum in soils, plants, and animals. Soil Sci. 60:29–40. doi:10.1097/00010694-194507000-00004.
- Järup L. 2003. Hazards of heavy metal contamination. Br Med Bull. 68:167–182. doi:10.1093/bmb/ldg032.
- Jiang H, Zhao X, Fang J, Xiao Y. 2018. Physiological responses and metal uptake of Miscanthus under cadmium/arsenic stress. Environ Sci Pollut Res. 25:28275–28284. doi:10.1007/s11356-018-2835-z.
- Jin HQ, Liu HB, Xie YY, Zhang YG, Xu QQ, Mao LJ, Li XJ, Lin CJ, Zhang FC, L C. 2018. Effect of the dark septate endophytic fungus Acrocalymma vagum on heavy metal content in tobacco leaves. Symbiosis. 74:89–95. doi:10.1007/s13199-017-0485-4.
- Jumpponen ARI, Trappe JM. 1998. Dark septate endophytes: a review of facultative biotrophic root-colonizing fungi. New Phytol. 140:295–310. doi:10.1046/j.1469-8137.1998.00265.x.
- Jung C, Maeder V, Funk F, Frey B, Sticher H, Frossard E. 2003. Release of phenols from Lupinus albus L. roots exposed to Cu and their possible role in Cu detoxification. Plant Soil. 252:301–312. doi:10.1023/A:1024775803759.
- Kabata-Pendias A. 2011. Trace elements in soils and plants, 4th edition. Boca Raton: CRC Press.
- Kamiga M, Tejiri M. 2003. The present pollution of river-bed sediments and soil in the Watarase River basin and the Miyata River basin: the pollution level after abandoned working of the Ashio copper mine and the Hitachi mine. J Geol Soc Jpn. 109:533–547. doi:10.5575/geosoc.109.533. In Japanese.
- Kayama M. 2001. Comparison of the aluminum tolerance of Miscanthus sinensis Anderss. and Miscanthus sacchariflorus Bentham in hydroculture. Int J Plant Sci. 162:1025–1031. doi:10.1086/322890.
- Khodaverdiloo H, Han FX, Taghlidabad RH, Karimi A, Moradi N, Kazery JA. 2020. Potentially toxic element contamination of arid and semi-arid soils and its phytoremediation. Arid Land Res Manage. 34:361–391. doi:10.1080/15324982.2020.1746707.
- Kısa D, Elmastaş M, Öztürk L, Kayır Ö. 2016. Responses of the phenolic compounds of Zea mays under heavy metal stress. Appl Biol Chem. 59:813–820. doi:10.1007/s13765-016-0229-9.
- Kołodyńska D. 2011. Chelating agents of a new generation as an alternative to conventional chelators for heavy metal ions removal from different waste waters. In: Ning R Y., editor. Expanding issues in desalination. Rijeka: IN TECH d.o.o.; p. 339–370.
- Kubartová A, Ranger J, Berthelin J, Beguiristain T. 2009. Diversity and decomposing ability of saprophytic fungi from temperate forest litter. Microb Ecol. 58:98–107. doi:10.1007/s00248-008-9458-8.
- Larcher W. 2003. Physiological plant ecology 4th edition. In Ökophysiologie derPflanzen, 6th ed.; Huber Sannwald E, Translator. Berlin: Springer.
- Li T, Liu MJ, Zhang XT, Zhang HB, Sha T, Zhao ZW. 2011. Improved tolerance of maize (Zea mays L.) to heavy metals by colonization of a dark septate endophyte (DSE) Exophiala pisciphila. Sci Total Environ. 409:1069–1074. doi:10.1016/j.scitotenv.2010.12.012.
- Lu X, Yamaji K, Haruma T, Yachi M, Doyama K, Tomiyama S. 2021. Metal accumulation and tolerance in Artemisia indica var. maximowiczii (Nakai) H. Hara. and Fallopia sachalinensis (F. Schmidt) Ronse Decr., a naturally growing plant species at mine site. Minerals. 11:806. doi:10.3390/min11080806.
- Ma Y, Prasad MNV, Rajkumar M, Freitas HJBA. 2011. Plant growth promoting rhizobacteria and endophytes accelerate phytoremediation of metalliferous soils. Biotechnol Adv. 29:248–258. doi:10.1016/j.biotechadv.2010.12.001.
- Malicka M, Magurno F, Piotrowska-Seget Z. 2022. Plant association with dark septate endophytes: when the going gets tough (and stressful), the tough fungi get going. Chemosphere. 302:134830. doi:10.1016/j.chemosphere.2022.134830.
- Martino E, Perotto S, Parsons R, Gadd GM. 2003. Solubilization of insoluble inorganic zinc compounds by ericoid mycorrhizal fungi derived from heavy metal polluted sites. Soil Biol Biochem. 35:133–141. doi:10.1016/S0038-0717(02)00247-X.
- McGonigle TP, Miller MH, Evans DG, Fairchild GL, Swan JA. 1990. A new method which gives an objective measure of colonization of roots by vesicular – arbuscular mycorrhizal fungi. New Phytol. 115:495–501. doi:10.1111/j.1469-8137.1990.tb00476.x.
- Mehmood A, Hussain A, Irshad M, Hamayun M, Iqbal A, Khan N. 2019. In vitro production of IAA by endophytic fungus Aspergillus awamori and its growth promoting activities in Zea mays. Symbiosis. 77:225–235. doi:10.1007/s13199-018-0583-y.
- Mendez MO, Maier RM. 2008. Phytostabilization of mine tailings in arid and semiarid environments – an emerging remediation technology. Environ Health Perspect. 116:278–283. doi:10.1289/ehp.10608.
- Meng S, Cao J, Feng Q, Peng J, Hu Y. 2013. Roles of chlorogenic acid on regulating glucose and lipids metabolism: a review. Evid Based Complem Alternat Med. doi:10.1155/2013/801457.
- Miao F, Yang R, Chen DD, Wang Y, Qin BF, Yang XJ, Zhou L. 2012. Isolation, identification and antimicrobial activities of two secondary metabolites of Talaromyces verruculosus. Molecules. 17:14091–14098. doi:10.3390/molecules171214091.
- Mongkhonsin B, Nakbanpote W, Hokura A, Nuengchamnong N, Maneechai S. 2016. Phenolic compounds responding to zinc and/or cadmium treatments in Gynura pseudochina (L.) DC extracts and biomass. Plant Physiol Biochem. 109:549–560. doi:10.1016/j.plaphy.2016.10.027.
- Naveed M, Hejazi V, Abbas M, Kamboh AA, Khan GJ, Shumzaid M, Ahmad F, Babazadeh D, Xia FF, Modarresi-Ghazani F, et al. 2018. Chlorogenic acid (CGA): a pharmacological review and call for further research. Biomed Pharmacother. 97:67–74. doi:10.1016/j.biopha.2017.10.064.
- Ohtsuka T, Sakura T, Ohsawa M. 1993. Early herbaceous succession along a topographical gradient on forest clear-felling sites in mountainous terrain, central Japan. Ecol Res. 8:329–340. doi:10.1007/BF02347192.
- Parveen I, Wilson T, Donnison IS, Cookson AR, Hauck B, Threadgill MD. 2013. Potential sources of high value chemicals from leaves, stems and flowers of Miscanthus sinensis ‘Goliath’ and Miscanthus sacchariflorus. Phytochemistry. 92:160–167. doi:10.1016/j.phytochem.2013.04.004.
- Pourret O, Hursthouse A. 2019. It’s time to replace the term “heavy metals” with “potentially toxic elements” when reporting environmental research. Int J Environ Res Public Health. 16:4446. doi:10.3390/ijerph16224446.
- Prajapati K, Modi HA. 2012. The importance of potassium in plant growth – a review. Indian J Plant Sci. 1:177–186.
- Read DJ, Haselwandter K. 1981. Observations on the mycorrhizal status of some alpine plant communities. New Phytol. 88:341–352. doi:10.1111/j.1469-8137.1981.tb01729.x.
- Rodriguez RJ, White JF, Arnold AE, Redman RS. 2009. Fungal endophytes: diversity and functional roles. New Phytol. 182:314–330. doi:10.1111/j.1469-8137.2009.02773.x.
- Samson RA, Yilmaz N, Houbraken J, Spierenburg H, Seifert KA, Peterson SW, Varga J, Frisvad JC. 2011. Phylogeny and nomenclature of the genus Talaromyces and taxa accommodated in Penicillium subgenus Biverticillium. Stud Mycol. 70:159–183. doi:10.3114/sim.2011.70.04.
- Sawyer DT. 1964. Metal-gluconate complexes. Chem Rev. 64:633–643. doi:10.1021/cr60232a003.
- Schulz B, Boyle C, Draeger S, Römmert AK, Krohn K. 2002. Endophytic fungi: a source of novel biologically active secondary metabolites. Mycol Res. 106:996–1004. doi:10.1017/S0953756202006342.
- Schulz B, Römmert AK, Dammann U, Aust HJ, Strack D. 1999. The endophyte-host interaction: a balanced antagonism? Mycol Res. 103:1275–1283. doi:10.1017/S0953756299008540.
- Sherameti I, Tripathi S, Varma A, Oelmüller R. 2008. The root-colonizing endophyte Pirifomospora indica confers drought tolerance in Arabidopsis by stimulating the expression of drought stress-related genes in leaves. Mol Plant Microbe Interact. 21:799–807. doi:10.1094/MPMI-21-6-0799.
- Smith FA, Smith SE. 1997. Tansley review no. 96 structural diversity in (vesicular) arbuscular mycorrhizal symbioses. New Phytol. 137:373–388. doi:10.1046/j.1469-8137.1997.00848.x.
- Soviguidi DRJ, Pan R, Liu Y, Rao L, Zhang W, Yang X. 2022. Chlorogenic acid metabolism: the evolution and roles in plant response to abiotic stress. Phyton Int J Exp Bot. 91:239–255. doi:10.32604/phyton.2022.018284.
- Stoyke G, Currah RS. 1991. Endophytic fungi from the mycorrhizae of alpine ericoid plants. Can J Bot. 69:347–352. doi:10.1139/b91-047.
- Sun X, Song B, Xu R, Zhang MM, Gao P, Lin HZ, Sun WM. 2021. Root-associated (rhizosphere and endosphere) microbiomes of the Miscanthus sinensis and their response to the heavy metal contamination. J Environ Sci. 104:387–398. doi:10.1016/j.jes.2020.12.019.
- Suzuki S, Hosoe T, Nozawa K, Kawai KI, Yaguchi T, Udagawa SI. 2000. Antifungal substances against pathogenic fungi, talaroconvolutins, from Talaromyces convolutus. J Nat Prod. 63:768–772. doi:10.1021/np990371x.
- Tsuyuzaki S. 1995. Ski slope vegetation in central Honshu, Japan. Environ Manag. 19:773–777. doi:10.1007/BF02471959.
- Wang JL, Li T, Liu GY, Smith JM, Zhao ZW. 2016. Unraveling the role of dark septate endophyte (DSE) colonizing maize (Zea mays) under cadmium stress: physiological, cytological and genic aspects. Sci Rep. 6:22028. doi:10.1038/srep22028.
- Wang M, Yang L, Feng L, Hu F, Zhang F, Ren J, Qiu Y, Wang Z. 2019. Verrucculosins A–B, new oligophenalenone dimers from the soft coral-derived fungus Talaromyces verruculosus. Mar Drugs. 17:516. doi:10.3390/md17090516.
- Waqas M, Khan AL, Kamran M, Hamayun M, Kang SM, Kim YH, Lee IJ. 2012. Endophytic fungi produce gibberellins and indole acetic acid and promotes host-plant growth during stress. Molecules. 17:10754–10773. doi:10.3390/molecules170910754.
- Wasay SA, Barrington SF, Tokunaga S. 1998. Organic acids to remediate a clay loam polluted by heavy metals. Can Agric Eng. 40:9–15.
- White TJ, Bruns T, Lee S, Taylor J. 1990. Amplification and direct sequencing of fungal ribosomal RNA genes for phylogenetics. In: Innis MA, Gelfand D H, Sninsky JJ, White TJ, editor. PCR protocols: a guide to methods and applications. New York: Academic Press; p. 315–322.
- Wigney DI, Allan GS, Hay LE, Hocking AD. 1990. Osteomyelitis associated with Penicillium verruculosum in a German shepherd dog. J Small Anim Pract. 31:449–452. doi:10.1111/j.1748-5827.1990.tb00512.x.
- Wilson D. 1995. Endophyte: the evolution of a term, and clarification of its use and definition. Oikos. 73:274–276. doi:10.2307/3545919.
- Xu JG, Hu QP, Liu Y. 2012. Antioxidant and DNA-protective activities of chlorogenic acid isomers. J Agric Food Chem. 60:11625–11630. doi:10.1021/jf303771s.
- Xue L, Liu J, Shi S, Wei Y, Chang E, Gao M, Chen L, Jiang Z. 2014. Uptake of heavy metals by native herbaceous plants in an antimony mine (Hunan, People’s Republic of China). Soil Air Water. 42:81–87. doi:10.1002/clen.201200490.
- Xue L, Ren H, Li S, Gao M, Shi S, Chang E, Wei Y, Yao X, Jiang Z, Liu J. 2015. Comparative proteomic analysis in Miscanthus sinensis exposed to antimony stress. Environ Pollut. 201:150–160. doi:10.1016/j.envpol.2015.03.004.
- Yamaji K, Fukushi Y, Hashidoko Y, Yoshida T, Tahara S. 1999. Characterization of antifungal metabolites produced by Penicillium species isolated from seeds of Picea glehnii. J Chem Ecol. 25:1643–1653. doi:10.1023/A:1020849202413.
- Yamaji K, Ichihara Y. 2012. The role of catechin and epicatechin in chemical defense against damping-off fungi of current-year Fagus crenata seedlings in natural forest. For Pathol. 42:1–7. doi:10.1111/j.1439-0329.2010.00709.x.
- Yamaji K, Nagata S, Haruma T, Ohnuki T, Kozaki T, Watanabe N, Nanba K. 2016b. Root endophytic bacteria of a 137Cs and Mn accumulator plant, Eleutherococcus sciadophylloides, increase 137Cs and Mn desorption in the soil. J Environ Radioact. 153:112–119. doi:10.1016/j.jenvrad.2015.12.015.
- Yamaji K, Watanabe Y, Masuya H, Shigeto A, Yui H, Haruma T. 2016a. Root fungal endophytes enhance heavy-metal stress tolerance of Clethra barbinervis growing naturally at mining sites via growth enhancement, promotion of nutrient uptake and decrease of heavy-metal concentration. PLoS One. 11:e0169089. doi:10.1371/journal.pone.0169089.
- Yang L, Wen KS, Ruan X, Zhao YX, Wei F, Wang Q. 2018. Response of plant secondary metabolites to environmental factors. Molecules. 23:762. doi:10.3390/molecules23040762.
- Yoon J, Cao X, Zhou Q, Ma LQ. 2006. Accumulation of Pb, Cu, and Zn in native plants growing on a contaminated Florida site. Sci Total Environ. 368:456–464. doi:10.1016/j.scitotenv.2006.01.016.
- Zhao A, Gao L, Chen B, Feng L. 2019. Phytoremediation potential of Miscanthus sinensis for mercury-polluted sites and its impacts on soil microbial community. Environ Sci Pollut Res. 26:34818–34829. doi:10.1007/s11356-019-06563-3.
- Zhao H, Wang B, He J, Yang J, Pan L, Sun D, Peng J. 2013. Genetic diversity and population structure of Miscanthus sinensis germplasm in China. PLoS One. 8:e75672. doi:10.1371/journal.pone.0075672.
- Zhou H, Zeng M, Zhou X, Liao BH, Liu J, Lei H, Zhong QY, Zeng H. 2013. Assessment of heavy metal contamination and bioaccumulation in soybean plants from mining and smelting areas of southern Hunan Province, China. Environ Toxicol Chem. 32:2719–2727. doi:10.1002/etc.2389.
- Zunino H, Martin JP. 1977. Metal-binding organic macromolecules in soil: 1. Hypothesis interpreting the role of soil organic matter in the translocation of metal ions from rocks to biological systems. Soil Sci. 123:65–76. doi:10.1097/00010694-197702000-00001.