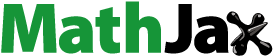
Abstract
In this work, nano-sized mixed oxides of Mn3O4 and CuO were successfully prepared by a simple hydrothermal method and used as an effective heterogeneous sono-Fenton catalyst for p-nitrophenol (PNP) degradation at the neutral condition. The prepared nanocomposite was characterised by X-ray diffraction (XRD), field-emission scanning electron microscopy (FE-SEM), energy-dispersive X-ray spectroscopy (EDS), Fourier transform infrared spectroscopy (FTIR) and N2 adsorption–desorption analysis. The results showed that the Mn3O4.CuO nanocomposite can catalyze efficiently the decomposition of PNP under ultrasonic irradiation in comparison with pristine Mn3O4 and CuO. The almost complete degradation of PNP was achieved within 20 min at pH 8.0 by using 0.1 mol/L of H2O2 and 0.01 g of the catalyst. Also, the stability and reusability test showed that the prepared nanocomposite had good stability during the Fenton process. Finally, the main oxidative species involved in the sono-Fenton process based on the results of radical species trapping experiments were identified.
1. Introduction
p-Nitrophenol (PNP) as one of the important phenolic derivatives is widely used as a precursor or intermediate in several industries, including the production of paints, plastics, explosives, organo-phosphorus pesticides, dyes and pharmaceuticals [Citation1,Citation2]. Because of its toxicity, potentially carcinogenic and mutagenic effects, when the industrial effluents containing PNP were discharged into the water body, which could have adverse effects on public health and living resources[Citation1]. Besides, due to high stability and solubility, it is difficult to be degraded by natural physical and biological processes and can persist in the environment for a long time. Thus, some efficient treatment processes including adsorption [Citation3–5], biodegradation [Citation6,Citation7], photodegradation [Citation8,Citation9], and advanced oxidation processes (AOPs) [Citation1,Citation10,Citation11] were developed to remove of PNP from wastewater.
Among these, AOP seems to be a popular choice due to its operational simplicity, mild temperature and pressure condition of in-situ generation of potent oxidizing species, i.e. hydroxyl radicals (•OH) and superoxide radical anions (•O2−), which can attack and decompose most of the organic molecule to smaller molecules and finally mineralise them to non-toxic CO2 and H2O [Citation12]. In the Fenton method, as one of the AOPs, •OH species is generated by decomposition of H2O2 in the presence of dissolved ferrous salts as a homogeneous catalyst. Due to its high removal efficiency, simplicity, and low operating cost, this method is popular and has been widely used [Citation13]. However, it suffers from the narrow acidic pH range, a large amount of sludge formation, and impossible regeneration of catalyst [Citation14]. To overcome these limitations, a heterogeneous Fenton-like process has been proposed, which can be operated over a broad range of pH and without sludge as well as can be easily recovered and reused several times. In this manner, the Fenton reaction takes place at active sites on the surface of solid catalyst rather than Fe2+ ion and can decompose organic contaminants [Citation15].
Despite these benefits, mass transfer of the substrates to the catalyst surface and deactivation of active catalytic sites by adsorbed organic species are two main limitations of heterogeneous Fenton-like process [Citation16,Citation17]. It was found that the hybrid strategy based on coupling ultrasonic irradiation (US) and the heterogeneous catalytic processes can overcome these limitations [Citation18,Citation19]. When the US enters into a liquid medium, convection can be created by different type of physical phenomena such as microturbulence, microstreaming, and shock waves [Citation20]. These phenomena can accelerate the mass transfer rate, break up the catalyst particles into small particles with higher surface area and increases the activity of the catalyst due to the continuous cleaning of the surface catalyst [Citation21].
Although many studies have been reported for the degradation of a wide range of pollutants by coupling US irradiation and heterogeneous catalytic process [Citation15,Citation18,Citation19], there are only a few studies where this hybrid strategy has been used to the elimination of nitrophenol compounds from aqueous solution. Bagal et al. [Citation22] utilised ultrasound irradiation and Fenton reaction (FeSO4/H2O2) as well as Fenton like reactions (Fe0/H2O2 and CuO/H2O2) to remove 2,4-dinitrophenol from aqueous solution. Only 5.8% of the 2,4-dinitrophenol was removed by ultrasound irradiation, while the degradation efficiency was significantly increased when the Fenton process was combined with US irradiation. ElShafei et al. [Citation23] studied the removal of 2-nitrophenol by combined US and UV irradiations and metal oxychlorides (Fe, Cu, Bi, and Zn) as heterogeneous Fenton catalysts. Degradation of 2-nitrophenol was studied by using individual and combined processes over these catalysts, and the results showed that the sonophotocatalytic system was more efficient than separate processes. Mishra et al. [Citation24] found that the degradation efficiency of PNP was increased when the US was combined with heterogeneous catalysts (TiO2 and CuO). Based on obtained results, TiO2 particle shows a higher catalytic activity in comparison to CuO particle, which may be attributed to the fact that TiO2 particle provides activated surface area that can accelerate •OH production and also providing nuclei for cavitation. In contrast, CuO particle only provides additional nuclei for cavitation.
In the present study, copper–manganese mixed oxide has been prepared by a facile one-step hydrothermal procedure and applied as a novel heterogeneous Fenton catalyst for the US-assisted oxidative degradation of PNP. XRD, FE‒SEM, EDS, FT-IR and N2 adsorption–desorption techniques were used to investigate crystalline structure, morphology, chemical composition and textural property of the prepared nanocomposite. The influence of mass of catalyst, solution pH, sonication time, and concentration of H2O2 on the removal of PNP were investigated. Also, the main oxidative species produced during the catalytic oxidative degradation of PNP over CuO.Mn3O4 nanoparticles were identified.
2. Materials and methods
2.1. Materials
Manganese nitrate (Mn(NO3)2·4H2O), copper nitrate (Cu(NO3)2·3H2O), hydrogen peroxide, p − nitrophenol (PNP), sodium hydroxide, hydrochloric acid, chloroform, tert-Butyl alcohol were purchased from Merck. A 100 mg L−1 stock solution of PNP was prepared by dissolving the appropriate amount of PNP (0.050 gr) in 50.0 mL of 0.01 mol L−1 NaOH. Standard solution of 10.0 mg L−1 PNP was prepared daily by appropriate dilution of the stock solution with double distilled water.
2.2. Preparation of Mn3O4·CuO nanocomposite
Mn3O4·CuO nanocomposite was prepared by a facile one-pot hydrothermal approach. Typically, 3.00 g of Mn(NO3)2·4H2O and 1.45 g of Cu(NO3)2·3H2O were dissolved in 20.0 mL of NaOH solution (0.01 mol L−1), and resulting suspension was stirred for a few minutes. The pH of the suspension was adjusted between 11.0 and 11.5 and loaded into a Teflon-lined stainless-steel autoclave (25 mL capacity) and maintained for three hours at 120 °C. The obtained nanocomposite was collected, washed several times with water and ethanol (1:1) and dried in an oven at 70 °C for two hours.
2.3. Characterization
The surface morphology and composition of the as-prepared Mn3O4·CuO nanocomposite was investigated with a Mira 3-XMU field emission scanning electron microscope equipped with an energy dispersive X-ray spectrometer (EDS). FTIR spectra of Mn3O4, CuO, and Mn3O4·CuO samples were recorded with an IR spectrophotometer (Bruker, Tensor 27) from 360 to 4000 cm‒1. Nitrogen adsorption-desorption studies were carried out at 77 K using a Belsorp-mini II instrument (Bel, Japan). XRD spectra of the as-prepared samples were achieved from 5° to 90° with a Bruker D8 Advance diffractometer, operated at 40 kV and 100 mA. The metallic component content of the nanocomposite was analyzed by inductively coupled plasma-optical emission spectrometry (Perkin Elmer, Optima 7300DV).
2.4. Degradation process
The catalytic performance of the prepared nanocomposite for the degradation of PNP by US irradiation in the presence of H2O2 was examined in the batch experiments. In a typical experiment, 0.015 g of nanocatalyst was added into 25.0 mL of 10.0 mg L−1 PNP, and the pH of the suspension was adjusted at 8.0 by adding NaOH or HCl. Then, the flask was placed in an ultrasonic bath (Elma SH30, 37 kHz, Italy), and the degradation reaction was initiated by adding 0.5 mL of H2O2 (30%) into PNP solution. After 20 min sonication, an aliquot of 0.5 mL of suspension was taken and centrifuged by Hettich EBA20 at 5000 rpm for 1 min. The supernatants were transferred into a 10-mm micro quartz cuvette, and the absorbance spectra were recorded (wavelength range of 250–530 nm) on a UV–Visible spectrophotometer (PG Instrument, T80+). The degradation extent of PNP was measured at a wavelength of 400 nm.
3. Results and discussion
3.1. Characterization of the Mn3O4·CuO nanocomposite
illustrates the XRD pattern of the Mn3O4, CuO, and Mn3O4.CuO samples. The diffraction peaks at 35.5, 38.6, 48.8, 58.2, 61.5, 65.6 and 68.1° can be indexed to (), (1 1 1), (
), (2 0 2), (
), (0 2 2) and (2 2 0) crystal planes of monoclinic phase of CuO (JCPDS card no. 01-080-0076). In the pattern of the Mn3O4 sample, the main diffraction peaks appeared at 2θ values of 18.0, 28.9, 32.4, 36.1, 44.4, 59.9 and 64.6° were attributed to (1 0 1), (1 1 2), (1 0 3), (2 1 1), (2 2 0), (2 2 4) and (4 0 0) crystal planes of Mn3O4. The observed diffraction patterns of pristine Mn3O4 match well with crystal planes of the tetragonal structure of Mn3O4 (JCPDS Card 01-080-0382). The Mn3O4.CuO nanocomposite showed a very similar diffraction pattern as the Mn3O4 sample with additional weak diffraction peaks at 38.6, 32.5, 48.8° because of the presence of CuO. These results suggested that the obtained nanocomposite is formed of Mn3O4, and CuO, and any new compound were not formed.
FT-IR spectra of the CuO, Mn3O4, and Mn3O4.CuO samples are shown in . The presence of three characteristic bands at 422, 520, and 609 cm−1 which appear at FT-IR spectrum of pristine CuO attributed to the of νCu–O modes of monoclinic − phase CuO [Citation25]. The origin of the characteristic bands at 504 and 609 cm−1 are due to Cu–O stretching along the (−2 0 2) direction, while the origin of the band at 412 cm−1 is assigned to Cu–O stretching along the (2 0 2) direction [Citation26]. The two bands at 1384 and 2426 cm−1 can be attributed to atmospheric CO2 molecules that are adsorbed to the CuO surface [Citation27]. The observed bands at 1629 and 3423 cm−1 are assigned to the O–H stretching and bending vibrations of water remaining, respectively [Citation26]. The FT-IR spectrum of Mn3O4 shows three bands at 412, 518, and 628 cm−1 corresponding to the characteristic stretching vibrational modes of Mn–O bond in the spinel structure of Mn3O4 [Citation28,Citation29]. Similarly, the bands at 1384 and 2424 cm−1 are assigned to the adsorbed CO2 molecules on the surface of Mn3O4 nanoparticles, and the bands observed at 1631, and 3440 cm−1 are attributed to the O–H stretching and bending vibrations of water remaining, respectively. Three broad bands observed at 424, 534 and 630 cm−1 originate from the overlapping characteristic stretching vibrational modes of Mn–O and Cu–O bonds in the prepared Mn3O4·CuO nanocomposite. Likewise, the FTIR spectrum of Mn3O4·CuO sample exhibits similar bands at 11629, 1384, and 3438 cm−1. The presence of characteristic vibration bands between 400 cm−1 and 650 cm−1 with a little shift in the prepared nanocomposite suggests that the nanoparticles are composed of Mn3O4 and CuO.
The surface area and pore size distribution of pristine Mn3O4 and Mn3O4·CuO nanocomposite material was estimated by N2 adsorption–desorption measurements at 77 K. The N2 adsorption–desorption isotherms and the corresponding pore size distribution curves determined by Barrett–Joyner–Halenda (BJH) method of the Mn3O4 and Mn3O4·CuO samples are shown in . Two samples exhibit typical V adsorption isotherm with H3 hysteresis loops, which suggests the mesoporous nature of the catalysts [Citation30]. The obtained results exhibit that the BET specific surface area of the Mn3O4·CuO nanocomposite was 23.64 m2g−1 which are higher than that of pristine Mn3O4 (14.89 m2g−1). Also, Pore volume of nanocomposite was found to be 5.43 cm3g−1, which was 1.6 times higher than those of pristine Mn3O4 (3.42 cm3g−1). The pore-size distribution of samples based on the desorption branch of the nitrogen isotherm and BJH calculation method were measured. The most pore diameters in pristine Mn3O4 were around 12 nm, which reflects the mesoporous nature of the Mn3O4 sample. On the other hand, the majority of pores of Mn3O4·CuO nanocomposite are located at pore diameters of 1.64, 4.60, 12.21, 25.54, and 46.13 nm, indicating the existence of micro- and mesopores in the Mn3O4·CuO nanocomposite.
Figure 3. N2 adsorption and desorption isotherms for the Mn3O4 and Mn3O4.CuO samples (a); pore size distribution of Mn3O4 and Mn3O4.CuO (b).
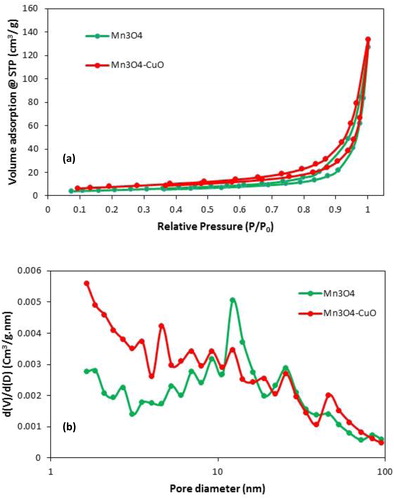
The FE-SEM image of the Mn3O4·CuO nanocomposite is shown in . The prepared nanocomposite showed a uniform spherical structure with severe aggregation. The FE-SEM image indicates that the Mn3O4.CuO nanoparticles have diameters of 15–40 nm. As shown in , the FE-SEM-EDS spectrum confirmed the presence of the copper, manganese, and oxygen elements in the prepared nanoparticles. The results of EDS analysis showed that the content of Cu and Mn elements in a single particle of the nanocomposite was 26.6 and 44.2 wt%, respectively. According to percentage weight, the mole ratio of Cu: Mn was found to be 1:1.92, which is very close to the results of the ICP-OES (i.e. 1:1.94) that represent a uniform distribution of components in nanocomposite. Also, the selected area elemental mapping in confirmed the clear homogeneous distribution of manganese, copper, and oxygen in the prepared sample.
3.2. Fenton catalytic activity of Mn3O4·CuO nanocomposite
Firstly, the degradation of the PNP in aqueous solution was done using different processes, namely H2O2, Mn3O4·CuO and H2O2/Mn3O4·CuO (with stirring and sonication). As shown in , nanocatalyst and hydrogen peroxide individually have no ability to degradation of the PNP after 20 min of mechanical stirring. In the meanwhile, almost 54% of PNP was removed by H2O2/Mn3O4·CuO process. Almost no PNP was degraded by H2O2 after 20 min of US irradiation, and only 7% of PNP was removed by using Mn3O4·CuO and US irradiation. The degradation efficiency of PNP was significantly increased and reached to more than 95% when H2O2 and Mn3O4·CuO was combined with US irradiation. It is obvious that in the absence of catalyst or H2O2, processes had little capability to degradation of the PNP under US irradiation, while in the presence of Mn3O4·CuO nanoparticles and H2O2 almost all of PNP degraded after 20 min. The high degradation of the PNP by H2O2/Mn3O4·CuO process indicated that the nanocatalyst and US irradiation play leading roles in generating reactive oxygen species for oxidative degradation of PNP.
The catalytic activity of prepared nanocomposites with various mole ratios of Cu2+ and Mn2+ precursors (1:1, 1:2, and 2:1) were evaluated. The catalyst Mn3O4.CuO (1:1) and Mn3O4·CuO (1:2) in the presence of H2O2 exhibit 81 and 83% of PNP degradation after 20 min US irradiation, while Mn3O4·CuO (2:1) catalyst showed more than 95% degradation under the same condition. Also, the catalytic activity of Mn3O4·CuO (2:1) nanocomposite was compared with those of pristine Mn3O4 and CuO samples. The catalyst Mn3O4 and CuO in the presence of H2O2 exhibit 12.2% and 39.8% of PNP degradation after 20 min US irradiation, respectively, while with Mn3O4·CuO nanocomposite the degradation efficiency could reach to 95.0%. This higher activity can be attributed to the greater surface area and hence the larger number of available sites for catalysis compared to pristine metal oxides. Also, this observation can be attributed to the synergic effect between the Mn3O4 and CuO nanoparticles. The synergistic effect might be caused by redox interactions between Mn3+/Mn2+ and Cu2+/Cu+ redox couples, which facilitated regeneration of active Mn2+ and Cu2+ on the catalyst. An in situ experiment was performed to verify the synergistic effect between CuO and Mn3O4. The physical mixture of Mn3O4 and CuO with a mole ratio of 2:1 yielded a degradation efficiency of 53% for PNP, which was significantly lower than that of the hydrothermally synthesised Mn3O4·CuO nanocomposite. This suggests that the catalytic activity of nanocomposite derived from the synergistic effect between these two metal oxides, which may be related to more efficient interfacial charge transfer [Citation31].
In order to obtain the best degradation efficiency of PNP by the proposed process, the effects of main operating parameters (pH, nanocatalyst mass, and initial concentration of H2O2) have been studied. The degradation efficiency of PNP was examined in various amounts of nanocatalyst mass (0.005–0.02 g). According to , after exposure of US radiation for 20 min to PNP solution in the absence of Mn3O4·CuO nanocomposite, almost no degradation was observed, while even at a low content of Mn3O4·CuO nanocomposite (0.005 g), high degradation efficiency can be achieved. An increase of nanocatalyst amount to 0.01 g, led to the enhancement of degradation of PNP to 95% and became constant for further increasing Mn3O4·CuO nanocomposite amount up to 0.02 g. Thus, 0.01 g of nanocatalyst has been considered as the optimal value for the removal of PNP.
Figure 6. Influence of different operation parameters on PNP degradation. Catalyst mass (a) (experimental conditions: initial concentration of H2O2: 0.1 mol L-1, sonication time: 20 min and pH: 8.0); H2O2 concentration (b) (experimental conditions: catalyst mass: 0.015 g, sonication time: 20 min and pH: 8.0); pH (c) (experimental conditions: catalyst mass: 0.015 g, sonication time: 20 min and initial concentration of H2O2: 0.1 mol L-1); Sonication time (d) (experimental conditions: catalyst mass: 0.015 g, initial concentration of H2O2: 0.1 mol L-1 and pH: 8.0).
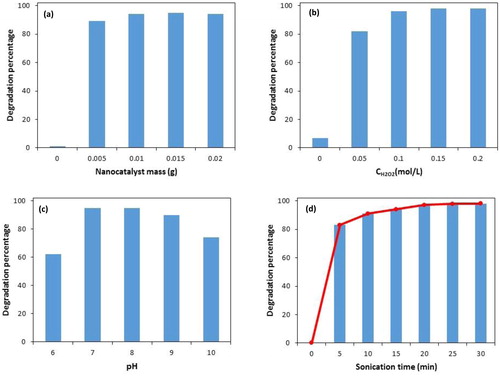
The effect of H2O2 concentration on PNP degradation with Mn3O4·CuO nanocomposite was investigated (). As it is seen, under US irradiation, only 7% PNP was degraded in the absence of H2O2, whereas the degradation efficiency increased to 82% at 0.05 mol/L H2O2 concentration. After that, by increasing the H2O2 concentration to 0.1 mol/L, the degradation efficiency of PNP was almost 96%. This enhancement is due to the increasing amount of reactive oxygen species. However, a further increase in the amount of H2O2, the degradation efficiency of PNP becomes constant. Therefore, 0.1 mol/L of H2O2 was used as the optimal value for the removal of PNP. Degradation of PNP in the absence of the H2O2 suggested that Mn3O4.CuO nanocomposite under US irradiation can catalyze the generation of hydroxyl radicals from water.
To evaluate the effect of pH on the PNP degradation, the pH of the suspensions was varied in the range 6.0–10.0. As it is seen in , 62.0% PNP was degraded at pH 6.0, whereas the degradation efficiency significantly increased to 95.0% at pH 7.0 and 8.0. After that, at pH 9.0 and 10.0, the degradation efficiency decreased to 90.0 and 74.0%, respectively. The low degradation of PNP at alkaline conditions may be related to the decomposition of H2O2 into H2O and O2, and as a result, •OH production rate decreases [Citation32]. The obtained results showed that in contrast to the conventional Fenton catalysts, higher degradation efficiencies are obtained by as-prepared nanocomposite under neutral and mild alkaline conditions. This feature is vital since a large amount of wastewaters that are discharged into the natural streams have neutral or basic pH values.
The changes in degradation efficiency of PNP by Mn3O4·CuO nanocomposite as a function of time were shown in . Clearly, the percentage of degradation rapidly increases with increasing US irradiation time, and it can reach 83% within 5 min. By increasing in US irradiation time up to 20 min, degradation efficiency was enhanced to above 95%, and after that, no significant change in the PNP degradation was observed.
In optimal experimental conditions, the reproducibility of the results was investigated by comparing the three independent degradation experiments. The average PNP degradation efficiency was 96.4% with relative standard deviation of 1.52%. The low RSD value indicates that the proposed procedure is highly reproducible.
3.3. Stability of Mn3O4.CuO nanocomposite
The long-term stability of the heterogeneous nanocatalyst as a vital factor in the practical application of the Fenton process was investigated. The stability and reusability of the Mn3O4·CuO nanocomposite was evaluated by five repeating degradation experiments under optimum conditions. After each experiment, the nanocomposite was separated centrifugally, washed with distilled water several times and dried at the oven. As shown in the degradation percentages of PNP in the first four-cycles were more than 85%, and after that, the catalytic activity decreased to 78%.
The leaching of Cu from the catalyst to the solution during the catalytic oxidative degradation of PNP was studied. The concentration of total dissolved copper ions among five cycles was 1.9 mg L−1 at pH 8.0, which indicates a little copper ion is leached into the solution. The decrease of catalytic activity after each cycle may be related to copper leaching, pore blocking and reduced amount of surface hydroxyl groups [Citation33,Citation34]. The results imply that the prepared nanocomposite has good stability and is reused at least five times.
3.4. The implication of reactive oxidizing species
It is well-known that the most reactive species in Fenton based pollutant degradation are •OH and •O2− [Citation35,Citation36]. Hydroxyl radicals as the highly reactive agent are generated in homogeneous and heterogeneous Fenton processes and able to degrade toxic environmental compounds. At neutral pH, it has the high standard redox potential () and can be attacked to almost all organic compounds. Superoxide radical anions with standard redox potential (
) of 0.91 V at pH 7 are generated by the addition of one electron to O2 and similar to hydroxyl radicals can react with different kinds of organic molecules [Citation37]. To investigate the role of active radical species involved in the degradation of PNP, tert-butyl alcohol and chloroform were used as •OH and •O2− scavengers, respectively. tert-Butyl alcohol was chosen as a •OH scavenger because it would react most rapidly with hydroxyl radicals (k•OH = 5.2 × 108 M−1 s−1). Conversely, chloroform reacts more rapidly with •O2− (k•O2− = 3 × 1010 M−1s−1) than •OH (k•OH = 7 × 106 M−1 s−1) and it is one of the best scavengers of superoxide species [Citation38,Citation39].
As shown in , the degradation efficiency of PNP in 20 min was above 95% in the absence of tert-butyl alcohol and CHCl3. With the addition of 1 and 3 mmol tert-butyl alcohol, 49% and 24% of PNP was degraded at the same time, respectively. The results show that tert-butyl alcohol significantly inhibited degradation process; hence it can be concluded that PNP was mainly decomposed by the attack of •OH radicals and these species play a vital role in US/H2O2/Mn3O4.CuO system. Meanwhile, the results illustrated that the catalytic degradation efficiency of PNP in 20 min decreased to 65% and 60% in the presence of 1 and 3 mmol CHCl3, respectively. This result confirms that, besides •OH radicals, •O2− radicals could also contribute to the degradation process, but it was not as significant as hydroxyl radicals.
According to our results ( and ) and based on previous reports of the metal oxides in heterogeneous Fenton-like reactions [Citation40–43], the possible formation pathways of reactive oxidizing species can be explained as follows:
(1)
(1)
(2)
(2)
(3)
(3)
(4)
(4)
(5)
(5)
(6)
(6)
(7)
(7)
First, H2O2 react with ≡Cu2+ to generate hydroperoxyl (•OOH) radical (EquationEquation (1)(1)
(1) ), where ≡Cu2+ represents Cu(II) sites on the catalyst surface. The •OOH radical is not stable and react further with ≡Cu+ to produce •OH radical through EquationEquation (2)
(2)
(2) . Simultaneously, •OH and •OOH radicals are formed through the reactions of the ≡Mn2+ and ≡Mn3+ with H2O2 (EquationEquations (3)
(3)
(3) and Equation(4)
(4)
(4) ), respectively, where ≡Mn2+ and ≡Mn3+ represent Mn(II) and Mn(III) sites on the catalyst surface.
On the other hand, according to the EquationEquation (5)(5)
(5) , hydroxyl radicals are able to react with hydrogen peroxide to generate a higher concentration of •OOH radicals. As reported, the •OOH is a weak acid (pKa=4.8) and its conjugated base (•O2−) is dominant at neutral aqueous solutions [Citation44]. Although •O2− is less reactive than •OH, according to our result, •O2− radical could also contribute directly to the degradation process.
The standard redox potentials of Mn3+/Mn2+ is 1.51 V and Cu2+/Cu+ is 0.16 V; hence the electron transfer reaction between Cu+ and Mn3+ is thermodynamically favoured (EquationEquation (7)(7)
(7) ). The efficient regeneration of Mn2+ and Cu2+ could contribute to the significant increase of production reactive oxygen species and a higher degradation rate of PNP.
The use of ultrasound irradiation increases the mass transfer rate, breaks up the catalyst particles into small particles with higher surface area and promotes the activity of the catalyst due to the continuous cleaning of the surface catalyst. Therefore, formation of reactive oxidizing species due to the above mentioned reactions under ultrasound irradiation can be enhanced.
3.5. Comparison with other reported heterogeneous sono-Fenton catalysts
The comparison of catalytic dosage, sonication time and removal efficiency of proposed catalyst and reported catalysts for the degradation of nitrophenol compounds was presented in . As can be seen, the proposed catalyst displayed relatively short sonication time and also considerable removal efficiency comparing with other reported catalysts [Citation22–24,Citation45,Citation46]. The results reported in this study showed that the prepared catalyst is a potential candidate for the removal of PNP from aqueous solution.
Table 1. Comparison of the catalytic performance of some reported catalysts with Mn3O4.CuO nanocomposite for ultrasonic degradation of nitrophenol compounds.
4. Conclusions
In this study, Mn3O4.CuO nanocomposite was prepared by a facile one-step hydrothermal method and used as an efficient and stable heterogeneous Fenton catalyst for the US-assisted oxidative degradation of PNP. This nanocomposite exhibits higher catalytic degradation efficiency owing to the synergetic effect between these two metal oxides compared to the pristine Mn3O4 and CuO. Under the optimal conditions, more than 95% of PNP was achieved within 20 min. The advantages such as inexpensive and straightforward synthesis, high stability and reusability, remarkable catalytic activity in neutral pH allow considering the Mn3O4.CuO nanocomposite as being good candidate for the removal of PNP from aqueous solution.
Disclosure statement
No potential conflict of interest was reported by the authors.
Additional information
Funding
References
- Sun S-P, Lemley AT. p-Nitrophenol degradation by a heterogeneous Fenton-like reaction on nano-magnetite: process optimization, kinetics, and degradation pathways. J Mol Catal A Chem. 2011;349(1-2):71–79.
- Wu Z, Yuan X, Zhong H, et al. Enhanced adsorptive removal of p-nitrophenol from water by aluminum metal-organic framework/reduced graphene oxide composite. Sci Rep. 2016;6:25638–25638.
- Ahmed MJ, Theydan SK. Adsorptive removal of p-nitrophenol on microporous activated carbon by FeCl3 activation: equilibrium and kinetics studies. Desalin Water Treat. 2015;55(2):522–531.
- Chen J, Sun X, Lin L, et al. Adsorption removal of o-nitrophenol and p-nitrophenol from wastewater by metal–organic framework Cr-BDC. Chin J Chem Eng. 2017;25(6):775–781.
- Shen H-M, Zhu G-Y, Yu W-B, et al. Fast adsorption of p-nitrophenol from aqueous solution using β-cyclodextrin grafted silica gel. Appl Surf Sci. 2015;356:1155–1167.
- Tomei MC, Annesini MC, Rita S, et al. Biodegradation of 4-nitrophenol in a two-phase sequencing batch reactor: concept demonstration, kinetics and modelling. Appl Microbiol Biotechnol. 2008;80(6):1105–1112.
- Suja E, Nancharaiah YV, Venugopalan VP. p-Nitrophenol biodegradation by aerobic microbial granules. Appl Biochem Biotechnol. 2012;167(6):1569–1577.
- Pouretedal HR, Tavakkoli M. Photodegradation of para-nitrophenol catalyzed by Fe2O3/FeS nanocomposite. Desalin Water Treat. 2013;51(22-24):4744–4749.
- Yang L, Luo S, Li Y, et al. High efficient photocatalytic degradation of p-nitrophenol on a unique Cu2O/TiO2 p-n heterojunction network catalyst. Environ Sci Technol. 2010;44(19):7641–7646.
- Qiu Y, Zhou J, Cai J, et al. Highly efficient microwave catalytic oxidation degradation of p-nitrophenol over microwave catalyst of pristine α-Bi2O3. Chem Eng J. 2016;306:667–675.
- Yin C, Cai J, Gao L, et al. Highly efficient degradation of 4-nitrophenol over the catalyst of Mn2O3/AC by microwave catalytic oxidation degradation method. J Hazard Mater. 2016;305:15–20.
- Singh L, Rekha P, Chand S. Cu-impregnated zeolite Y as highly active and stable heterogeneous Fenton-like catalyst for degradation of Congo red dye. Sep Purif Technol. 2016;170:321–336.
- Nekoeinia M, Salehriahi F, Moradlou O, et al. Enhanced Fenton-like catalytic performance of N-doped graphene quantum dot incorporated CuCo2O4. New J Chem. 2018;42(11):9209–9220. 10.1039/C8NJ00219C
- Liu Y, Jin W, Zhao Y, et al. Enhanced catalytic degradation of methylene blue by α-Fe2O3/graphene oxide via heterogeneous photo-Fenton reactions. Appl Catal, B. 2017;206:642–652.
- Zhang M-h, Dong H, Zhao L, et al. A review on Fenton process for organic wastewater treatment based on optimization perspective. Sci Total Environ. 2019; 2019/06/20/670:110–121.
- Zhong X, Xiang L, Royer S, et al. Degradation of C.I. Acid Orange 7 by heterogeneous Fenton oxidation in combination with ultrasonic irradiation. J Chem Technol Biotechnol. 2011;86(7):970–977.
- Zhang H, Gao H, Cai C, et al. Decolorization of Crystal Violet by ultrasound/heterogeneous Fenton process. Water Sci Technol. 2013;68(11):2515–2520.
- Sathishkumar P, Mangalaraja RV, Anandan S. Review on the recent improvements in sonochemical and combined sonochemical oxidation processes – a powerful tool for destruction of environmental contaminants. Renewable Sustain Energy Rev. 2016;55:426–454.
- Eren Z. Ultrasound as a basic and auxiliary process for dye remediation: a review. J Environ Manage. 2012;104:127–141.
- Hamdaoui O. Intensification of the sorption of Rhodamine B from aqueous phase by loquat seeds using ultrasound. Desalination. 2011;271(1-3):279–286.
- Zhong X, Royer S, Zhang H, et al. Mesoporous silica iron-doped as stable and efficient heterogeneous catalyst for the degradation of C.I. Acid Orange 7 using sono–photo-Fenton process. Sep Purif Technol. 2011;80(1):163–171.
- Bagal MV, Lele BJ, Gogate PR. Removal of 2,4-dinitrophenol using hybrid methods based on ultrasound at an operating capacity of 7 L. Ultrason Sonochem. 2013;20(5):1217–1225.
- ElShafei GMS, Al-Sabagh AM, Yehia FZ, et al. Metal oxychlorides as robust heterogeneous Fenton catalysts for the sonophotocatalytic degradation of 2-nitrophenol. Appl Catal, B. 2018;224:681–691.
- Mishra KP, Gogate PR. Ultrasonic degradation of p-nitrophenol in the presence of additives at pilot scale capacity. Ind Eng Chem Res. 2012;51(3):1166–1172.
- Mageshwari K, Sathyamoorthy R. Organic free synthesis of flower-like hierarchical CuO microspheres by reflux condensation approach. Appl Nanosci. 2013;3(2):161–166.
- Anu Prathap MU, Kaur B, Srivastava R. Hydrothermal synthesis of CuO micro-/nanostructures and their applications in the oxidative degradation of methylene blue and non-enzymatic sensing of glucose/H2O2. J Colloid Interface Sci. 2012;370(1):144–154.
- Gopalakrishnan M, Purushothaman V, Ramakrishnan V, et al. The effect of nitridation temperature on the structural, optical and electrical properties of GaN nanoparticles. CrystEngComm. 2014;16(17):3584–3591.
- Dubal DP, Dhawale DS, Salunkhe RR, et al. A novel chemical synthesis and characterization of Mn3O4 thin films for supercapacitor application. Appl Surf Sci. 2010;256(14):4411–4416.
- Ahmed KAM, Huang K. Formation of Mn3O4 nanobelts through the solvothermal process and their photocatalytic property. Arabian J Chem. 2019;12(3):429–439.
- Thommes M, Kaneko K, Neimark A, et al. Physisorption of gases, with special reference to the evaluation of surface area and pore size distribution (IUPAC Technical Report). Pure Appl Chem. 2015;87(9-10):1051–1069.
- Wang Q, Guan S, Li B. 2D graphitic-C3N4 hybridized with 1D flux-grown Na-modified K2Ti6O13 nanobelts for enhanced simulated sunlight and visible-light photocatalytic performance. Catal Sci Technol. 2017;7(18):4064–4078. 10.1039/C7CY01134B
- Wang H, Jiang H, Wang S, et al. Fe3O4–MWCNT magnetic nanocomposites as efficient peroxidase mimic catalysts in a Fenton-like reaction for water purification without pH limitation. RSC Adv. 2014;4(86):45809–45815. 10.1039/C4RA07327D
- Dong Q, Chen Y, Wang L, et al. Cu-modified alkalinized g -C 3 N 4 as photocatalytically assisted heterogeneous Fenton-like catalyst. Appl Surf Sci. 2017;426:1133–1140. 07/01
- Queirós S, Morais V, Rodrigues CSD, et al. Heterogeneous Fenton’s oxidation using Fe/ZSM-5 as catalyst in a continuous stirred tank reactor. Sep Purif Technol. 2015;141:235–245.
- Muruganandham M, Suri RPS, Jafari S, et al. Recent developments in homogeneous advanced oxidation processes for water and wastewater treatment. J Int J Photoenergy. 2014;2014:1–21.
- Bokare AD, Choi W. Review of iron-free Fenton-like systems for activating H2O2 in advanced oxidation processes. J Hazard Mater. 2014;275:121–135.
- Sheng Y, Abreu IA, Cabelli DE, et al. Superoxide dismutases and superoxide reductases. Chem Rev. 2014;114(7):3854–3918.
- Wang H, Zhao Y, Su Y, et al. Fenton-like degradation of 2,4-dichlorophenol using calcium peroxide particles: performance and mechanisms. RSC Adv. 2017;7(8):4563–4571. 10.1039/C6RA26754H
- Farooq U, Danish M, Lu S, et al. Synthesis of nZVI@reduced graphene oxide: an efficient catalyst for degradation of 1,1,1-trichloroethane (TCA) in percarbonate system. Res Chem Intermed. 2017;43(5):3219–3236.
- Subbulekshmi NL, Subramanian E. Nano CuO immobilized fly ash zeolite Fenton-like catalyst for oxidative degradation of p-nitrophenol and p-nitroaniline. J Environ Chem Eng. 2017;5(2):1360–1371.
- Rhadfi T, Piquemal J-Y, Sicard L, et al. Polyol-made Mn3O4 nanocrystals as efficient Fenton-like catalysts. Appl Catal, A. 2010;386(1-2):132–139.
- Wan Z, Wang J. Degradation of sulfamethazine using Fe3O4-Mn3O4/reduced graphene oxide hybrid as Fenton-like catalyst. J Hazard Mater. 2017;324(Pt B):653–664. 2017/02/15/
- Zhu J, Zhang G, Xian G, et al. A high-efficiency CuO/CeO(2) catalyst for diclofenac degradation in fenton-like system. Front Chem. 2019;7:796–796.
- Kim S-U, Villamena FA. Reactivities of superoxide and hydroperoxyl radicals with disubstituted cyclic nitrones: a DFT study. J Phys Chem A. 2012;116(2):886–898.
- Eshaq G, Wang S, Sun H, et al. Core/shell FeVO4@BiOCl heterojunction as a durable heterogeneous Fenton catalyst for the efficient sonophotocatalytic degradation of p-nitrophenol. Sep Purif Technol. 2020;231:115915.
- Eshaq G, Wang S, Sun H, et al. Superior performance of FeVO4@CeO2 uniform core-shell nanostructures in heterogeneous Fenton-sonophotocatalytic degradation of 4-nitrophenol. J Hazard Mater. 2020;382:121059.