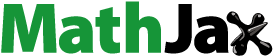
Abstract
Sol-gel process with continuous ultrasonication was used to produce pure and vanadium doped titania powders. The amount of vanadium doped in titania was varied by using different initial concentrations of vanadium solutions. Scanning electron microscopy and particle size analyses showed formation of spherical shaped particles with diameter ranging from 3 to 11 nm. The average pore volume was 0.0248 cm³/g, and the surface areas of pure and V-doped titania were 62.9 and 66.1 m2/g, respectively. The maximum amount of vanadium doped on titania catalyst, as determined using energy dispersive X-ray spectroscopy was 1.7 wt%, and the X-ray diffraction patterns showed the presence of mainly anatase form of titania. The photocatalytic activities of pure and V-doped titania were tested for methylene blue, and the metal uptake was found to increase from 10 mg/g for pure titania to 17 mg/g for V-doped titania. Adsorption isotherms for removal of lead and zinc from aqueous solutions were generated under ultraviolet exposure for 24 h, and the results revealed that the photocatalytic activity was enhanced when titania was doped with vanadium.
1. Introduction
Nano-sized titanium dioxide particles have gained wide attention in many physical and chemical applications. These include optical coating, manufacture of optoelectronic devices, photocatalysis applications for removal of dyes and heavy metals from aqueous solutions, and selective photoreduction of CO2 for solar fuels [Citation1–7]. Utilisation of nanocrystalline titania for the removal of toxic hydrocarbons and heavy metals from aqueous solutions has been shown to be effective in environmental clean-up. In this process, UV light is utilised to activate titania by the absorption of photons of ultra-band gap energy which leads to promotion of electrons (e-) from the valence band to the conduction band; this generates holes (h+) in the valance band. The photo generated e-/h+ pairs on the surface of the catalyst are highly oxidising and reducing, and could react with the adsorbed species on the catalyst surface to generate free radicals, hence can be used for the removal of heavy metal ions and hydrocarbons efficiently to obtain high reduction percentages similar to the values obtained by novel materials such as carbon materials derived from metal organic frameworks (MOFs), Zeolitic imidazolate frameworks (ZIFs) and graphene-based composites [Citation8–10].
Several methods have been used for the synthesis of nanocrystalline titania, including sputtering, spray synthesis, chemical vapour deposition, and sol-gel method. Among these, sol-gel method is the most widely used as it is relatively simple and no special equipment are required. In order to enhance the photocatalytic activity of titania, it is crucial to prevent recombination of the photogenerated e-/h+ pair. One way to achieve this is by incorporating other metal oxides into the matrix of titania, which is known as doping [Citation11]. The doped metals act as promoters on the surface to prevent any surface agglomeration and reduce the space charge region, and thereby separate the electron hole pair. The choice of metals to be doped on the surface of titania depends on its ability to form a homogeneous surface metal oxide [Citation12–19].
Herein, nanocrystalline pure and vanadium-doped titania were synthesised via sol-gel process, and their photocatalytic activities were tested for the degradation of methylene blue (MB) at different pH values. The major objective of this study is to investigate the application of pure and V-doped titania for the removal of lead and zinc ions from aqueous solutions.
2. Material and methods
2.1. Materials
Titanium (IV) isopropoxide, hydrochloric acid, methanol, and ammonium metavanadate were acquired from Sigma-Aldrich and used without further purification. Deionised (DI) water was obtained from a Milli-Q water purification system (Millipore). All Pyrex glassware were washed with diluted nitric acid, soap, and DI water and dried in an oven at 75 °C.
2.2. Methods
2.2.1. Syntheses of pure and vanadium doped titania
Nanocrystalline titania was synthesised using sol-gel process wherein a mixture of 5 ml of titanium isopropoxide (99.99%) and 25 ml of methanol at 5 °C was subjected to continuous ultrasonication. Another mixture of 25 ml of methanol, 0.5 ml of HCl (1.3 M), and 0.5 ml of DI water was prepared and added drop wise to the first mixture of solvent and isopropoxide to prevent sudden formation of gel which may lead to incomplete reaction. The resulting sol was stirred for 1 h in an ultrasonic bath to prevent any agglomeration of the catalyst. The sol was dried in an oven for 12 h at 75 °C, followed by crushing and calcination at 450 °C for 4 h. A similar procedure was followed to prepare V-doped titania by replacing DI water with a solution of vanadium prepared by dissolving an appropriate amount of ammonium metavanadate in water. Different concentrations of vanadium solution were used to vary the amount of vanadium doped in titania, as shown in .
Table 1. Experimental conditions for the synthesis of V-doped titania.
2.2.2. Adsorption isotherm experiments
For MB degradation experiments, a stock solution of 100 ppm was prepared by dissolving 100 mg MB in DI water. Different concentrations ranging from 10 to 100 ppm were prepared from the stock solution, and the pH of the adsorbate solution was maintained at 4, 6, and 10 using standard buffer solutions. For each concentration, 100 ml solution was taken and divided into half (50 ml) in two conical flasks; 0.1 g of the catalyst was added to one of them while the other was left as a blank. Both the blank and the catalyst containing flasks were exposed to ultraviolet (UV) light (300 nm) for 24 h at 25 °C to ensure the equilibrium, and then the concentration of MB in all the samples was measured using an atomic absorption spectrophotometer. The difference between the concentrations of MB in the blank and the catalyst containing samples yielded the amount of MB removed by titania. A similar procedure was followed for the adsorption of Zn and Pb, where the stock solutions were prepared using zinc nitrate and lead nitrate, respectively, while all metals solutions were adjusted at pH of 2, based on several research articles suggesting optimum metals reductions at lower pH values [Citation20,Citation21]. All experiments were performed five times using the same catalyst at the same conditions for each measurement and the average was taken. Variations were found to be negligible (less than 6% maximum).
2.3. Characterisation
The microstructural analysis of the synthesised titania powders was performed using scanning electron microscopy (SEM; JOEL JSM-6460LU), and the particle size distribution was obtained using a particle size analyzer (PSA; Microtrac-Zetatrac, MICROMERTICS-S3500), where 0.05 wt% of the catalyst was ultrasonicated in a water bath for 15 min to obtain good dispersion. The amount of vanadium doped in titania was determined by energy-dispersive X-ray spectroscopy (EDS; OXFORD INCAx-SIGHT); its resolution at 5.9 keVis 137 eV. The surface area was measured using Brunauer, Emmett, and Teller technique (BET; MICROMERITICS-ASAP 2020) using liquid nitrogen. The crystalline phase and structure of the nanoparticles were characterised by X-ray diffraction (XRD).
3. Results and discussion
The synthesis of titanium dioxide takes place via two steps. First, the hydrolysis of titanium isopropoxide by acid takes place according to reaction (1):
(1)
(1)
The second step involves condensation of the produced hydroxides to form hydrated titanium dioxide as described by reaction (2):
(2)
(2)
The produced hydrated titanium dioxide is thermally treated to produce titania nanoparticles. The shape and the size of the catalyst depend mainly on the ratio of titanium to water; generally, a high water content leads to fast hydrolysis of titanium isopropoxide and hence, formation of large aggregates [Citation22].
3.1. Microstructural analysis
shows the SEM image of V-doped titania prepared at different Ti/H2Oratios. At a high Ti/H2O ratio (less amount of water), the catalyst particles are small and spherical. The particle size distribution is in the range of 3–11 nm as shown in . As the amount of water increased (), larger aggregates tend to form from smaller particles, known as Ostwald ripening, wherein coarsening and aggregation compete with nucleation during hydrolysis. The extent of this aggregation is a direct result of the Ti/H2O ratio; a higher ratio (>5) yields smaller particle size, and a smaller ratio may lead to combustion of residual hydrocarbonduring calcination process, resulting in the formation of coke in the final catalyst [Citation23].
BET analysis of pure and V-doped titania was carried out, and the results are shown in . The surface areas of pure and V-doped titania are similar (62.9 m2/g for pure titania and 66.1 m2/g for V-doped titania). This is because there is no separate growth of metal oxide on the surface of titania. Moreover, vanadium oxide is well dispersed on the surface, which was confirmed by the absence of any characteristic peaks of vanadium in the XRD patterns (discussed later). The results also indicated similar Langmuir surface areas and pore sizes. shows that the N2 adsorption–desorption isotherm of V-doped titania is of type IV, typical of a mesoporous catalyst.
Table 2. BET analysis results of pure and V-doped titania.
Titania exists in three phases, namely anatase, rutile, and brookite. Anatase is the most active phase and can be converted to rutile by thermal treatment at 500 °C. The XRD pattern of 1.7 wt% V-doped titania is shown in . Peaks corresponding to (101), (004), (200), (105), and (220) planes were observed at 2θ values of 25, 36, 48, 54, and 62°, respectively. The presence of (101) peak at 2θ of 25° (JCPDS 21-1272) confirms that the main phase of titania formed is anatase. The additional peaks indicate the presence of rutile phase of titania and crystalline (lattice) distortion caused by the incorporation of vanadium because of the difference in atomic radii of vanadium (134 pm) and titanium (147 pm) as stated by Pauling principle [Citation12,Citation16,Citation24].
The amount of vanadium doped on the surface of titania was measured using EDS, and the percentage of vanadium on the catalyst was verified using different initial concentrations of vanadium solution; the results are shown in . At concentrations higher than 4000 ppm, the amount of vanadium doped in titania tends to remain constant at 1.7 wt%, which is attributed to saturation of the titania surface with vanadium oxide formed by oxygen–metal bond. The EDS graph of V-doped titania prepared at 5000 ppm concentration, as shown in , shows the presence of minor amount of Cl originating from the HCl acid used in the synthesis process.
3.2. Photocatalytic degradation of MB
The effect of incorporation of vanadium into titania matrix on the degradation of MB is depicted in . It is evident that the doping of vanadium in titania increases the catalyst uptake significantly from 10 to 17 mg/g. This enhancement in the photocatalytic performance of titania can be attributed to the increment in the surface barrier which leads to less space in the charge region. The resulting large electric field makes the electron hole pair more separated and hence, more efficient.
shows the effect of different solution pH on the degradation of MB using V-doped titania. The catalyst uptake increased from 14 mg/g at pH 4 to 16 mg/g at pH 6 and 20 mg/g at pH 10. This is because at higher pH, dimerisation of MB may take place during the period of adsorption resulting in the reaction of negatively charged hydroxyl group with MB in solution. In addition, physical interaction may occur between the negatively charged catalyst surface and the nitrogen charge on MB [Citation25,Citation26].
3.3. Adsorption of Zn and Pb
The adsorption of Zn and Pb was studied using pure and V-doped titania under the same conditions to determine the metal uptake for both catalysts. The photocatalysis pathway involves mass transfer of metal ions to the surface of the catalyst, followed by adsorption of metal ions to active surface sites, where photocatalytic degradation can occur. At these sites, photo-generated electrons and holes can be utilised for the reduction of the metal ions. Degradation is followed by desorption of the degraded products, and finally mass transfer of the products away from the catalyst surface.
Doping can enhance the optical properties and photocatalytic activity of TiO2 by using band gap modifications to enhance absorption of light in Doping can also prevent electron-pair recombination and charge separation [Citation27].
The photocatalytic removal data for Zn and Pb are shown in and , respectively. The results indicate that pure titania adsorbs Pb more efficiently than Zn. For pure titania, a maximum uptake of 7 mg Zn2+/g of solid was obtained, whereas that of Pb was 17 mg Pb2+/g of solid. In the case of V-doped titania, the maximum uptake of Zn increased from 7 to 11 mg Zn2+/g of solid, and for Pb, the maximum uptake increased from 17 to 26 mg Pb2+/g of solid. At optimum conditions, the reaction constants calculated using the kinetic model developed by Mirghani et al. [Citation27] were 3.75 × 10-4 and 4.63 × 10-4 cm2/J for Zn and Pb respectively.
Similar trends were reported for photocatalytic removal of Cr(VI) using TiO2 doped with different transition metals. Removal efficiencies of Cr(VI) using Cu–TiO2 and Fe–TiO2 catalysts were 72.8% and 62.1%, respectively [Citation28].
There are many factors that can justify the preference of specific adsorbents toward some adsorbates than others. The tendency of titania to uptake more Pb than Zn can be explained by the ratio of charge to volume. Both Pb and Zn are amphoteric oxides. Pb exhibits oxidation states of +4 and +2, while Zn has oxidation states of +2 and +1. The atomic radii of Pb and Zn are 175 and 134 pm respectively. Hence, the higher oxidation state of Pb makes the surface of titania more attractive for Pb than Zn in this case. On the other hand, the electronegativity of Pb, which is 2.33 (Pauling scale) is higher than that of Zn (1.65 on Pauling scale); this makes the negatively charged surface of titania more attractive to Zn than Pb. However, the sum of these two factors (charge to volume ratio and electronegativity) makes titania more attractive for Pb than Zn, as indicated by the adsorption isotherms [Citation29,Citation30].
4. Conclusion
Pure and V-doped titania were synthesised via sol-gel process, and the microstructural analysis showed spherical shaped particles. The photocatalytic activity of the catalyst was tested for the degradation of MB at different pH values. It was found that the catalyst uptake increased as the pH of the solution increases. The effect of vanadium doping in titania was investigated by applying both pure and V-doped titania for the removal of Pb and Zn from aqueous solutions. The results revealed that the photocatalytic activity was enhanced when titania was doped with vanadium; the maximum Zn and Pb uptakes of the catalyst increased from 7 mg Zn2+/g for pure titania to 11 mg Zn2+/g for V-doped titania and from 17 mg Pb2+/g for pure titania to 26 mg Pb2+/g for V-doped titania, respectively.
Disclosure statement
No potential conflict of interest was reported by the author.
Additional information
Funding
References
- Li Y, Ma M, Wang X, et al. Preparation of cerium-doped titaniamacroporous films by a sol–gel spin coating using polypropylene glycol (PPG) as pore-creating agent: effects of Ce ions, PPG and calcination on photocatalytic activity. Surf Coat Technol. 2010;204(9–10):1353–1358.
- Trung T, Cho WJ, Ha CS. Preparation of TiO2 nanoparticles in glycerol-containing solutions. Mater Lett. 2003;57(18):2746–2750.
- Bhattacharyya K, Patra AK, Sastry PU, et al. Microstructural characterization of the V-doped nano-titania. J Alloys Compd. 2009;482(1–2):256–260.
- Li X, Yu J, Jaroniec M, et al. Cocatalysts for selective photoreduction of CO2 into solar fuels. Chem Rev. 2019;119(6):3962–4179.
- Doudou R, ZiZhan L, Yun HN, et al. Strongly coupled 2D-2D nanojunctions between P-doped Ni2S (Ni2SP) cocatalysts and CdS nanosheets for efficient photocatalytic H2 evolution. Chem Eng J. 2020;390:1385–8947.
- Dong S, Xin L, Changchang M, et al. Synthesized Z-scheme photocatalyst ZnO/g-C3N4 for enhanced photocatalytic reduction of CO2. New J Chem. 2020;44:16390.
- Shen R, Xie J, Xiang Q, et al. Ni-based photocatalytic H2-production cocatalysts. Chinese J Catal. 2019;40(3):240–288.
- Mengjie H, Muqing Q, Hui Y, et al. Recent advances on preparation and environmental applications of MOF-derived carbons in catalysis. Sci Tot Environ. 2021;760:0048–9697.
- Yue L, Hongwei P, Xiangxue W, et al. Zeolitic imidazolate framework-based nanomaterials for the capture of heavy metal ions and radionuclides: a review. Chem Eng J. 2021;406:1385–8947.
- Sai Z, Bingfeng L, Xiangxue W, et al. Recent developments of two-dimensional graphene-based composites in visible-light photocatalysis for eliminating persistent organic pollutants from wastewater. Chem Eng J. 2020;309:1385–8947.
- Li X, Yu J, Jaronie M. Hierarchical photocatalysts. Chem Soc Rev. 2016;45(9):2603–2636.
- Hamadanian M, Reisi-Vanani A, Majedi A. Preparation and characterization of S-doped TiO2 nanoparticles, effect of calcination temperature and evaluation of photocatalytic activity. Mater Chem Phys. 2009;116(2–3):376–382.
- Kaczmarek D, Domaradzki J, Prociow EL, et al. TiO2 thin films doped with Pd and Eu for optically and electrically active TOS–Si heterojunction. Opt Mater. 2009;31(9):1337–1339.
- Takeuchi M, Deguchi J, Hidaka M, et al. Enhancement of the photocatalytic reactivity of TiO2 nano-particles by a simple mechanical blending with hydrophobic mordenite (MOR) zeolite. Appl Catal B Environ. 2009;89(3–4):406–410.
- Zhang Z, Weng X, Gong K, et al. Photocatalytic activity of nitrogen doped nano-titanias and titanium nitride towards methylene blue decolouration. In: NSTI Nanotech, NSTI nanotechnology conference and trade show, technical proceedings, Part 1: Materials, fabrication, particles, and characterization; 2008 Jun 1–5. Boston, MA, US: Taylor & Francis Inc.; 2008. p. 680–683.
- Chen M, Ma CY, Mahmud T, et al. Hydrothermal synthesis of TiO2 nanoparticles: process modelling and experimental validation. In: Wu CY, Ge W, editors. Particulate materials: Synthesis, characterisation, processing and modelling. Great Britain: The Royal Society of Chemistry; 2011. p. 28–33.
- Einaga H, Futamura S, Ibusuki T. Photocatalytic decomposition of benzene over TiO2 in a humidified airstream. Phys Chem Chem Phys. 1999;1(20):4903–4908.
- Ishiguro H, Nakano R, Yao Y, et al. Photocatalytic inactivation of bacteriophages by TiO2-coated glass plates under low-intensity, long-wavelength UV irradiation. Photochem Photobiol Sci. 2011;10(11):1825–1829.
- Rengifo-Herrera JA, Pizzio LR, Blanco MN, et al. Photocatalytic discoloration of aqueous malachite green solutions by UV-illuminated TiO2 nanoparticles under air and nitrogen atmospheres: effects of counter-ions and pH. Photochem Photobiol Sci. 2011;10(1):29–34.
- Xin Z, Wen L, Zhipeng L, et al. Highly efficient enrichment mechanism of U(VI) and Eu(III) by covalent organic frameworks with intramolecular hydrogen-bonding from solutions. Appl Surf Sci. 2020;504:0169–4332.
- Xin Z, Zhipeng L, Wen L, et al. The magnetic covalent organic framework as a platform for high-performance extraction of Cr(VI) and bisphenol a from aqueous solution. J Hazard Mater. 2020;393:122353.
- Tian G, Pan K, Fu H, et al. Enhanced photocatalytic activity of S-doped TiO2-ZrO2 nanoparticles under visible-light irradiation. J Hazard Mater. 2009;166(2–3):939–944.
- Kalfa OM, Yalçınkaya Ö, Türker AR. Synthesis of nano B2O3/TiO2 composite material as a new solid phase extractor and its application to preconcentration and separation of cadmium. J Hazard Mater. 2009;166(1):455–461.
- Hu MZ, Lai P, Bhuiyan MS, et al. Synthesis and characterization of anodized titanium-oxide nanotube arrays. J Mater Sci. 2009;44(11):2820–2827.
- Al-Harahsheh M, Shawabkeh R, Al-Harahsheh A, et al. Surface modification and characterization of Jordanian kaolinite: application for lead removal from aqueous solutions. Appl Surf Sci. 2009;255(18):8098–8103.
- Shawabkeh RA. Hydrometallurgical extraction of zinc from Jordanian electric arc furnace dust. Hydrometallurgy. 2010;104(1):61–65.
- Mirghani M, Al-Mubaiyedh UA, Nasser MS, et al. Experimental study and modeling of photocatalytic reduction of Pb2+ by WO3/TiO2 nanoparticles. Sep Purif Technol. 2015;141:285–293.
- Zhang J, Fu D, Wang S, et al. Photocatalytic removal of chromium(VI) and sulfite using transition metal (Cu, Fe, Zn) doped TiO2 driven by visible light: feasibility, mechanism and kinetics. J Ind Eng Chem. 2019;80:23–32.
- Shawabkeh R, Al-Harahsheh A, Hami M, et al. Conversion of oil shale ash into zeolite for cadmium and lead removal from wastewater. Fuel. 2004;83(7–8):981–985.
- Shawabkeh RA, Abu-Nameh ESM. Absorption of phenol and methylene blue by activated carbon from pecan shells. Colloid J. 2007;69(3):355–359.