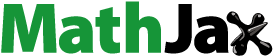
Abstract
Nickel oxide-zinc oxide (NiO-ZnO) nano-catalyst was embedded in a carbohydrate polymer (Alg/NiO-ZnO) was successfully synthesized and used for the (photo)catalytic conversion of deleterious contaminants such as organic dyes (Acridine Orange ‘ArO’, Methylene Blue ‘MB’, Methyl Orange ‘MO’) and 4-Nitrophenol ‘4-NP’ as well. Solar light helped photo-degradation and NaBH4-assisted reduction of organic dyes, as water pollutants, were studied using Alg/NiO-ZnO. Furthermore, utilizing Na alg/NiO-ZnO as a catalyst for the photocatalytic degradation was found to be more efficient for ArO, however catalytic reduction of MB was the best among all contaminants. Scanning electron microscopy (SEM), energy dispersive spectrometry (EDS), X-Ray Diffractometry (XRD) and Fourier Transform Infrared Spectroscopy (FTIR) techniques were used to identify the shape and structure of Alg/NiO-ZnO. To gather the data, an ultraviolet-visible (UV) spectrophotometer is employed. Data collection and processing, graph creation, and analysis were all accomplished with Origin 2018. In just 13 minutes, the reduction percentage of MB was determined to be 95.25%, while ArO underwent photocatalytic degradation up to 77.5% in 150 minutes. Several factors are used to optimize the catalytic property of Alg/NiO-ZnO in the reduction of organic dyes. Alg/NiO-ZnO thus provides a promising technique for the efficient oxidation and reduction of pollutants from industrial effluents.
1. Introduction
Water occupies around 70% of the Earth’s atmosphere. Approximately 97% (1.414 × 1024 g) of the total mass of water on Earth is saline, which could neither be drinkable nor good for agricultural or industrial purposes. Only 3% of the water on earth is freshwater, and by looking closely, about 30% and 69% of the freshwater are underground and water in glaciers and ice caps respectively. Massive sizes of water are surrounded by the deepest reaches of Earth. Freshwater supply available for usage is less than 1%, found in rivers, lakes, ponds, etc. [Citation1–4]. Water supplies are significantly strained as a result of technological advancements and growing industrialization [Citation5, Citation6].
The prompt rise of the human population has led to significant pollution of the water, air, and soil. By the middle of the twenty-first century, the world’s population is expected to rise from around 7.5 billion to a terrifying 9 billion [Citation7, Citation8]. Water is the most extraordinary molecule on the planet, despite its common appearance. Its small size, on the other hand, hides the intricacy of its chemical properties, which are suitable for carbon-based life [Citation9]. Human prosperity and growth are gravely threatened by the toxic and barely biodegradable organic and inorganic contaminants that have been introduced to the water supply [Citation10]. Removal of pollutants from water has become extremely important because it is one of the most important components on the planet for both living beings and aquatic species [Citation11]. It is extremely desirable and related to the sustainability of our world to be able to cleanse wastewater, especially industrial effluent, to a dischargeable or reusable quality [Citation12].
Nanomaterials (NMs) are, generally, materials whose sizes are around 1 to 100 nanometers (in one dimension at least) [Citation13–15]. The nanoscale size of NMs is responsible for their considerably high-quality properties (optical, electrical, mechanical, and magnetic), a plethora of exceptionally superb characteristics (large surface area, high capacity of adsorption, high reactivity, adaptable molecular size, ease of separation, regeneration and recyclability, perfect catalytic activity, and high porosity) as well as differing from classical materials splendidly [Citation15–18]. In terms of sorption behaviors, magnetic activity, and chemical reduction, metal oxide nanoparticles have distinctive features [Citation19–21]. A variety of nanoparticles (NPs) including carbon-based, metal/metal oxide, and polymeric NPs, can be dispersed in a polymer matrix to create blended scaffolds with better characteristics. Compared to rudimentary polymer platforms, hybrid scaffolds with nano-fillers offer customized functionality and promising physical, chemical, electrical, and biological features [Citation22, Citation23].
Natural polymer-based active sorbents are becoming more progressively marketable. Alginate is one of these polymers, which has biodegradability, resorb-ability, no carcinogenicity, and has been proven to be toxicity-free [Citation24, Citation25]. Diverse alginate bio-composites have been used in the elimination of impurities in the textile (containing several toxic and carcinogenic dyes) and agricultural industries [Citation11, Citation19, Citation26–29]. The physicochemical properties of alginate-based NMs have been extensively reviewed for various chemical and biological uses [Citation30].
Freshly, there has been considerable evolution in the betterment of hydrogel composites and their implementation in multiple frameworks including environmental decontamination. Hydrogels incorporated with NPs, which reinforce the mechanical features of the alginate, appear more competent. Over the past 30 years, photocatalytic degradation of toxic matter to the environment by transforming solar energy into chemicals has been a growing concern [Citation31]. Heterogeneous photo-catalysts have plenty of merits over other photo-catalysts, for they can be economically feasible, stable, nontoxic, and powerful oxidizing and reducing agents at ambient temperature and pressure [Citation32].
Several semiconductors with enhanced photocatalytic activity in visible light regions have been created and characterized. These can be used in practical applications by using semiconductors with smaller band gaps or by doping semiconductors with wide band gap energy with other metal oxides to trap charge carriers [Citation33].
Chemists and scientists would offer innovative NMs (Alginate/metal oxide nanocomposites) that were discovered to be potent and highly effective nano-catalysts for the remediation of water and wastewater pollution to guarantee a cost-effective solution to solve issues of water contamination [Citation34, Citation35]. There are several techniques for surface modification and material preparation in heterogeneous catalysis. We used the wet-impregnation procedure on one of them. It is the best and most straightforward way to prepare assisted catalysts. This method used a variety of techniques, such as surface OH group modification and adsorption, to support the fixed amount of catalyst precursor [Citation36].
The effectiveness of Na alg/NiO-ZnO as a catalyst for the reduction and solar-assisted photodegradation of organic pollutants was examined using various catalyst amounts, dye types, reaction times and reducing agent dosages at room temperature. The best catalyst for the photodegradation of ArO under solar light was found to be Na alg/NiO-ZnO, which was also proven to be an excellent catalyst for MB reduction when combined with NaBH4.
2. Experimental section
2.1. Materials and methods
ArO, MB, MO, 4-NP, sodium hydroxide, hydrochloric acid, and zinc (II) chloride were purchased from Sigma-Aldrich Chemical Company in the United States. Nickel (II) acetate tetra-hydrate was brought from Germany – Merck. Sodium Borohydride (97%), and Sodium Alginate were obtained from Loba Chemie, Mumbai, India. All these materials were used without any purification. Distilled water (DW) was used in the preparation of all solutions. All reagents used are analytical and spectral-grade pure.
2.2. Synthesis of nickel oxide-zinc oxide nanocomposite
In a 500-mL beaker, 100 mL of DW was placed. Using a piece of aluminum foil, a spatula, and a sensitive balance, 2.4900 g of nickel (II) acetate tetra-hydrate powder and 1.3678 g of zinc (II) chloride powder were weighed and added to the DW. Similarly, 1.9966 g of sodium hydroxide pellets were weighed and placed in a 500 mL volumetric flask, shaken well, and completed to the mark with DW. Nickel (II) acetate tetra-hydrate and zinc (II) chloride solutions were mixed, and some drops of sodium hydroxide solution were added until the solution become alkaline . (the nanoparticles start falling down at the bottom of the beaker as the pH of the solution approached 10, allowing the precipitation), heated up to 60 °C with stirring for 24 h. Then the solution was atmospherically well-dried at room temperature by placing it in a pottery dish for 2 days, collected, and put inside the muffle for calcination at 500 °C. The metal oxide nanocomposites were ready for use. All solutions prepared were 0.1 Molar before they were mixed together.
2.3. Preparation of sodium alginate/nickel oxide-zinc oxide nanocomposites
Metal oxide nano-catalysts were dispersed in sodium alginate solution and the catalyst was then chemically wrapped up with alginate hydrogel by crosslinking the alginate by adding concentrated hydrochloric acid. Then the gelled mixture was washed using plenty of DW for 4–5 min to wash up the extra hydrochloric acid. After washing, the catalyst is ready for use.
2.4. Characterization
A few milligrams of the metal oxide nano-catalyst NiO-ZnO (powder), Alginate/metal oxide nanocomposite (solid gel), and sodium alginate (hydrogel) were placed in three small plastic vials separately, labeled and sent for analysis. Results were successfully collected, received, analyzed, and reported in the coming sections.
2.4.1. Structure characterization and apparatus
2.4.1.1. FESEM
The size and surface morphology were investigated using a scanning electron microscope (SEM, JSM-6701F, JEOL), after covering the samples with gold films. SEM images with their congruent elemental mapping of the material were obtained at distinct magnifications (1000x, 750x, etc.) shown in .
Figure 1. SEM Images of (a) NiO-ZnO, (b) Na alg/NiO-ZnO, and (c) pure sodium alginate (hydrogel) for lower resolutions and (a′), (b′) and (c′) for higher resolutions respectively.
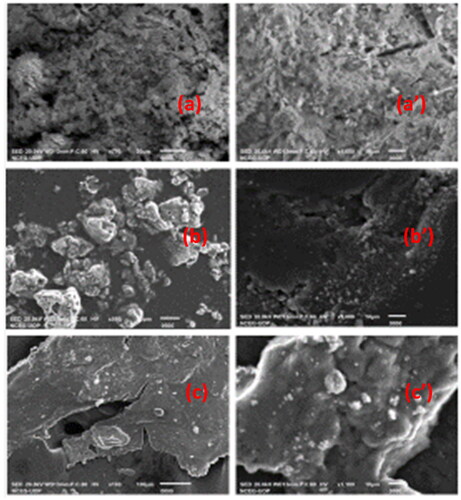
Results obtained from images taken using FESEM technique showed that the NiO-ZnO nanoparicles has grown in huge quantities and have irregular surfaces with grooves () and its alginate composite (NiO-ZnO-embedded in alginate hydrogel) has an irregular rough surface (), in both magnifications. () shows that alginate surface structure is composed of quite flat sheets with larger grooves, as can be well seen in the image with higher magnification depicted in . confirms well-dispersed NiO-ZnO in the hydrogel matrix.
Since it has been mentioned above in the literature that porosity is, at least, one of the most important features for a material to be a good catalyst, these results showed that this alginate hydrogel has to be an excellent catalyst for the removal of organic pollutants and other contaminants as well. SEM analysis depicted the metal oxide composite nanostructure; it gave crystal clear evidence about the formation of NiO-ZnO at the nano-level.
2.4.1.2. EDS
EDS was used to determine the elemental compositions of the materials. To confirm the formation of the NiO-ZnO composite, energy dispersive spectrometric analysis was performed. shows that nickel and zinc together compose (70.5 mass %), exactly (41.89 mass %) nickel, and (28.61 mass %) zinc whereas oxygen content is only (12.76 mass %). Carbon content is (30.97 mass %) by mass and (56.10 mass %) for oxygen, which collectively constitute a mass % of 87.07 of the total mass of alginate hydrogel (). The analysis also affirms the presence of sodium in the polymer structure in () down below. The nanocomposite material (Na alg/NiO-ZnO) contains 21.21% by mass nickel and about (mass % 5.5) zinc, the above-mentioned percentages were dramatically dropped to (19.42% and 44.10% by mass) for carbon and oxygen respectively due to the addition of nickel and zinc oxides (as NiO-ZnO) to alginate hydrogel. The actual content of the carbohydrate polymer is 6% by weight, which is prepared by dissolving 6 grams of sodium alginate powder in 100 mL of DW, well shaken and stirred.
A resolution of 1280*960 pixels for all (a), (b), and (c) was used, while the magnifications were different which were 330x, 370x, and 550x for (a), (b), and (c) respectively as illustrated in .
2.4.1.3. FTIR
FTIR chromatograms of NiO-ZnO and Na alg/NiO-ZnO were determined for the qualitative analysis and used to confirm the presence or absence of certain bonds and functional groups e.g. A broad intense absorption stretching band is due to the formation of metal oxide (Ni = O and Zn = O) double bonds at around 500 cm−1 as can be clearly seen in . Absorption stretching bands with a shoulder at 920, 1050, (around 1250 and 1635) cm−1 give rise to the precursor-related band (in both spectra), C-O, C-C single bonds, and COO− (symmetric and asymmetric stretching vibrations) in the Na alg/NiO-ZnO spectrum respectively, while the broadband stretching vibration in the Na alg/NiO-ZnO spectrum at between 2850 cm−1 and 3450 cm−1 appears due to O-H groups of the cross-linked oxides with hydronium ion of the acid, free O-H groups of alginate and C-H bond stretch overlap.
2.4.1.4. UV
The UV spectra were recorded on (Thermo Scientific Evolution 300 UV-visible spectrophotometer, Waltham, MA, USA) at wavelengths between (200 and 600) nm for both ArO and MO; and (200–700), (200–800) nm for 4-NP and MB respectively. 4-NP, ArO, MO, and MB solutions were used as standards to collect their respective base peaks.
2.4.1.5. XRD
By using (XRD) spectroscopy, the structural characteristics, phase purity and crystallinity of the produced alginate hydrogel and NiO-ZnO nanocomposite were evaluated. Ultima IV X-ray diffractometer (Kuraray Co. Ltd. N KYOWAGLAS-XA), made in Japan, was used to perform this experiment. The scan speed used is 2.0000°/min. whereas the scan range for alginate hydrogel and NiO-ZnO nanocomposite XRD experiments were 15.0000° − 50.0000° and 10.000° − 85.0000° respectively. The obtained XRD spectra are given in . The hump between 15.0000° and 35.0000° in proves the amorphous structure of the pure alginate hydrogel. The synthesized NiO-ZnO nanocomposite’s crystallinity was demonstrated by the acquired XRD spectrum in . Peaks at 37.0000°, 43.0000°, 63.0000°, 75.0000°, and 79.0000° are due to the presence of nickel oxide in the hybrid structure of the metal oxide composite material. On the other hand, zinc oxide gives rise to peaks at 31.0000°, 34.0000°, 47.0000°, 56.00000°, 66.0000°, 68.0000°, and 69.0000° ().
2.5. Monitoring the photo-catalytic degradation reaction
In a 2 L Erlenmeyer volumetric flask, 37.18 mg of organic dye (ArO) powder was taken using a sensitive balance and filled up to the mark with DW. And in a similar manner, two organic dyes (MB, MO) and 4-NP were prepared. In a 250 mL beaker, 10 mL of 0.07 mM ArO and 10.10 mg of nano-catalyst were taken using a pipette and a sensitive balance respectively. Then the reactants were put under single beam solar light and after 15, 30, 60, 90, 120, 150, 180 minutes, et cetera, portions of the solution were taken into a quartz cell and the degradation extent of the organic dye with time was determined. A Thermo Scientific Evolution 300 UV-Visible Spectrophotometer was used, and the results were recorded. A graph for each sample was drawn. Graphs were compared and an analysis of data was carried out. The molar concentration of all three organic dyes was the same (0.07 mM), while the Molarity of 4-NP prepared for this study was 0.13 mM. The typical reaction is repeated twice more with different catalyst amounts, that is, 20 and 30 mg.
2.6. Monitoring the catalytic reduction of organic dyes and 4-NP
A 25-mL volumetric flask was well washed, on average 56.88 mg of was placed in it and about 15 mL of DW was added, and the solution dissolved immediately. Notice that the sodium borohydride used should be freshly prepared; otherwise, it would not potentially work and its concentration could have been altered.
Using a pipette, 2.5 mL of each of organic dye and 4-NP were – separately – taken into a quartz cuvette, the UV-Vis. The spectrophotometer was run then the chromatogram was obtained, 0.5 mL of 0.1 M was added, UV-visible Spectrophotometer was run and, 10.00 mg of the catalyst encapsulated with alginate hydrogel was added, then the spectra were recorded every minute for no more than 20 min. The reduction percentage (%R) can be calculated using the equation:
(1)
(1)
Where C0 and Ct are the initial and final concentrations respectively [Citation4, Citation9, Citation37].
For real sample analysis, a total of three samples were collected from seawater, well water, and sterile water for irrigation and were examined against MB using (0.1 M) NaBH4. All experiments were performed at rt. The same reaction has been carried out by varying the amount of the nanocomposite catalyst using 20 and 30 mg for each run.
3. Results and discussion
3.1. Choice of materials
Porous hydrogels with high porosity, variable density, and swells in an aqueous environment are viable alternatives as floatable materials that can be separated and recovered efficiently and easily. Alginate-wrapped metal oxide nanocomposites are excellent nano-catalysts for the purpose of eradicating these harmful dyes and eliminating them from water. This fact has been mentioned in several earlier research articles some of which have been reviewed in the literature. For these reasons alg/NiO-ZnO would be an exquisite choice [Citation38–40]. Therefore, NiO-ZnO was dispersed in sodium alginate solution and cross-linked by adding a few drops of concentrated HCl which is a frequently used crosslinking agent for the polymers bearing carboxylate groups. The degree of interaction between carboxyl or carboxylate groups and H+ ions define the solidity of the gel matrix [Citation41].
Na alg/NiO-ZnO was applied for the oxidation and reduction of organic dyes and pigments which are among the most common contributors to water pollution in industries such as textiles, leather, paper, cosmetics, paint, and printing. Textile industrial dyestuffs, in particular, comprise various harmful organic components and are a substantial contributor (20%) to total water pollution among paints, food dyes, food colorants, and other chemicals. The majority (>50%) of all industrial organic water pollutants are organic dyes/pollutants containing naphthalene or benzene rings [Citation42].
Oxidation was performed under solar light while the reduction was carried out in the presence of NaBH4. NaBH4 (Redox Potential = −1.24 V in aqueous medium) is a moderate but also a good reducing agent for the reduction of organic compounds such as aldehydes, ketones, and organic colorants/dyes [Citation43].
3.2. Removal of organic pollutants
3.2.1. Photocatalytic degradation
In the industrial sector, over 10,000 different kinds of dyes are utilized for diversified purposes. These dyes are produced on a global scale of around 30,000 tons per year. Most of these dyes have a complex chemical structure that makes them resistant to degradation and allows them to persist in the environment for long periods of time, making it recondite for light to penetrate the water and disrupt aquatic flora’s normal photosynthetic potential (Animals are also negatively affected in the same way) [Citation33, Citation44, Citation45].
Photo-catalysis is a key mechanism in dye effluent treatment, in which irradiated electrons are stimulated from the valence band to the conduction band, resulting in the production of electron-hole pairs. The produced hydroxyl radical is a powerful oxidizing agent that totally converts the dye into non-hazardous compounds (e.g., CO2, H2O, etc.) [Citation45, Citation46].
This research additionally investigated the photochemical degradation mechanism. The majority of them, on the other hand, have focused on the increased generation of hydroxyl radicals or holes and their functions in the breakdown of organic contaminants [Citation47]. The photocatalytic activity of these semiconductors could be explained by their higher electronic band gaps, which could be responsible for the photo-conversion effect in the given photoactive material. Furthermore, by adding some dopant material to the band gap, the band gap may be modified according to the need and accessibility, allowing for more donor/acceptor levels, and thereby facilitating electron flow [Citation48, Citation49]. The photocatalytic activity of the synthesized sample was analyzed by solar light to degrade ArO dye.
3.2.1.1. Degradation mechanism
Na alg/NiO-ZnO is first responsible for photon absorption because it excites electrons from the valence band to the conduction band and creates a positive hole in the valence band.
A beam of solar light (hv) hit up the catalyst where the photon of light was absorbed by the catalyst and by which irradiated electrons of the catalyst are stimulated from the valence band to the conduction band, resulting in the production of electron-hole pairs. The irradiated electrons in the conduction band convert oxygen molecules to the hydroperoxy (.OOH) free radicals and these free radicals can spontaneously attack the organic dye molecules and degrade them, as illustrated in . While electron-hole pairs produce hydroxyl radicals from water molecules which are powerful oxidizing agent that would totally convert the dye into non-hazardous compounds.
3.2.1.2. Selectivity towards organic dyes
To study the oxidation (also known as photocatalytic degradation) of some organic dyes, diluted aqueous solutions of 7*10−5 M ArO and MB were prepared, and, each time, 30 mg of the nanocomposite (NiO-ZnO embedded in alginate hydrogel) was used. By using UV-Vis. spectrophotometer, the photocatalytic degradation of ArO (at around λmax 485 nm) and MB (at λmax 665 nm) under solar energy was measured. At the beginning, the catalyst reactivity with both organic dyes showed similar photocatalytic activity even though the performance of the catalyst in the reaction with ArO was better and this can be simply noticed as shown in .
3.2.1.3. Effect of varying the catalyst amount
In this study, various conditions such as varying the amount of the catalyst, reusability as well as contact time are checked and thoroughly studied. As shown in ArO appeared to be far better than MB in the photocatalytic degradation (Degradation percentage, %D, is 77.49% and 3.83% in 150 min for both ArO and MB).
Out of three different amounts of the catalyst used 10, 20, 30, and 20 mg stood out and gave the best results (%D = 59.80, 77.66, and 61.00% respectively). Starting the reaction, adsorption occurred (up to 33%) in all three separate cases, then degradation of the organic dye ArO proceeded ().
Figure 7. UV-Visible absorption spectra show the different amounts of the catalyst that are being used. (a) compares the photo-degradation efficiencies of the three amounts, while (b), (c), and (d) for the catalyst amounts 30, 20, and 10 mg respectively.
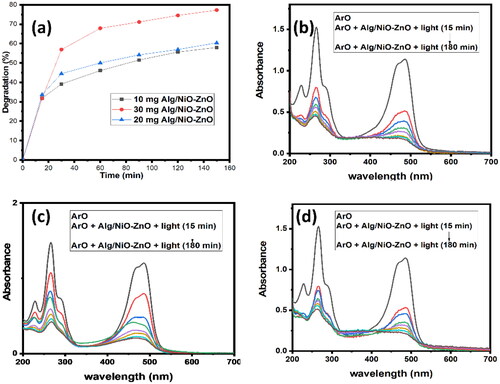
These graphs explain three different amounts (10, 20, and 30 mg) of the catalyst that were being used during the process of ArO photo-degradation.
Both reactions followed Pseudo-First Order and the rates of both reactions were calculated using the formula [Citation39, Citation50]:
(2)
(2)
Simply by plotting (At/A0) vs removal time t. The photocatalytic efficiency of the catalyst was determined from the UV-Visible spectrum by applying the following equation:
Degradation % = 100 – (At * 100/A0) [Citation50].or
Degradation efficiency (%D) = ((A0 – At)/At) * 100 [Citation51].
A0 is the initial absorbance and At is the absorbance at various time intervals.
The photo-degradation rate constant, k, can be computed using the linear relationship of ArO dye degradation, in which At and A0 are the corresponding absorbance values of the ArO dye solution at time equals t and 0 respectively, and k is the pseudo-first-order rate constant.
The absorbance change (At/A0) of the ArO solution appears to be directly proportional to concentration change (Ct/C0) during the photocatalytic process, according to the Beer–Lambert law [Citation39, Citation52].
3.2.1.4. Reusability of the catalyst
The nanocatalyst, Sodium alg/NiO-ZnO pellet, was reused up to two consecutive times for this reaction with a low decline in its efficacy. The removal percentage is 77.5% and 74.4% for the 1st and 2nd runs successively. The catalyst can be easily taken from the reaction mixture, washed with DW, and then re-used twice ().
Figure 8. UV-Visible absorption spectra for the reusability of the nano-catalyst. Graph (a) shows the reusability of the catalyst in the degradation of ArO for two successive cycles.
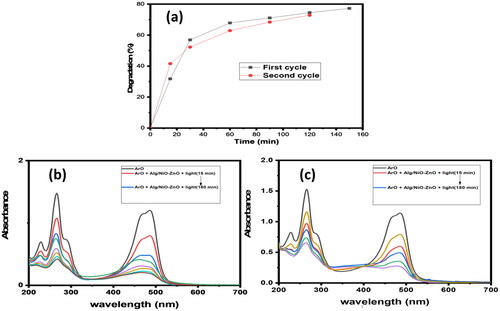
Figure 9. ESI mass spectra of the N-de-methylated intermediates that were isolated using the HPLC-ESI-MS method and produced during the photodegradation of the AO dye ( is regenerated from a paper published by Lu C, catalysts 2013).
Abbreviations:
ESI-MS = electrospray ionization mass spectrometry, HPLC = high performance liquid chromatography, AO or ArO = acridine orange dye, AO-M = N-de-monomethyl acridine orange, AO-MM = N,N′-de-dimethyl acridine orange, AO-D = N,N-de-dimethyl acridine orange, AO-DM = N,N,N′-de-trimethyl acridine orange, AO-DD = N,N,N′,N′-de-tetramethyl acridine orange.
A total of 6 compounds (including AO) have been detected, all of which are derived from the parent AO.
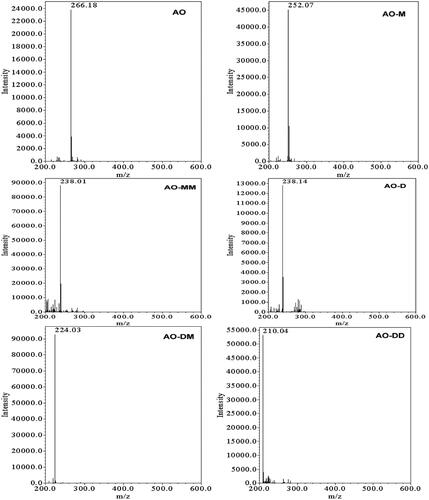
Equation 1: The following chemical equation explains the degradation of Acridine Orange dye by Alg/NiO-ZnO nano-catalyst in the presence of light (hv), and as a result, the degradation products would be less harmful.
3.2.1.5. Fragment identification
A mass spectrometer would help to identify the fragments that are produced from the above reaction by giving mass spectral data of the light-assisted degradation of ArO. Using the HPLC-ESI-MS technique, the N-de-methylated intermediates were further verified. It seems that the intermediates’ acid forms contained the molecular ion peaks. The N-de-methylated intermediates’ mass peaks determined by interpreting their mass spectra are shown in . We verified that the component 1, m/z = 266.18, in the liquid chromatogram was ArO based on the mass spectrum analysis results. Other components were N-de-mono-methyl-acridine orange (2, m/z = 252.07); N,N-de-dimethyl-acridine orange (3, m/z = 238.01); N,N′-de-dimethyl-acridine orange (4, m/z = 238.14); N,N,N′-de-trimethyl-acridine orange (5, m/z = 224.03) and N,N,N′,N′-de-tetramethyl-acridine orange (6, m/z = 210.04). The sequential N-de-methylation of maternal ArO is shown by these peaks, which differ exactly by 14 mass units in succession [46, Citation53].
3.2.2. Catalytic reduction
The utility of metal oxide nanoparticles as nano-catalysts could surely be confirmed, especially when it comes to the reduction of some organic dyes in wastewater such as MB, ArO, etc., for the fact that these metal oxide nanocomposites have many extraordinary features that strongly qualify them in the list of dynamic catalysts, e.g. improved specific surface area makes interactions between the material and dyes easier and other above-mentioned characteristics [Citation47].
Methylene blue is a heterocyclic aromatic compound that contains a thiazine ring, a cationic dye that is used in the textile and medical industries. It is an effective drug as an antimalarial and a potent remedy for methemoglobinemia.
Na alg/NiO-ZnO was added to the mixture in varying amounts (10, 20, 30 mg), and the UV-visible spectrum was collected at various intervals. On the basis of the study, the process was optimized for the reduction of the most selective dye by examining the effects of several factors, including catalyst quantity, reducing agent amount, and reusability.
Equation 2: The following chemical equation displays the NaBH4-assisted catalytic reduction of MB.
MB has a maximal UV-visible spectrum peak at about 665 nm, and reduced methylene blue, often known as leucomethylene blue, is colorless. Because MB is carcinogenic and mutagenic to living organisms, it is hazardous in natural water, and its accumulation in the human body can be terribly dangerous [Citation48, Citation49] ().
Figure 10. Schematic representation outlines the decolorization process of MB in presence of sodium borohydride using the Na alg/NiO-ZnO nanocomposite as a catalyst.
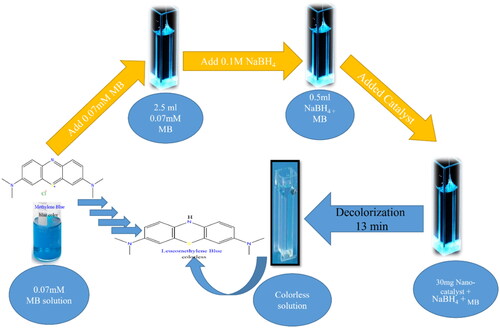
3.2.2.1. Selectivity of organic dyes
The same reaction was carried out for catalyzed reduction of 4-NP, ArO, MB, and MO using as a reducing agent. The results in showed that the reduction of MB dye, using the afore-mentioned method and technology, has been completed and provided the highest removal percentage (%R) compared with the remaining pollutants under study. This means that NaBH4-assisted catalytic reduction making use of Na alg/NiO-ZnO as a nanocomposite is more possible and convenient to remove MB from the water this way.
Figure 11. This graph outlines selectivity upon reduction (%R) as a function of time of four contaminants 4-NP, AO, MB, and MO.
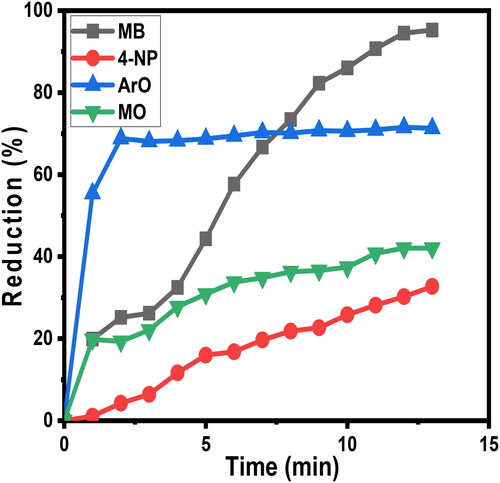
By looking at , it is clear that MB curve is nearly steadily progressive and complete (reduction % > 95%). Photo-oxidation reaction of ArO dye exhibited an excellent removal rate (about 70%) in the first 60 minutes, then plateaued, see . The decline in absorption at the wavelength of the dye molecules’ absorbance maximum would be observed as the catalytic reduction of dyes progresses [Citation54, Citation55].
Figure 12. UV-Visible absorption spectra explain the selectivity upon four different organic dyes. These graphs (a), (b), (c) and (d) for UV-Visible spectra of MB, MO, 4-NP and ArO respectively.
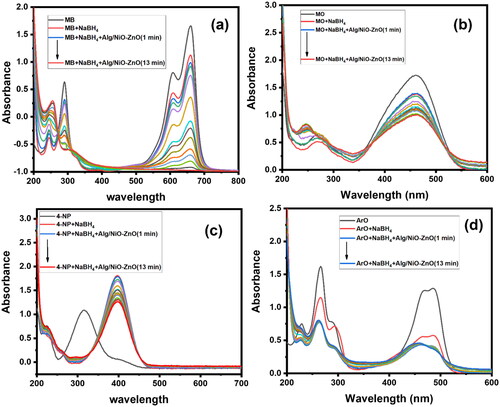
3.2.2.2. Effect of varying the amount of the catalyst
In this work, distinct amounts of the metal oxide nano-catalyst (10, 20, 30 mg) have been used and the reaction responses were tracked. The ideal amount for the catalytic reduction of different organic dyes is displayed down below:
Out of three different doses of catalyst employed, (10, 20, and 30 mg) the results obtained when using 30 mg were consistently prime (removal % = 70.92%, 74.66%, and 95.25% respectively).
By adding NiO-ZnO embedded in alginate hydrogel to the dye solutions, ultimate fading and de-coloration of MB solutions occurred, as it is obviously seen in the progressive decline of the dye λmax absorbance value with time in rt.
3.2.2.3. Recyclability of the catalyst
In this experiment, the same nano-catalyst was easily removed, washed, and re-used thrice. This study has proved a very powerful technique in the use of sodium alg/NiO-ZnO as a novel nano-composite material (in the presence of 0.1 M Sodium Borohydride as the reducing agent) for the elimination of some of the organic compounds such as MB and some other organic dyes. In the first cycle, the removal percentage (%R) is up to 95.25. Although the results for the 2nd and 3rd cycles were almost never altered, there is still a huge efficiency gap (42.3% drop) in comparison with the first run, with only 54.99 and 54.5% of MB reduced for the 2nd and 3rd cycles, respectively. The decline in the catalytic reduction efficiency would be due to the fact that the nanocatalyst would have been affected by a combination of factors, including catalyst poisoning, sintering, surface contamination, and inhibition (reaction products may partially block the catalyst active sites). The removal rate from time = 0 up to the 6th minute is similar among the three cycles; while the first session curve has sharply peaked, the 2nd and 3rd curves plateaued after a tiny increase starting at the 6th minute. The removal rate in the first cycle is much faster (the removal percentage is slightly greater than 95% in 13 minutes).
The catalytic activity of the nano-composite material decreased, and that is probably due to the fact that some of the material has been lost by washing, and not only for this reason but rather because it has become partly saturated with organic contaminants.
4. Conclusion
Photocatalytic degradation and catalytic reduction of some organic dyes in wastewater have been studied. For the oxidation and reduction of organic dyes, sodium alg/NiO-ZnO was used as an effective catalyst. According to this study, these findings confirmed that the utilization of metal oxide nanomaterials wrapped up with alginate hydrogel is much better than the traditional methods for water treatment, some of which have been reviewed in the introductory section above. Sodium alg/NiO-ZnO can be recovered and used successively twice, with only 4% less photocatalytic activity against ArO. Also, the nano-catalyst used for the catalytic reduction of MB emerged as a substantially successful catalyst for this purpose and it is recoverable and can be recycled and reused for three successive cycles. The catalyst could be an exquisite choice for the reduction and degradation of organic dyes and therefore positively affect the environmental safety of diverse water-based setups.
Acknowledgement
Both authors acknowledged the assistantship that has been received from Professor Abdullah M. Asiri of Center of Excellence for Advanced Materials Research, King Abdulaziz University, P. O. Box 80203, Jeddah 21589, Saudi Arabia, for reviewing and helpful revision of the manuscript, and the supervision that he has been providing throughout the lab work. Also, our sincerest gratitude extends to the reviewers for their valuable comments, which helped improve the quality of this paper.
Disclosure statement
The authors declare that they have no conflict of interests.
Data availability statement
Not applicable
References
- Drinkwater M, Kerr Y, Font J, et al. Exploring the water cycle of the blue planet. The Soil Moisture and Ocean Salinity mission. ESA Bull-Eur Space; 2009.
- Elgarahy AM, Elwakeel KZ, Akhdhar A, et al. Recent advances in greenly synthesized nanoengineered materials for water/wastewater remediation: an overview. Nanotechnol. Environ. Eng. 2021;6(1):1–24. doi: 10.1007/s41204-021-00104-5.
- Nestola F, Smyth JR. Diamonds and water in the deep Earth: a new scenario. Int Geol Rev. 2016;58(3):263–276. doi: 10.1080/00206814.2015.1056758.
- Pashaei-Fakhri S, Peighambardoust SJ, Foroutan R, et al. Chemosphere crystal violet dye sorption over acrylamide/graphene oxide bonded sodium alginate nanocomposite hydrogel. Chemosphere. 2021;270:129419. doi: 10.1016/j.chemosphere.2020.129419.
- Jayalakshmi R, Jeyanthi J. Dynamic modelling of alginate-cobalt ferrite nanocomposite for removal of binary dyes from textile effluent. J Environ Chem Eng. 2021;9(1):104924. doi: 10.1016/j.jece.2020.104924.
- Omer AM, Tamer TM, Abou-Taleb WM, et al. Development of iron oxide nanoparticles using alginate hydrogel template for chromium (VI) ions removal. DWT. 2020;175:229–243. doi: 10.5004/dwt.2020.24916.
- McDonald RI, Green P, Balk D, et al. Urban growth, climate change, and freshwater availability. Proc Natl Acad Sci U S A. 2011;108(15):6312–6317. doi: 10.1073/pnas.1011615108.
- Baruah S, Najam M, Joydeep K. Perspectives and applications of nanotechnology in water treatment. Environ Chem Lett. 2016;14(1):1–14. doi: 10.1007/s10311-015-0542-2.
- Bakhsh EM, Akhtar K, Fagieh TM, et al. International Journal of Biological Macromolecules Development of alginate @ tin oxide – cobalt oxide nanocomposite based catalyst for the treatment of wastewater. Int J Biol Macromol. 2021;187:386–398. doi: 10.1016/j.ijbiomac.2021.07.100.
- Opoku F, Kiarii EM, Govender PP, et al. Metal oxide polymer nanocomposites in water treatments. London (UK): IntechOpen; 2017, vol. 8.
- Goikuria U. Effect of metal-oxide nanoparticle presence and alginate cross-linking on cellulose nanocrystal-based aerogels. J App Pol Sci. 2021. doi: 10.1002/app.50639.
- Gade R, Ahemed J, Yanapu KL, et al. Photodegradation of organic dyes and industrial wastewater in the presence of layer-type perovskite materials under visible light irradiation. J Environ Chem Eng. 2018;6(4):4504–4513. doi: 10.1016/j.jece.2018.06.057.
- Buzea C, Pacheco II, Robbie K. Nanomaterials and nanoparticles: sources and toxicity. Biointerphases. 2007;2(4):MR17–71. doi: 10.1116/1.2815690.
- Pachapur VL, Larios AD, Cledón M, et al. Behavior and characterization of titanium dioxide and silver nanoparticles in soils. Sci Total Environ. 2016;563-564:933–943. doi: 10.1016/j.scitotenv.2015.11.090.
- Lu H, Wang J, Stoller M, et al. An Overview of Nanomaterials for Water and Wastewater Treatment. Adv in Mat Sci and Eng. 2016;2016:1–10. vol. doi: 10.1155/2016/4964828.
- Zahid M, Nadeem N, Mansha A, et al. Hybrid nanomaterials for water purification. In: Multifunctional hybrid nanomaterials for sustainable agri-food and nanomaterials; 2020.155–188. doi: 10.1016/B978-0-12-821354-4.00007-8.
- Mahmoud ME, Saleh MM, Zaki MM, et al. A sustainable nanocomposite for removal of heavy metals from water based on crosslinked sodium alginate with iron oxide waste material from steel industry. J Environ Chem Eng. 2020;8(4):104015. doi: 10.1016/j.jece.2020.104015.
- Markovi D, Tseng H, Nunney T, et al. Applied Surface Science Novel antimicrobial nanocomposite based on polypropylene non-woven fabric, biopolymer alginate and copper oxides nanoparticles. App Surf Sci. 2020;527:146829. doi: 10.1016/j.apsusc.2020.146829.
- Sarkar S, Guibal E, Quignard F, et al. Polymer-supported metals and metal oxide nanoparticles: synthesis, characterization, and applications. J Nanopart Res. 2012;14(2):1–24. doi: 10.1007/s11051-011-0715-2.
- Schnepp Z, Wimbush SC, Mann S, et al. Alginate-mediated routes to the selective synthesis of complex metal oxide nanostructures. CrystEngComm. 2010;12(5):1410–1415. doi: 10.1039/b923543b.
- Annu AA, Ahmed S. Green synthesis of metal, metal oxide nanoparticles, and their various applications. Handbook Ecomater. 2018;2018:1–45.
- Bibi A, Rehman S, Yaseen A. Alginate-nanoparticles composites: kinds, reactions and applications. Mater. Res. Express. 2019;6(9):092001. doi: 10.1088/2053-1591/ab2016.
- Cheung L. Properties of hydrogels incorporating graphene oxide-based nanoparticles for tissue engineering purposes. University of Waterloo; 2016.
- Borkowski D, Krucińska I, Draczyński Z. Preparation of nanocomposite alginate fibers modified with titanium dioxide and zinc oxide. Polymers (Basel). 2020;12(5):1040. doi: 10.3390/polym12051040.
- Zhang K, Luo X, Yang L, et al. Progress toward hydrogels in removing heavy metals from water : problems and solutions: a review. ACS Est Water. 2021;1(5):1098–1116. doi: 10.1021/acsestwater.1c00001.
- Hurtado A, Aljabali AAA, Mishra V, et al. Alginate: enhancement strategies for advanced applications. Int J Mol Sci. 2022;23(9):4486. doi: 10.3390/ijms23094486.
- Priya VN, Rajkumar M, Mobika J, et al. Alginate coated layered double hydroxide/reduced graphene oxide nanocomposites for removal of toxic As (V) from wastewater. Physica E Low Dimens Syst Nanostruct. 2021;127:114527. doi: 10.1016/j.physe.2020.114527.
- Satriaji KP, Garcia CV, Kim GH, et al. Antibacterial bionanocomposite films based on CaSO4-crosslinked alginate and zinc oxide nanoparticles. Food Packag Shelf Life. 2020;24:100510. doi: 10.1016/j.fpsl.2020.100510.
- Thakur S. An overview on alginate based bio-composite materials for wastewater remedial. Mater Today Proc. 2021;37:3305–3309. doi: 10.1016/j.matpr.2020.09.120.
- Mohammadi A, Hossein A, Mahmood K. International Journal of Biological Macromolecules Alginate/calix [4] arenes modi fi ed graphene oxide nanocomposite beads: Preparation, characterization, and dye adsorption studies. Int J Biol Macromol. 2018;120:1353–1361. doi: 10.1016/j.ijbiomac.2018.09.136.
- Priyan I, Fernando S, Lee W, et al. Alginate-based nanomaterials : Fabrication techniques, properties, and applications. Chem. Eng. J. 2020;391:123823. doi: 10.1016/j.cej.2019.123823.
- Polymers I, Review GA, Falah M. Photocatalytic nanocomposite materials based on catalysts. 2020.
- Masula K, Sreedhar P, Vijay Kumar P, et al. Synthesis and characterization of NiO–Bi2O3 nanocomposite material for effective photodegradation of the dyes and agricultural soil pollutants. Mater Sci Semicond Process. 2023;160:107432. doi: 10.1016/j.mssp.2023.107432.
- Nasrollahzadeh M, Sajjadi M, Iravani S, et al. materials for sustainable water treatment : a review. Carbohydr Polym. 2021;251:116986. doi: 10.1016/j.carbpol.2020.116986.
- Baek S, Joo SH, Toborek M. Treatment of antibiotic-resistant bacteria by encapsulation of ZnO nanoparticles in an alginate biopolymer: insights into treatment mechanisms. J Hazard Mater. 2019;373:122–130. doi: 10.1016/j.jhazmat.2019.03.072.
- Masula K, Bhongiri Y, Raghav Rao G, et al. Evolution of photocatalytic activity of CeO2–Bi2O3 composite material for wastewater degradation under visible-light irradiation. Opt Mater (Amst). 2022;126:112201. doi: 10.1016/j.optmat.2022.112201.
- Xiao D, He M, Liu Y, et al. Strong alginate/reduced graphene oxide composite hydrogels with enhanced dye adsorption performance. Polym. Bull. 2020;77(12):6609–6623. doi: 10.1007/s00289-020-03105-7.
- Bakhsh EM, Akhtar K, Fagieh TM, et al. Sodium alginate nanocomposite based efficient system for the removal of organic and inorganic pollutants from wastewater. Int J Biol Macromol. 2021;191:243–254. doi: 10.1016/j.ijbiomac.2021.09.029.
- Radoor S, Karayil J, Parameswaranpillai J, et al. Removal of anionic dye Congo red from aqueous environment using polyvinyl alcohol/sodium alginate/ZSM – 5 zeolite membrane. Sci Rep. 2020;10(1):15452. doi: 10.1038/s41598-020-72398-5.
- Makhado E, Pandey S, Modibane KD, et al. Sequestration of methylene blue dye using sodium alginate poly (acrylic acid)@ ZnO hydrogel nanocomposite: kinetic, isotherm, and thermodynamic investigations. Int J Biol Macromol. 2020;162:60–73. doi: 10.1016/j.ijbiomac.2020.06.143.
- Talbot D, Abramson S, Griffete N, et al. pH-sensitive magnetic alginate/γ-Fe2O3 nanoparticles for adsorption/desorption of a cationic dye from water. J Water Process Eng. 2018;25:301–308. doi: 10.1016/j.jwpe.2018.08.013.
- Zheng Y, Wang A. Ag nanoparticle-entrapped hydrogel as promising material for catalytic reduction of organic dyes. J. Mater. Chem. 2012;22(32):16552–16559. doi: 10.1039/c2jm32774k.
- Nazim M, Aslam A, Khan P, et al. Exploring rapid photocatalytic degradation of organic pollutants with porous CuO nanosheets: synthesis, dye removal, and kinetic studies at room temperature. ACS Omega. 2021;6(4):2601–2612. doi: 10.1021/acsomega.0c04747.
- Chen J, Spear SK, Huddleston JG, et al. Polyethylene glycol and solutions of polyethylene glycol as green reaction media. Green Chem. 2005;7(2):64–82. doi: 10.1039/b413546f.
- Chequer FMD, Oliveira GARD, Ferraz ERA, et al. Textile dyes: dyeing process and environmental impact. Eco-Friendly Textile Dyeing Finish. 2013;6(6):151–176.
- Lu CS, Mai FD, Wu CW, et al. Titanium dioxide-mediated photocatalytic degradation of acridine orange in aqueous suspensions under UV irradiation. Dyes Pigm. 2008;76(3):706–713. doi: 10.1016/j.dyepig.2007.01.009.
- El-Monaem EMA, El-Latif MMA, Eltaweil AS, et al. Cobalt nanoparticles supported on reduced amine-functionalized graphene oxide for catalytic reduction of nitroanilines and organic dyes. Nano. 2021;16(04):2150039. doi: 10.1142/S1793292021500399.
- Sarkar D, Xie X, Kang J, et al. Functionalization of transition metal dichalcogenides with metallic nanoparticles: implications for doping and gas-sensing. Nano Lett. 2015;15(5):2852–2862. doi: 10.1021/nl504454u.
- Ismail M, Khan MI,. Bahadar S, et al. Catalytic reduction of picric acid, nitrophenols and organic azo dyes via green synthesized plant supported Ag nanoparticles. J Mol Liq. 2018;268:87–101. doi: 10.1016/j.molliq.2018.07.030.
- Marimuthu S, Antonisamy AJ, Malayandi S, et al. Silver nanoparticles in dye effluent treatment: a review on synthesis, treatment methods, mechanisms, photocatalytic degradation, toxic effects and mitigation of toxicity. J Photochem Photobiol B. 2020;205:111823. doi: 10.1016/j.jphotobiol.2020.111823.
- Hashmi SS, Shah M, Muhammad W, et al. Potentials of phyto-fabricated nanoparticles as ecofriendly agents for photocatalytic degradation of toxic dyes and waste water treatment, risk assessment and probable mechanism. J Indian Chem Soc. 2021;98(4):100019. doi: 10.1016/j.jics.2021.100019.
- Yang J, Chen C, Ji H, et al. Mechanism of TiO2-assisted photocatalytic degradation of dyes under visible irradiation : photoelectrocatalytic study by TiO2-film electrodes. J Phys Chem B. 2005;109(46):21900–21907. doi: 10.1021/jp0540914.
- Lu CS, Chen CC, Huang LK, et al. Photocatalytic degradation of acridine orange over NaBiO3 driven by visible light irradiation. Catalysts. 2013;3(2):501–516. doi: 10.3390/catal3020501.
- Azad UP, Ganesan V, Pal M. Catalytic reduction of organic dyes at gold nanoparticles impregnated silica materials: influence of functional groups and surfactants. J Nanopart Res. 2011;13(9):3951–3959. doi: 10.1007/s11051-011-0317-z.
- Naseem K, Begum R, Wu W, et al. Catalytic reduction of toxic dyes in the presence of silver nanoparticles impregnated core-shell composite microgels. J Clean Prod. 2019;211:855–864. doi: 10.1016/j.jclepro.2018.11.164.