Abstract
Nanotechnology can be defined as the science and engineering involved in the design, synthesis and application of materials and devices on the nanometer scale. It is not in itself a single emerging discipline, but rather a meeting of several traditional sciences such as chemistry, physics, materials science, biology and engineering to bring together the required expertise needed to develop these new technologies. The unique properties of nanoscale materials provide benefits in remediation, pollution prevention, and efficient use of resources; however, the greatest contribution to green chemistry is likely to be the new manufacturing strategies available through nanoscience. So, the present review mainly focuses on the synthesis of semiconductor CuO nanomaterials from different starting materials by adopting various routes such as vapor-solid, vapor-liquid-solid, solid-liquid, etc. to reveal the morphological features of the precursor.
1. Introduction
Nanomaterials have attracted much attention in recent years because they are both important and interesting: important, as nanomaterials can have useful properties not exhibited by either small molecular systems or larger particulate matter; interesting, as working on supramolecular scales raises exciting synthetic challenges and allows intimate exploration of the relationships among atoms, molecules, and extended systems. Hence in recent years, synthesis and fabrication of one-dimensional (1D) nanostructured materials have received much research attention, due to their outstanding properties and potential applications of this class of materials Citation1. For example, 1D nanobuilding blocks of transition metal oxides, hydroxides, sulfides, semiconductors, metals, and metal salts have now been prepared by various process techniques giving rise to the possibility of constituting a nanotoolbox for a “bottom-up” approach in nanoscience and nanotechnology Citation1–4. Among the various materials mentioned above, semiconductor nanoscale materials, such as nanowires, nanorods, and nanodiscs have stimulated great interest due to their importance in basic research and potential applications Citation5. They are quite attractive as components of electronic devices because many of their physical properties can be controlled through modification of the sizes and shapes of the building blocks Citation6, Citation7. To this end, various techniques including the vapor-phase transport, laser ablation, chemical vapor deposition, arc-discharge, template-based, solution-chemistry methods, etc., have been developed to prepare well-aligned 1D nanomaterials Citation8–13. However, it is still a challenge to develop simple methods for the fabrication of uniform nanomaterials that are cost-effective and allow large-scale production. Our main focus here is to review recent progress to establish convenient route (vapor–solid, vapor–liquid–solid, solid–liquid, etc.) to prepare robust semiconductor CuO nanostructured materials because this material has an advantage for application towards electronic and optoelectronic industry.
2. Preparation and characterization of CuO nanoparticles starting from the corresponding salts
In past decades, most of the research on synthesis of anisotropic nanocrystals has largely focused on II–IV semiconductor systems Citation14–20 other systems reported in the literature are very limited. Recently, copper-based nanomaterials have received attention because of their potential application in optoelectronic devices, catalysis and superconductors. CuO is a p-type semiconductor (Eg = 1.2 eV) with excellent photovoltaic, electrochemical, and catalytic properties Citation21-24. Also, it is inexpensive, non-toxic, and readily available.
Xu et al . Citation25, Citation26 reported the synthesis of CuO nanocrystals by one step solid state reaction under ambient conditions by grinding 2:5 molar ratios of CuCl2 · 2H2O and NaOH in an agate mortar followed by a short induction period of 30 min. The obtained black product was washed with an ultrasonic wave, distilled water (3 times), alcohol (2 times), and then dried in air. This method yielding polyhedral CuO nanocrystals with an average size of 12 nm was confirmed by TEM image (). XRD diffraction peaks () also confirmed that the size of this CuO nanocrystal was found to be same by immediately pressing the powder as a pellet. Whereas an increase in grain size from 12.1 nm to 16.4 nm was observed on aging (15 days) of the pellet may be due to the more closely packed nanocrystals.
Figure 1. TEM micrograph of the CuO particles (×150,000) Citation25.
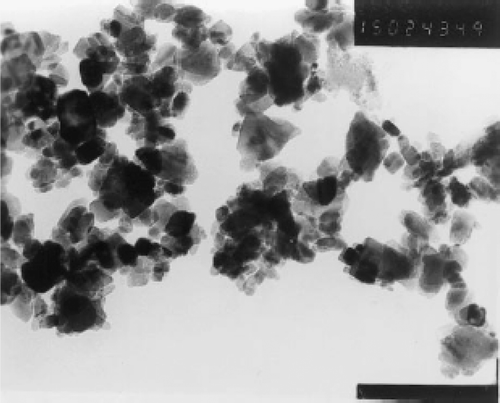
Figure 2. XRD curves of CuO pellet samples (a) just after pressed, (b) 4 days later, and (c) 15 days later at RT Citation25.
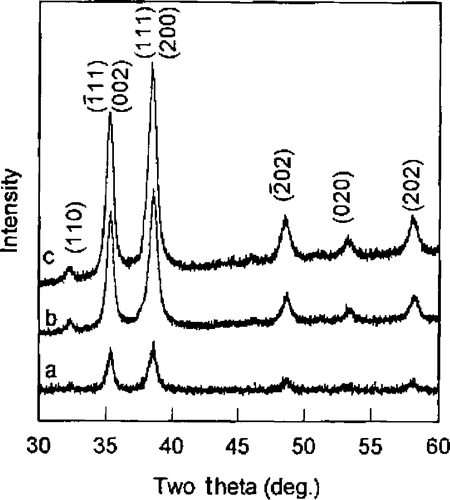
CuO belongs to the space group shows three acoustic modes (Au + 2Bu), six infrared active modes (3Au + 3Bu), and three Raman active modes (Ag + 2Bg). Thus, the Raman spectra of CuO nanocrystal show three peaks at 282 cm−1, 332 cm−1 and 618 cm−1 (). With aging, Raman peaks become sharper and show a slight blue shift because the grain size increases from 12.1 nm to 16.4 nm. From this study, it is concluded that there was change in the grain size only in the pressed pellets (due to their being more closely packed), not in CuO powder from XRD and Raman measurements.
Figure 3. Raman spectra of CuO pellet samples (a) just after pressed, (b) 4 days later at RT, (c) 15 days later at RT, (d) annealed at 500°C for 2 h, and (e) annealed at 800°C for 2 h Citation25.
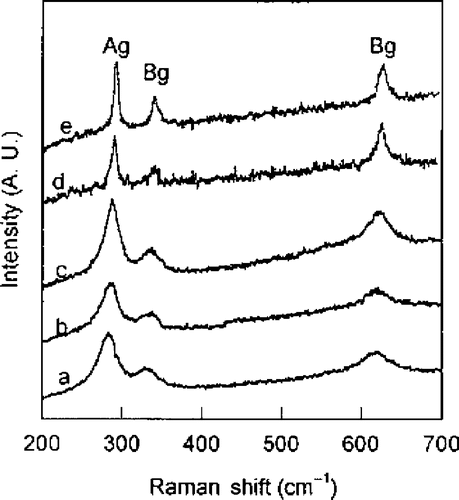
Wang et al . Citation27 prepared CuO nanowhiskers similar to Xu et al . Citation25 but in the presence of a nonionic surfactant. About 5.045 g of CuCl2 · 2H2O and 3 g of solid NaOH were ground for 5 min. To this mixture, 6 ml (6.78 g) of PEG 400 was added and the grinding continued for 30 min before washing the mixture several times with distilled water in an ultrasonic bath and then with ethanol to remove PEG 400, NaCl and unreacted precursors. Finally, the products were dried for 3 h in an oven at 60°C in air. The CuO nanowhiskers most likely form via the following reaction:
Figure 4. TEM image of the CuO nanowhiskers sample Citation27.
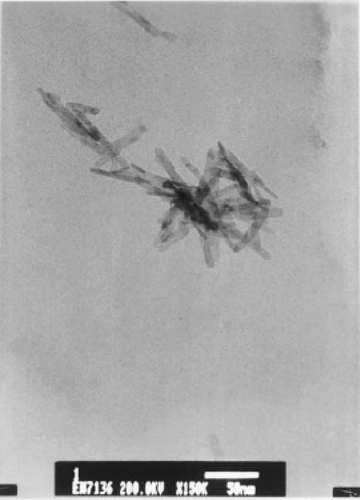
In addition, they also carried out preparation of CuO nanorods in the presence of nonionic surfactant Citation29 as follows. About 200 mg of PEG (Mw 20,000) and 178.48 mg of CuCl2 · 2H2O were dissolved in 200 ml of H2O, which was stirred with a magnetic stirrer continuously until both the PEG and the CuCl2 dissolved completely. Then 2 ml of NaOH solution (6 M) was added to the above mixture drop wise under constant stirring. While stirring Cu(OH)2 blue precipitate formed which upon heating by steam for 30 min, generates CuO. The black CuO precipitate was washed several times with distilled water and then with ethanol to remove the PEG completely, filtered and dried in an oven at 80°C for 5 h. TEM image () revealed that these materials have a relatively straight rod-like shape (diameters of ∼10 nm and of length up to 400 nm) and smooth surfaces. The CuO lattice constants calculated for these nanorods by XRD data is consistent with the standard values of bulk CuO Citation30 and also results clearly indicate that the obtained nanorods were in fact crystalline CuO belonging to the monoclinic space group .
The CuO nanorods form here via two steps as shown below:
Figure 5. TEM image of the CuO nanorods Citation29.
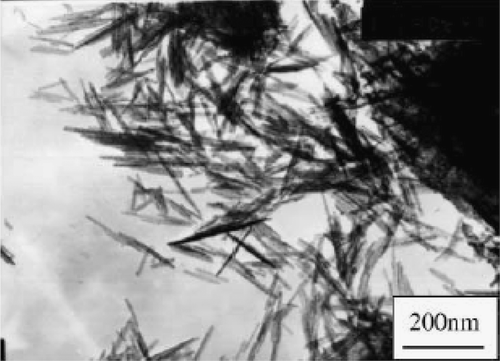
Similarly, Gao et al . Citation31 synthesized CuO nanorods starting from CuCl2 as follows. First, Cu(OH)2 precipitates were prepared by mixing 0.5 M solution of both CuCl2 and NaOH. The obtained precipitates were dispersed in a 15 M NaOH solution of which one part of the dispersion was transferred into an autoclave with a PTFE container and kept at room temperature, while the second part was maintained at 100°C for 48 h. The obtained precipitates were washed in distilled water (until the solution pH ∼ 7) and then dried at 100°C in air for 1 day. The average crystalline sizes of obtained CuO samples at room temperature and 100°C were estimated to be 17 nm and 73 nm from the XRD patterns (using Sherrer equation). The room temperature product was uniform fine nanorods of about 10–20 nm thick and several hundred nanometers long which are even thinner than the precursor Cu(OH)2 precipitate (40–60 nm). Whereas at a temperature of 100°C, bulk CuO nanorods were obtained with a diameter 60–100 nm in size and length of up to 1 µm (). Thus, the morphological features of the synthesized nanorods were highly dependent on temperature and caustic soda present in autoclaves. Also from the selected area electron diffraction (SAED) patterns it is concluded that bulk nanorods obtained at 100°C consist of single crystals while the fine nanorods obtained at room temperature have a polycrystalline structure (see inset of ).
Figure 6. TEM images of Cu(OH)2 precipitates dried at 100°C (a) and as-prepared nanorods at room temperature (b) and 100°C (c). The selected area electron diffraction of the as-prepared nanorods is inserted Citation31.
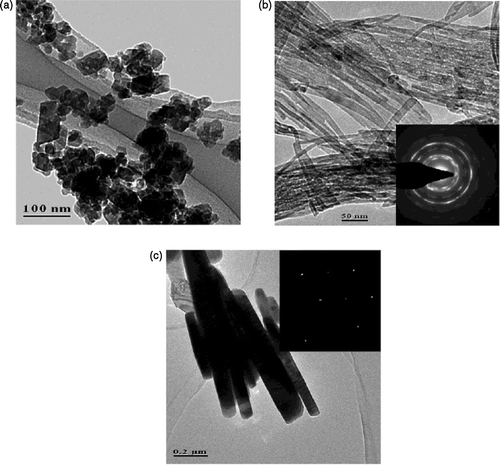
Secondly, Punnoose et al . Citation32 reported preparation of CuO nanoparticle by the sol-gel method. That is, mixing aqueous solutions of copper nitrate and sodium hydroxide at room temperature and by maintaining the pH ∼ 10. The resulting Cu(OH)2 gel was washed several times with distilled water until free of nitrate ions, then centrifuged and finally dried in air. In order to get CuO nanoparticles, the Cu(OH)2 gel obtained above was calcined in air for 3 h at the selected temperatures of 160, 200, 250, 300, 400, 600, 800 and 1000°C. The XRD patterns of the calcined samples () show only lines due to CuO nanoparticles and also the particle size increases from 6.6 nm to 37 nm as the calcined temperature increases is determined by employing the Scherrer relation to the (202), (202) and (113) reflections Citation33. The increase in grain size that may be due to the fusion of the particles on sintering is confirmed further by TEM micrographs (). That is, upon sintering small particles get aggregated and, in addition, the occurrence of surface faceting is evident from TEM micrographs.
Figure 7. Room-temperature XRD patterns of CuO particles annealed at different temperatures shown. The particle size shown is the average value determined from the (20), (202), and (11
) lines using the Scherrer relation, after correcting for the instrumental width. Temperatures are in °C Citation32.
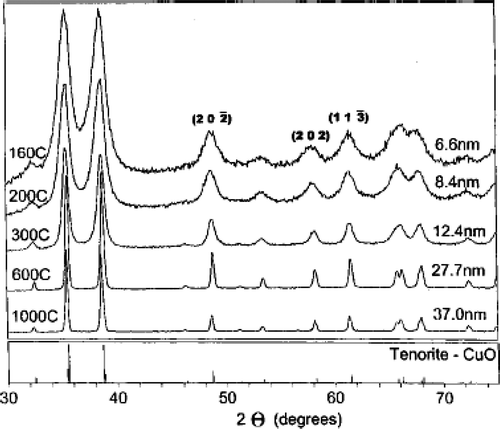
Figure 8. TEM characterization reveals agglomeration of the nanoscale particles. A cluster of 6.6 nm particles, shown in (a), consists of highly faceted single particles (b). A cluster of the nominal 33 nm particles is shown in (c). These large particles have surfaces decorated with smaller particles (d), which exhibit defects such as multiple twinning (arrow) Citation32.
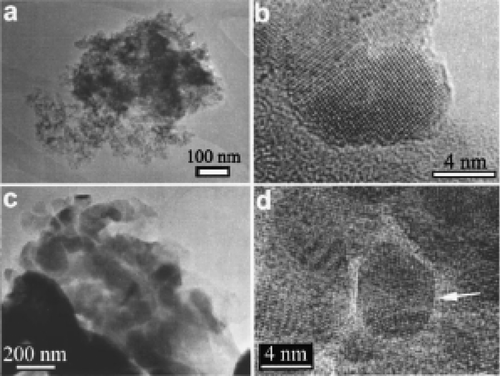
Viano et al . Citation34 confirmed the agglomeration of nanoscale particles by TEM analysis () while following the same synthesis procedure of Punnoose et al . Citation32. They used electron spin resonance (ESR) technique to probe the calcination temperature effect on magnetic ordering in nano and bulk CuO powder. From the ESR results (), they concluded that (1) line shapes belong to typical polycrystalline material, (2) the appearance of broad signals are ascribed to weak antiferromagnetic ordering in CuO, and (3) the difference in line widths of the ESR signals between bulk and nanopowder indicates a high defect concentration in nanopowder, which must arise from the comparatively high surface area of nanoparticles. Thus the comparative study of bulk and nano CuO powder highlights the importance of particle size on the magnetic properties of nanoparticles.
Figure 9. TEM image of CuO nanopowder calcined at 200°C Citation34.
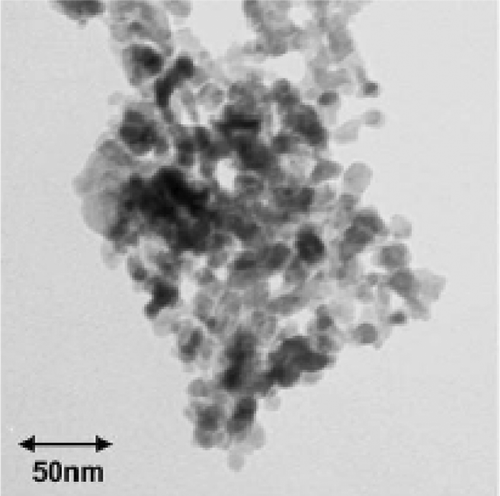
Figure 10. Overlay of ESR spectra of (a) CuO nanopowder and (b) CuO bulk calcined at different temperatures. The calcination temperature is written just above each curve Citation34.
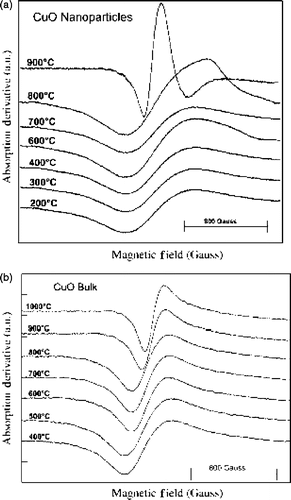
Zhu et al . Citation35 reported preparation of CuO nanoribbons starting from Cu2Cl(OH)3 nanoribbons by template free method. First, Cu2Cl(OH)3 nanoribbons were prepared by mixing 1:2 molar ratio of CuCl2 · 2H2O and (NH4)2CO3 solution and keeping the solution aside for 2–7 days. The jade-green precipitate formed was washed with distilled water and ethanol, and then dried at 60°C for 12 h yields Cu2Cl(OH)3 nanoribbons (see for TEM image). The reaction that accounts for the formation of Cu2Cl(OH)3 nanoribbons probably proceed as follows:
Figure 11. The TEM images of the Cu2Cl(OH)3 nanoribbon Citation35.
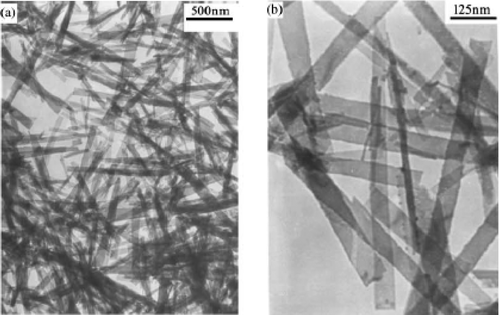
Figure 12. TEM image of CuO nanoribbon obtained by heat treatment of the Cu2Cl(OH)3 nanoribbon Citation35.
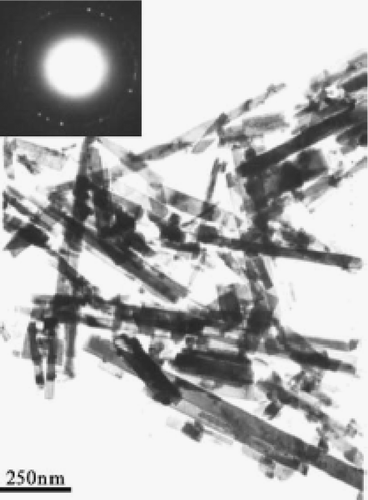
Song et al . Citation37 reported a different approach to the preparation for CuO nanowires starting from Cu(DEHP)2 complex is as follows. First, Cu(DEHP)2 complex were generated at pH 6 by mixing 2:1 molar ratio of bis(ethylhexyl) hydrogen phosphate (HDEHP) and CuCl2 in heptane and water. Secondly, Cu(OH)2 nanowire arrays were prepared at room temperature by injecting 20 ml of Cu(DEHP)2 complex (0.05 M) in n-heptane into 20 ml of aqueous NaOH solution (0.05 M) taken in a 50 ml test tube. After several minutes, a blue colour gradually grew at the solution interface showing that the reaction occurs at the static organic/aqueous biphasic boundary. The product formed was taken out of the test tube after a given reaction time, washed with absolute alcohol, deionozied water, and dried in air. XRD and TEM analysis () confirmed the formation of Cu(OH)2 nanowires at the interface. Finally, black CuO nanowire arrays () were obtained by allowing the above reactions to take place at (i) higher concentration of NaOH and (ii) more reaction time.
Figure 13. XRD and TEM image of Cu(OH)2 prepared at the static interface between Cu(DEHP)2 = 0.05 M in n-heptane and NaOH = 0.05 M Citation37.
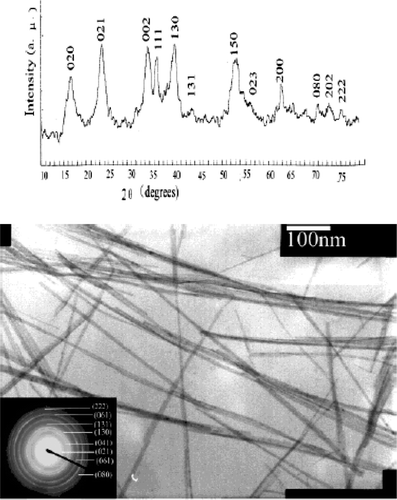
Figure 14. TEM images of CuO obtained from the dehydration of Cu(OH)2 at the different concentrations of NaOH (a) and (b) Cu(DEHP)2 = 0.05 M and NaOH = 0.1 M at different magnifications. (c) Cu(DEHP)2 = 0.05 M and NaOH = 0.2 M Citation37.
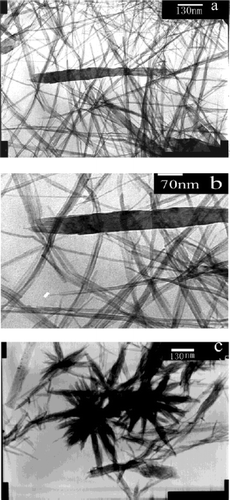
Lu et al . Citation38 reported a simple template-free solution route for the controlled synthesis of Cu(OH)2 and CuO nanostructures starting from CuSO4 solution. They followed two different methods for the synthesis of Cu(OH)2 nanostructures, i.e., the KOH/NH3 route and the NH3/KOH route. In the first route (KOH/NH3), about 0.8 ml of KOH solution (1M) was added into 2 ml of CuSO4 solution (0.1 M) under rigorous stirring at ambient temperature followed by addition of 0.3 ml of ammonia solution (12.5 wt%, ∼7.0 M). Further stirring the sample for a while and then kept mixed solution static in a sealed vessel for 12 h followed by filtering, washing thoroughly with deionized water and dried in a vacuum at 25°C for 12 h generates Cu(OH)2 nanowires (). For the second route (NH3/KOH), the synthesis procedures are same but the feeding order of NH3 and KOH are vice-versa compared to the first method. For this method Cu(OH)2 nanoribbons were obtained which was confirmed by SEM and TEM images (). The exact reactions for the selective formation of Cu(OH)2 nanowires and nanoribbons are as follows:
(a) KOH/NH3 route
(b) NH3/KOH route
Figure 15. SEM (a, b), TEM (c, d), and HRTEM (e) images of Cu(OH)2 nanowires prepared via the KOH/NH3 route aged for 12 h. Inset shows ED pattern of the bundled nanowires. Scale bars: (a) 5 µm; (b) 500 nm; (c) 100 nm; (d) 50 nm; (e) 5 nm Citation38.
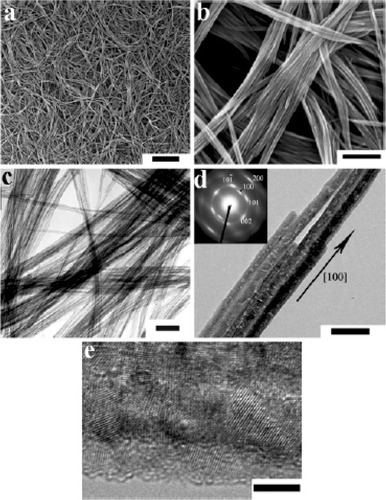
Figure 16. SEM (a, b) and TEM (c, d) images and ED (e) patterns of Cu(OH)2 nanoribbons prepared via the NH3/KOH route. The ED pattern shown in plate e corresponds to the single nanoribbon shown in plate d. Scale bars: (a) 5 µm; (b) 1 µm; (c) 200 nm; (d) 100 nm Citation38.
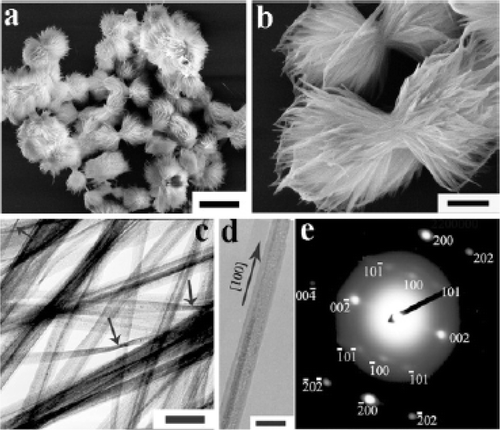
Figure 17. SEM images (a, b) of CuO nanoplatelets obtained by thermal dehydration of the Cu(OH)2 nanowires prepared via the KOH/NH3 route immediately after the addition of the KOH and ammonia solutions: (a) 30°C; (b) 50°C. Scale bars: 500 nm Citation38.
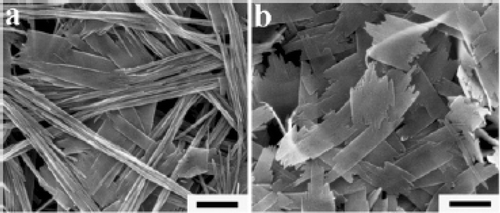
Figure 18. SEM (a) and TEM (b) images of CuO nanowires obtained by thermal dehydration of the as prepared Cu(OH)2 nanowires in the solid state at 120°C for 2 h. Scale bars: (a) 500 nm; (b) 200 nm Citation38.
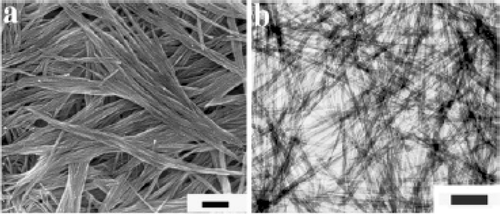
Figure 19. SEM images of CuO nanostructures obtained by thermal dehydration of the as prepared Cu(OH)2 nanoribbons in water at 70°C for 12 h. Scale bars: (a) 2 µm; (b) 500 nm Citation38.
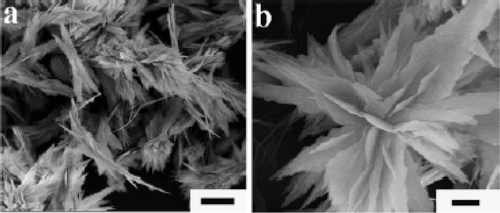
Zeng et al . Citation39 reported synthesis of CuO nanorods and nanoribbons by wet chemical method in which water--ethanol mixture is used as a solvent. 40 ml of Cu(NO3)2 · 3H2O (0.01 M in pure ethanol) was slowly added at a rate of 0.1 ml/min using a mechanically pumped syringe to 40 ml of NaOH (0.5 M in ethanol) solution under stirring in a Teflon flask at 65°C and the resultant product was then aged together with mother liquor at the same temperature for 12–36 h respectively. Separation of CuO nanocrystals were done by centrifugation at a speed of 6000 rpm for 10 min followed by washing with de-ionized water repeatedly and then dried under vacuum overnight at room temperature. In addition, they found that the dimensions of the nanostructured CuO can be controlled across a wide spatial span from nanometer to micrometer regime via manipulating synthetic parameters (summarized in ) and most importantly desired morphology of CuO crystallites (rod, wire, ribbon, net, platelet or sheet) can be obtained by selecting simple changes of reaction conditions. To achieve this, NaOH is playing a number of roles in the synthesis. First it combines with Cu(NO3)2 to form Cu(OH)2, which is then decomposed to CuO as follows:
Table 1. Experimental procedures and product results Citation39.
In addition, they reported how CuO crystallites self-organized into spherical assemblies or “dandelions” with a puffy appearance () Citation40. The synthesis procedure for such samples is as follows: About 15–25 ml of Cu(NO3)2 · 3H2O (1 M in pure ethanol solvent) was added to 15–25 ml of ammonia solution (32%), followed by an addition of 4–5 ml of aqueous NaOH (1 M) and 0–5 g of solid NaNO3. The solution mixture was then transferred to a Teflon-lined stainless steel autoclave and heated at 100–180°C for 2–24 h in an electric oven. After reaction, the autoclave was cooled in tap water, and CuO products were washed via centrifugation-redispersion cycles, with each successive supernatant being decanted and replaced with deionized water and ethanol. Under the reported conditions, the prepared CuO products are all in the “dandelion”-like morphology (100%), with diameters ranging from 4–8 µm.
Figure 20. SEM images of CuO microspheres (prepared at 100°C, 24 h): (a) overall product morphology; (b and c) detailed views on average sized spheres; and (d) a detailed view on an individual sphere Citation40.
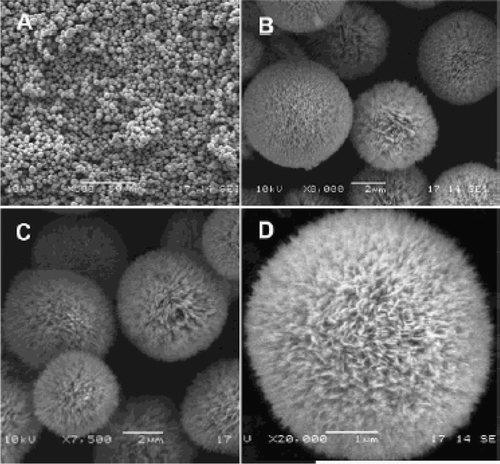
3. Preparation and characterization of CuO nanoparticles starting from the corresponding acids
In past decades, much effort has been made towards the synthesis and characterization of nanosized transition metal oxide particles. Among all transition metal oxides that have been investigated, much less time has been devoted to the preparation and characterization of CuO nanoparticles. For example, Yang and Gao Citation41 reported preparation of CuO nanocrystals with various morphologies via a simple and convenient hydrothermal decomposition route. That is, by mixing about 0.5 g of Cu(COOH)2 in a series of mixture of anhydrous dimethyl sulphoxide (DMSO) and distilled water (total volume 70 ml and volume ratios were 50:3, 10:1, 5:1, 5:2 and 1:1) in a Teflon-lined autoclave. The whole mixture was then maintained at 110°C for 3 h and cooled to room temperature naturally yields CuO nanocrystals. The obtained CuO black precipitates were collected and washed with distilled water followed by absolute ethanol several times. The final products were dried in vacuum at 60°C for 6 h.
The following reactions supported such preparation methods.
Figure 21. Representative TEM images of CuO nanocrystals synthesized at various volume ratios of DMSO and water: (a) 50:3; (b) 10:1; (c) 5:1; (d) 5:2; (e) 1:1 Citation41.
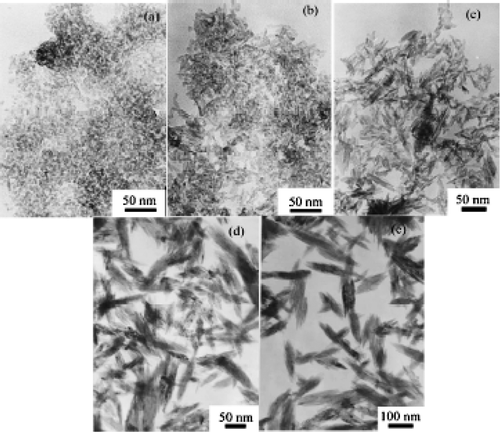
Xu et al . Citation44 reported preparation of CuO nanorods by thermal decomposition of CuC2O4 similar to Yang and Gao Citation41 but they use copper acetate, oxalic acid and surfactant (nonyl phenyl ether) as starting compounds. That is, about 3.99 g of Cu(CH3COO)2 · H2O and 2.53 g of H2C2O4 · 2H2O were mixed with 5 ml of nonyl phenyl ether 9/5 (NP-9/5) in a mortar, grind for several minutes and kept in a thermostat oven at 50–60°C for 6 h. Then the product was washed several times with distilled water and acetone to remove unreacted reactants and then dried in an oven at 70–80°C for 12 h to yield pure CuC2O4. To yield CuO nanorods, grind about 2 g of CuC2O4 as synthesized above with 8 g of NaCl powder, and then annealed at 950°C for 2 h in a porcelain crucible. Finally, the annealed sample was washed with distilled water one time, ethanol three times, ethyl ether one time using an ultrasonic bath and then centrifuge to yield CuO nanorods. TEM morphologies () confirmed the as-synthesized CuO nanorods were around 30 nm in diameter and length of several micrometers. The existence of conical tip at the end of nanorod conforms that the growth mechanism of the CuO nanorods is most likely controlled by the vapour-solid (VS) growth mechanism.
Figure 22. TEM images of the as-prepared CuO nanorods. (a) Morphologies of the as prepared CuO nanorods. (b) Morphologies of the as prepared single CuO nanorod Citation44.
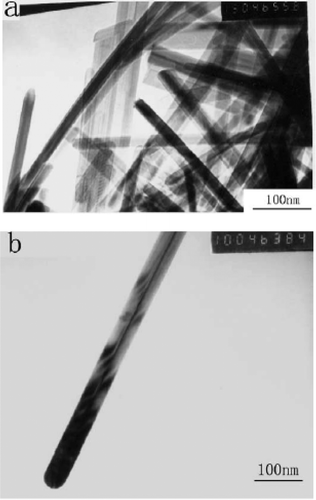
The following reactions supported such preparation methods.
Hong et al . Citation45 also followed alcohothermal route to synthesize CuO nanoparticles starting from copper acetate. That is 50 ml of copper acetate alcoholic solution (0.05 M) was taken in a Teflon lined stainless steel autoclave and kept constant in an oven at various temperatures (90°C, 110°C, 130°C, 150°C and 180°C) for 20 h. After cooling the autoclave to room temperature, the products were recovered by centrifugation, washed with distilled water (twice), ethanol (three times) and then vacuum dried at room temperature. Through this approach, stable CuO nanoparticles as small as 3–9 nm have been successfully synthesized below 150°C. However, the rate of formation of CuO nanoparticle was slow when the synthesis temperature was below 90°C and on the other hand, if the temperature was raised above 180°C, reducing alcohol would readily reduce the Cu(II) to metallic copper nanoparticles with a relatively large size of 54.1 nm. This may be confirmed from the XRD patterns ().
Figure 23. X-ray diffraction patterns of the samples prepared using the alcohothermal route at different temperatures: (a) 90°C; (b) 110°C; (c) 150°C; and (d) 180°C Citation45.
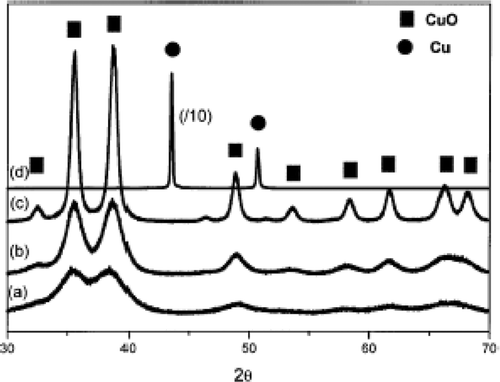
Similarly, Wang et al . Citation46 reported synthesis of CuO nanoparticles by microwave irradiation of copper acetate. About 25 ml ethanolic solutions of both copper acetate (0.2 M) and NaOH (0.01 M) were taken in a round-bottomed flask with 0.5 g of polyethylene glycol −19,000 (PEG). The reaction was carried out under ambient air by keeping the total mixture under microwave irradiation. The microwave oven followed a working cycle of 6 s on and 24 s off and altogether the on/off heating procedure was repeated 20 times. After cooling the irradiated mixture to room temperature, the products were recovered by centrifugation, washed with distilled water, absolute ethanol and acetone, and then dried in air at room temperature.
By this microwave irradiation approach, the size of the CuO particles obtained can be in the range of 3–5 nm () with the presence of dispersant (PEG). While in the absence of dispersant (PEG), CuO nanoparticles get agglomerated to form some quadrate aggregates of over 200 nm (). These can be explained by the stabilization effect of the surface caused by PEG. That is, the existence of dispersant PEG may reduce the Gibbs's free energy at the surface of the CuO nanoparticles and thus prevent the grains from merging into larger ones. The mechanism for this approach is proposed similar to Iwasaki et al . Citation47 as follows.
Figure 24. (a) TEM and (b) electron diffraction pattern of the as-prepared CuO nanoparticles Citation46.
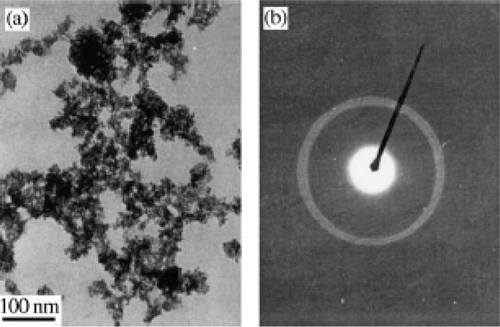
Figure 25. TEM image of the CuO nanoparticles prepared in the absence of PEG Citation46.
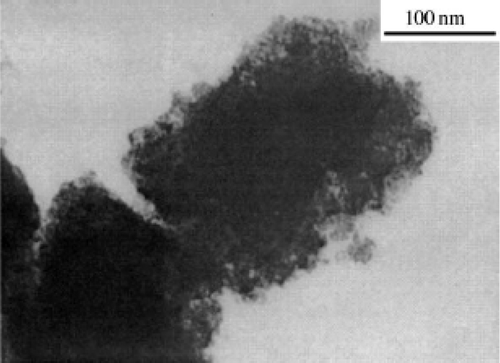
4. Preparation and characterization of CuO nanoparticles starting from the corresponding metal
Apart from the routine method of synthesis of CuO nanoparticles starting from their corresponding salts, there are also reports Citation1 available on syntheses of CuO nanoparticles from the corresponding metal. For example, Borgohain et al . Citation48 prepared stable CuO nanoparticles having size as small as 4 nm by novel electrochemical route using a copper metal electrode as sacrificial anode and platinum as cathode in an electrochemical bath comprised of tetraoctylammonium bromide (TOAB) in acetonitrile and tetrahydrofuran, in the ratio 4:1 as electrolyte. This method gives a high yield of sample, without any undesired side products compared to the synthetic routes involving corresponding salts as their starting material.
Secondly, Lo et al . Citation49 reported preparation of CuO nanofluid by submerged arc nanoparticle synthesis system (SANSS) using a copper electrode. A pure copper rod is deployed to prepare nanoparticles, submerged in dielectric liquid (deionized water) in the vacuum chamber. By generating an electric arc at high temperature (between 6000–12,000°C), the rod is melted and vaporized, undergoing nucleation, growth and condensation in the submerged deionized water to disperse nanoparticles. The morphology of the CuO nanoparticle prepared by this method revealed a needle-like structure () with an average width of 20 nm and length of 80 nm.
Figure 26. SEM image of the CuO nanoparticle Citation49.
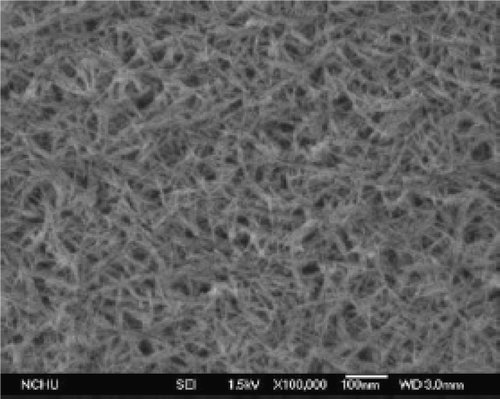
In the late 1950s, Nabarro and Jackson Citation5 reported preparation of CuO and Cu2O nanowhiskers of short length (<5 µm) and large diameter (>100 nm) by oxidizing copper substrate. But recently, Jiang et al . Citation50 reported preparation of CuO nanowires of controllable diameter in the range of 30–100 nm with lengths of up to 15 µm by simply heating copper foils at different temperature and growth time. They followed the procedure as follows: First, the copper substrate was cleaned in an aqueous 1 M HCl solution, followed by repeated rinsing with distilled water. After it had been dried under a N2 gas flow; it was placed in an alumina boat and immediately heated to the set-point temperature (at ambient pressure) in a furnace. The surfaces of the copper substrates were tarnished (when viewed by the naked eye) after they had been treated in air at elevated temperatures. Further examination of the sample under optical or electron microscope indicated the formation of wire-like nanostructures over the entire surfaces of these substrates. For example, TEM images () conclude that the diameter of the CuO nanowires could be varied in the range of 30–100 nm by controlling the reaction temperature. The following reactions can be summarized for this entire synthesis, with the second one being as the rate-determining step for the formation of CuO vapour Citation51.
Figure 27. TEM images of CuO nanowires prepared by heating copper grids in air for 4 h at various temperatures: (a) 400°C, (b) 500°C, and (c) 600°C. The corresponding size distributions of these nanowires are shown in graphs D to F. These results suggest that the diameter of the CuO nanowires could be varied in the range of 30–100 nm by controlling the reaction temperature Citation50.
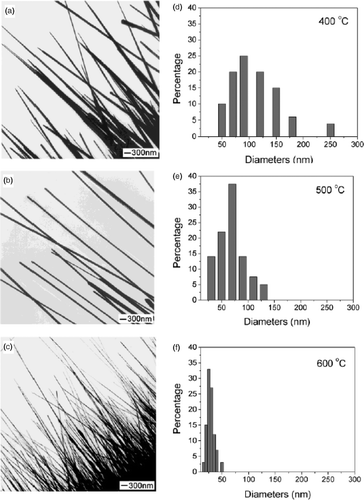
Similarly, Yu et al . Citation52 reported preparation of CuO nanorods of diameter 20–150 nm and length of several micrometers by annealing the copper plate at 400°C for 24 h in air. However, Huang et al . Citation53 prepared large scale of CuO nanowires by thermal evaporation of copper foil at various temperatures (300–800°C) under an oxygen flow rate of about 50 ml/min. Also, they determined that the diameter and length of nanowires vary with the evaporation temperature and time. For example, SEM images () show the morphologies of the samples prepared at various temperatures and they also proposed growth mechanism similar to Xia et al . Citation50.
Figure 28. SEM images of CuO nanowires prepared at various temperatures: (a) 300°C, (b) 400°C, (c) 500°C, (d) 600°C, (e) 750°C and (f) 800°C Citation53.
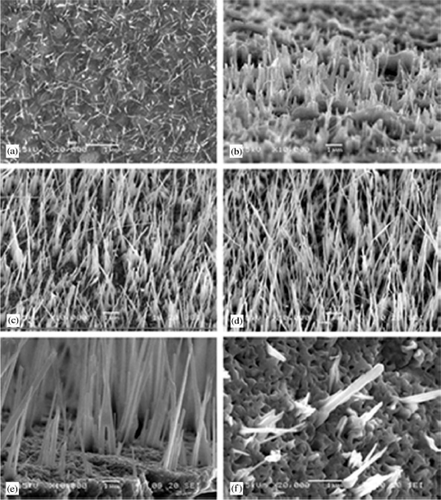
Though preparation of CuO nanoparticles by different approaches are mentioned above, Yang et al . Citation54 obtained CuO nanoribbon arrays on copper converted from Cu(OH)2 nanoribbon arrays prepared by solution-solid reactions. That is, copper foil was washed with a 4 M HCl aqueous solution and subsequently with deionized water repeatedly to remove surface impurities. The washed copper foil was then immersed into a dilute solution of ammonia (3.3 × 10−2 M, pH ≈ 10) for certain periods of time (12–96 h) and then taken out of the solution, washed with deionized water. The blue film covered uniformly on the Cu substrate was found to be Cu(OH)2 nanoribbon arrays, which was confirmed by SEM analysis (). To get CuO nanoribbons, the above prepared Cu(OH)2 nanoribbon sample was loaded into a quartz boat and heated in a furnace under constant flow of N2 (∼25 sccm) at 120°C for 3 h to get dehydration and then heated at 180°C for 2 h to promote crystallization. Adopting this procedure, CuO nanoribbons () were obtained with an average width of ∼50–60 nm and several nanometers long by varying the reaction time and temperature interval. The reactions that account for the formation of Cu(OH)2 and CuO nanoribbons probably proceed as follows:
Figure 29. SEM images of a Cu(OH)2 nanoribbon array. (a) Top view. (b) Side view Citation54.
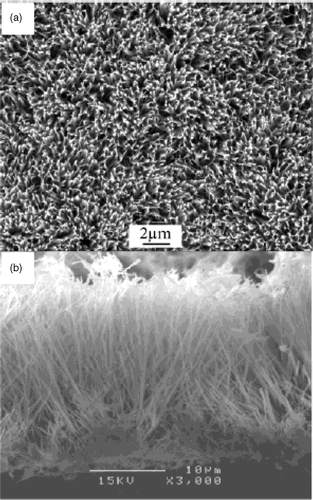
Figure 30. TEM image (a), XRD pattern (b), SEM image (c), and HRTEM image (d) of CuO nanoribbons converted from the Cu(OH)2 nanoribbons by heat treatment. Inset of part A: SAED pattern of an ensemble of CuO nanoribbons. Inset of part D: SAED pattern of a single CuO nanoribbon Citation54.
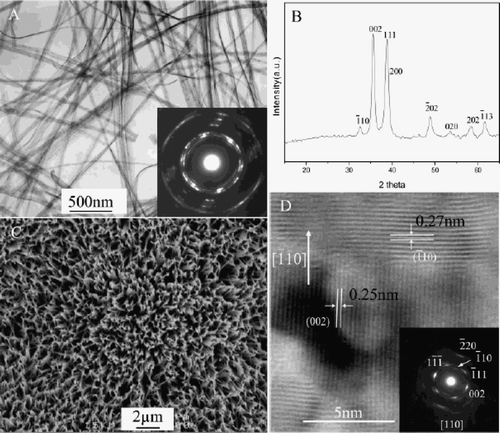
Figure 31. SEM images of p-CuO nanorods prepared at various pH and reaction time intervals: (a) pH 11 (96 h); (b) pH 11 (130 h); (c) pH 11.5 (96 h); (d) pH 11.5 (120 h); (e) pH 12 (96 h); (f) pH 12.3 (14 days) Citation55.
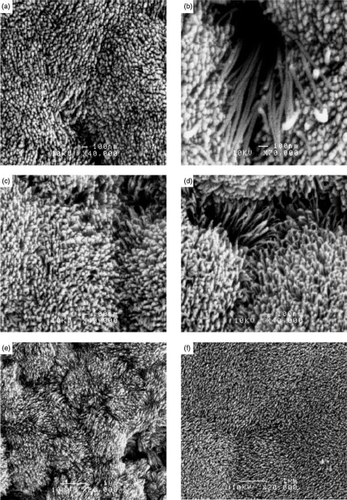
5. Conclusion
In summary, copper oxide nanoparticles of different crystalline structures and morphologies were synthesized successfully starting from the corresponding salts, acids and metal. Each method available here has its own significant advantages in preparing CuO nanoparticles of particular morphology and structure. But in order to identify which is the best method for large-scale production many factors (cost effective, preparation conditions, required facilities, etc) are to be taken into account. A great variety of procedures are mentioned in this paper, giving rise to the possibility of constituting a nanotoolbox for a “bottom-up” approach in nanoscience and nanotechnology. From the results, it is hoped that preparation of CuO nanoparticles will provide the impetus for solution-phase chemical synthesis to be considered as the most promising route in terms of cost, throughput, and the potential for large-scale production.
Overall, we are happy if the present survey gives researchers an opportunity to explore available methods to synthesize semiconductor CuO nanomaterials from different source materials. Thus, the given examples here illustrate the most impressive methods, which are available in the preparation of CuO nanomaterials in view of the nano-science. The investigation of nano-science will remain a challenging task in the near future until it is regarded as a genuine field of science.
Acknowledgements
Author (SA) wishes to thank DST, New Delhi for the Fast Track Young Scientist award and The Director of the National Institute of Technology, Trichy, for providing funding from the TEQIP programme to create a new lab (Nanomaterials and Solar Energy Conversion).
References
- Wang , ZL . 2003 . Nanowires and Nanobelts: Materials, Properties and Devices , Vol. 1-2 , Boston : Kluwer Academic Publisher .
- Iijima , S and Ichihashi , T . 1993 . Single-shell carbon nanotubes of 1 nm diameter . Nature , 363 : 603
- Wen , JG , Lao , JY , Wang , DZ , Kyaw , TM , Foo , YL and Ren , ZF . 2003 . Self-assembly of semiconducting oxide nanowires, nanorods, and nanoribbons . Chem. Phys. Lett. , 372 : 717
- Patzke , GR , Krumeich , F and Nesper , R . 2002 . Oxidic nanotubes and nanorods -- anisotropic modules for a future nanotechnology . Angew. Chem. Int. Ed. , 41 : 2446
- Nabarro , FRN and Jackson , PJ . 1958 . “ Growth of crystal whiskers ” . In Growth and Perfection of Crystal Growth , Edited by: Doremus , R.H. , Roberts , B.W. and Turnbull , D. 13 – 120 . New York : Wiley .
- Ahmadi , TS , Wang , ZL , Green , TC , Henglein , A and El-Sayed , MA . 1996 . Shape-controlled synthesis of colloidal platinum nanoparticles . Science , 272 : 1924
- Albe , V , Jouanin , C and Bertho , D . 1998 . Influence of II–VI nanocrystal shapes on optical properties . J. Cryst. Growth , 185 : 388
- Morales , AM and Lieber , CM . 1998 . A laser ablation method for the synthesis of crystalline semiconductor nanowires . Science , 279 : 208
- Martin , CR . 1994 . Nanomaterials -- a membrane-based synthetic approach . Science , 266 : 1961
- Wang , YD , Ma , CL , Sun , XD and Li , HD . 2002 . Preparation of nanocrystalline metal oxide powders with the surfactant-mediated method . Inorg. Chem. Commun. , 5 : 751
- Shi , WS , Zheng , YF , Wang , N , Lee , CS and Lee , ST . 2001 . A general synthetic route to III--V compound semiconductor nanowires . Adv. Mater. , 13 : 591
- Pan , ZW , Dai , ZR and Wang , ZL . 2001 . Nanobelts of semiconducting oxides . Science , 291 : 1947
- Liu , SW , Yue , J and Gedanken , A . 2001 . Synthesis of long silver nanowires from AgBr nanocrystals . Adv. Mater. , 13 : 656
- Li , YD , Liao , HW , Ding , Y , Qian , YT , Li , Y and Zhou , GE . 1998 . Nonaqueous synthesis of CdS nanorod semiconductor . Chem. Mater. , 10 : 2301
- Wang , WZ , Geng , Y , Yan , P , Liu , FY , Xie , Y and Qian , YT . 1999 . Synthesis and characterization of MSe (M = Zn, Cd) nanorods by a new solvothermal method . Inorg. Chem. Commun. , 2 : 83
- Yan , P , Xie , Y , Qian , YT and Liu , XM . 1999 . A cluster growth route to quantum-confined CdS nanowires . Chem. Commun. , : 1293
- Deng , ZX , Wang , C , Sun , XM and Li , YD . 2002 . Structure-directing coordination template effect of ethylenediamine in formations of ZnS and ZnSe nanocrystallites via solvothermal route . Inorg. Chem. , 41 : 869
- Manna , L , Scher , EC and Alivisators , AP . 2000 . Synthesis of soluble and processable rod-, arrow-, teardrop-, and tetrapod-shaped CdSe nanocrystals . J. Am. Chem. Soc. , 122 : 12700
- Qing , Y , Tang , KB , Wang , Ch.R. , Qian , YT and Zhang , Sh.Y. 2002 . PVA-assisted synthesis and characterization of CdSe and CdTe nanowires . J. Phys. Chem. B , 106 : 9227
- Chen , CC , Chao , CY and Lang , ZH . 2000 . Simple solution-phase synthesis of soluble CdS and CdSe nanorods . Chem. Mater. , 12 : 1516
- Hardee , KL and Bard , AJ . 1977 . Semiconductor electrodes X photoelectrochemical behavior of several polycrystalline metal oxide electrodes in aqueous solutions . J. Electrochem. Soc. , 124 : 215
- Hara , M , Kondo , T , Komoda , M , Ikeda , S , Shinohara , K , Tanaka , A , Kondo , JN and Domen , K . 1998 . Cu2O as a photocatalyst for overall water splitting under visible light irradiation . Chem. Commun. , : 357
- Nakayama , S , Kimura , A , Shibata , M , Kuwabata , S and Osakai , T . 2001 . Voltammetric characterization of oxide films formed on copper in air . J. Electrochem. Soc. , 148 : B467
- Nagase , K , Zhang , Y , Kodama , Y and Kakuta , J . 1999 . Dynamic study of the oxidation state of copper in the course of carbon monoxide oxidation over powdered CuO and Cu2O . J. Catal. , 187 : 123
- Xu , JF , Ji , W , Shen , ZX , Tang , SH , Ye , XR , Jia , DZ and Xin , XQ . 1999 . Preparation and characterization of CuO nanocrystals . J. Solid State Chem. , 147 : 516
- Xu , JF , Ji , W , Shen , ZX , Li , WS , Tang , SH , Ye , XR , Jia , DZ and Xin , XQ . 1999 . Raman spectra of CuO nanocrystals . J. Raman Spectrosc. , 30 : 413
- Wang , W , Zhan , Y , Wang , X , Liu , Y , Zheng , C and Wang , G . 2002 . Synthesis and characterization of CuO nanowhiskers by a novel one-step, solid-state reaction in the presence of a nonionic surfactant . Mater. Res. Bull. , 37 : 1093
- Sun , JH , Gong , YJ , Fan , WH , Wu , D and Sun , YH . 2000 . Chem. J. Chin. Univ. , 21 : 95
- Wang , W , Liu , Z , Liu , Y , Xu , C , Zheng , C and Wang , G . 2003 . A simple wet-chemical synthesis and characterization of CuO nanorods . Appl. Phys. A , 76 : 417
- 1991 . Joint Committee on Powder Diffraction Standards: diffraction Data File Pennsylvania No. 5–661, JCPDS International Center for Diffraction Data
- Gao , XP , Bao , JL , Pan , GL , Zhu , HY , Huang , PX , Wu , F and Song , DY . 2004 . Preparation and electrochemical performance of polycrystalline and single crystalline CuO nanorods as anode materials for Li ion battery . J. Phys. Chem. B. , 108 : 5547
- Punnoose , A , Magnone , H , Seehra , MS and Bonevich , J . 2001 . Bulk to nanoscale magnetism and exchange bias in CuO nanoparticles . Phy. Rev. B , 64 : 174420
- Ibrahim , MM , Zhao , J and Seehra , MS . 1992 . Determination of the particle size distribution in Fe2O3-based catalyst using magnetometry and X-ray diffraction . J. Mater. Res. , 7 : 1856
- Viano , A , Mishra , SR , Lloyd , R , Losby , J and Gheyi , T . 2003 . Thermal effects on ESR signal evolution in nano and bulk CuO powder . J. Non-crystal. Solids , 325 : 16
- Zhu , CL , Chen , CN , Hao , LY , Hu , Y and Chen , ZY . 2004 . Template-free synthesis of Cu2Cl(OH)3 nanoribbons and use as sacrificial template for CuO nanoribbon . J. Crys. Growth , 263 : 473
- Zhu , CL , Chen , CN , Hao , LY , Hu , Y and Chen , ZY . 2004 . In-situ preparation of 1D CuO nanostructures using Cu2(OH)2CO3 nanoribbons as precursor for sacrifice-template via heat-treatment . Solid State Commun. , 130 : 681
- Song , X , Sun , S , Zhang , W , Yu , H and Fan , W . 2004 . Synthesis of Cu(OH)2 nanowires at aqueous-organic interfaces . J. Phys. Chem. B , 108 : 5200
- Lu , C , Qi , L , Yang , J , Zhang , D , Wu , N and Ma , J . 2004 . Simple template-free solution route for the controlled synthesis of Cu(OH)2 and CuO nanostructures . J. Phys. Chem. B , 108 : 17825
- Chang , Y and Zeng , HC . 2004 . Controlled synthesis and self-assembly of single-crystalline CuO nanorods and nanoribbons . Crys. Growth Des. , 4 : 397
- Liu , B and Zeng , HC . 2004 . Formation of “Dandelions” . J. Am. Chem. Soc. , 126 : 8124
- Yang , R and Gao , L . 2004 . Novel way to synthesize CuO nanocrystals with various morphologies . Chem. Lett. , 33 : 1194
- Baker , ES and Jonas , J . Transport and relaxation properties of dimethyl sulfoxide-water mixtures at high pressure . J. Phys. Chem. , 89 1730 (1985), and the references cited therein
- Nemeth , J , Rodriguez-Gattorno , G , Diaz , D , Vazquez-Olmos , AR and Dekany , I . 2004 . Synthesis of ZnO nanoparticles on a clay mineral surface in dimethyl sulfoxide medium . Langmuir , 20 : 2855
- Xu , CK , Liu , YK , Xu , G and Wang , G . 2002 . Preparation and characterization of CuO nanorods by thermal decomposition of CuC2O4 precursor . Mater. Res. Bull. , 37 : 2365
- Hong , ZS , Cao , Y and Deng , JF . 2002 . A convenient alcohothermal approach for low temperature synthesis of CuO nanoparticles . Mater. Lett. , 52 : 34
- Wang , H , Xu , JZ , Zhu , JJ and Chen , HY . 2002 . Preparation of CuO nanoparticles by microwave irradiation . J. Crys. Growth , 244 : 88
- Iwasaki , M and Ito , S . 1997 . New route to prepare ultrafine ZnO particles and its reaction mechanism . J. Mater. Sci. Lett. , 16 : 1503
- Borgohain , K , Singh , JB , Rama Rao , MV , Shripathi , T and Mahamumi , S . 2000 . Quantum size effects in CuO nanoparticles . Phy. Rev. B. , 61 : 11093
- Lo , CH , Tsung , TT , Chen , LC , Su , CH and Lin , HM . 2005 . Fabrication of copper oxide nanofluid using submerged arc nanoparticle synthesis system (SANSS) . J. Nanopar. Res. , 7 : 313
- Jiang , X , Herricks , T and Xia , Y . 2002 . CuO nanowires can be synthesized by heating copper substrate in air . Nano Lett. , 2 : 1333
- Adegboyega , GA . 1990 . Preparation and characterization of thermally oxidized copper substrates for photothermal and photovoltaic energy conversion . Niger, J. Renew. Energy , 1 : 21
- Yu , T , Zhao , X , Shen , ZX , Wu , YH and Su , WH . 2004 . Investigation of individual CuO nanorods by polarized micro-Raman scattering . J. Crys. Growth , 268 : 590
- Huang , LS , Yang , SG , Li , T , Gu , BX , Du , YW , Lu , YN and Shi , SZ . 2004 . Preparation of large-scale cupric oxide nanowires by thermal evaporation method . J. Crys. Growth , 260 : 130
- Wen , XG , Zhang , WX and Yang , SH . 2003 . Synthesis of Cu(OH)2 and CuO nanoribbon arrays on a copper substrate . Langmuir , 19 : 5898
- Anandan , S , Wen , XG and Yang , SH . 2005 . Room temperature growth of CuO nanorod arrays on copper and their application as a cathode in dye-sensitized solar cells . Mater. Chem. Phys. , 93 : 35