ABSTRACT
To conveniently prepare the immobilized enzyme for green synthesis of vitamin A palmitate, thermomyces lanuginosus lipase (TLL) was assembled with apatite derived from calcium phosphate in solution to form TLL@apatite hybrid nanoflowers (hNFs) by mimetic biomineralization. The obtained hNFs was characterized by SEM and applied in the enzymatic synthesis of vitamin A palmitate. Under optimal condition, when palmitic acid and vitamin A acetate were used as substrates, the yield of the target product was up to 90.4%, about 1.5 folds of that using free TLL. In addition, when each cycle for catalytic synthesis was carried out in petroleum ether with 20% water content as solvent for 12 h, the hNFs still retains 65.8% of initial catalytic synthetic ability after 10 cycles. These results demonstrated the hNFs was a kind of green biocatalyst with high catalytic activity and high stability with great potential in the green pharmaceuticals industry.
GRAPHICAL ABSTRACT
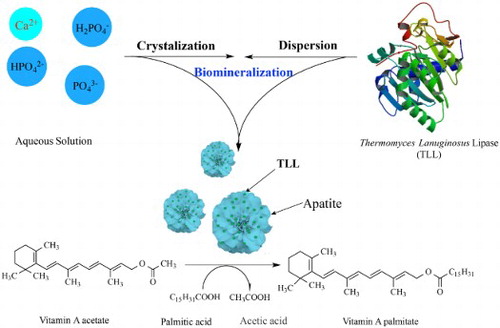
1. Introduction
Vitamin A is an essential nutrient that is important for the growth and maturation of organs and bones, the enhancement of the immune system, and the development and maintenance of eye health and night vision. According to the report by the World Health Organization (WHO), 190 million preschool children (under 5 years of age) and 19.1 million pregnant women are under-concentrated in vitamin A ( Citation1), and severe vitamin deficiencies can lead to visual impairment (night blindness), anemia, reduced resistance to infection, etc. Therefore, The WHO recommend maintaining a policy of universal supplementation for children under 5 years of age among those at risk of vitamin A deficiency (VAD) ( Citation2). Lots and lots of researchers are working to solve the thorny problems caused by VAD ( Citation3, Citation4). Penkert et al. have proposed to treat respiratory disease using vitamin A vaccine in school-age children ( Citation5). Moreover, WHO also have proposed some strategies to prevent and treat the problem such as fortification of foods, vitamin A supplement, etc. However, the main technical barrier to the fortification of food with vitamin A is its susceptibility to oxidation and isomerization, which result in loss of nutritional efficacy ( Citation6). When this vitamin is exposed to light, high temperature and in the presence of oxygen, a high number of unsaturated bonds render it prone to oxidation which results in the degradation ( Citation7).
Now, vitamin A is usually present in a more stable esterified form with palmitic acid or acetic acid, vitamin A palmitate wins with its higher low-temperature stability and easier storage ( Citation8). Chemical synthesis vitamin A palmitate suffer from disadvantages such as low selectivity or non-selectivity of esterification site, high protection requirements, difficult purification of deprotection steps, and that use of highly corrosive acids include hydrogen fluoride or sulfuric acid as catalysts during esterification, which leads to a series of drawbacks such as formation and high energy consumption along with many by-products ( Citation9). Moreover, most reported efficient acid and base catalysts are corrosive and environmentally unfriendly. Compared with chemical method, enzymatic synthesis of fine chemicals and medicine has been attracting great attention in recent years because of its mild reaction condition, higher catalytic efficiency and the inherent selectivity ( Citation10–12). Of course, in biocatalysis, the immobilized enzymes have various advantages in continuous practice ( Citation13) and are attracting more attentions than the free one. For example, the immobilized Candida antarctica lipase B (CALB) has been used to catalyze the synthesis of vitamin A palmitate ( Citation14).
However, in the preparation of the immobilized enzyme, there is a pity that most of the used carriers including organic materials ( Citation15–18) and inorganics ( Citation19–21) could not be regenerated and reused, and the loss of catalytic activity often occurs to enzymes in the immobilization process ( Citation22). Recently, Zare and his colleagues firstly have reported a facile method to prepare the immobilized enzyme with highly enhanced activity which are the enzyme-inorganic hybrid nanoflowers (hNFs) comprising copper phosphate (Cu3(PO4)2) and enzyme ( Citation23). The obtained laccase nanoflowers exhibit about 650% increase in activity (in terms of oxidizing syringaldazine) compared with free laccase in solution. Nevertheless, the copper ion belongs to heavy metal ion that could have extensive detrimental effects on humans and the environment due to their enhanced reactivity and high density ( Citation24, Citation25) such as copper-induced metal lothioneins (MTs) through conjugation to “metallothionein-like protein” (MTLP). On the contrary, calcium ion is the most biocompatible for its abundance in the bone of living organisms and various foods such as milk, peanuts, and beans. Thus, calcium ion is more environment-friendly and greener than other metal ions in catalytic reactions. Ke et al. ( Citation26) have described a new hybrid nanoflower using the organic component of Burkholderia cepacia lipase and an inorganic component of calcium phosphate, and the activity was 308% folds of the free one. In addition, in our previous work, we have also developed a sustainable dual-cycle method depending on calcium phosphate crystallization, dissolution and recrystallization, to reuse both enzyme and calcium phosphate support by re-blossoming enzyme-inorganic hybrid nanoflowers ( Citation27).
In fact, such co-assembly and co-crystalization of protein and inorganics to form complex structures, named as biomineralization, are widely found in the living tissue such as bone and teeth, and shells ( Citation28). Now, biomineralization has been developed as a facile method to prepare biomimetic materials ( Citation29–31) such as artificial bone and to recover the rare earth elements ( Citation32). In bone, teeth, and shells, the formation of apatite crystals is preceded by an amorphous calcium phosphate (ACP) precursor phase. ACP formation is thought to proceed through prenucleation clusters-stable clusters that are present in solution already before nucleation ( Citation31). The self-assembly of apatite and proteins is a critical process to induce the formation of the bones and teeth in vertebrates ( Citation29). In this work, TLL was assembled with apatite derived from calcium phosphate at low temperature to form TLL@apatite hNFs by mimetic biomineralization and vitamin A palmitate was synthesized using the obtained hNFs as green catalyst. Effects of reaction conditions were investigated such as reaction temperature, medium and content of water, respectively. The reusability of the TLL@apatite hNFs was also examined in the synthesis of vitamin palmitate under mild conditions.
2. Materials and methods
2.1 Materials and chemicals
Palmitic acid and Vitamin A acetate was purchased from Saen chemical technology (Shanghai) Co. Ltd. 4-nitrophenyl acetate was purchased from Alfa Aesar chemistry Lt. D (Tianjin).Vitamin A palmitate was purchased from Beijing bailingwei technology Co. Ltd and thermomyces lanuginosus lipase (TLL) was purchased from Novozymes. All other chemicals were provided by Sinopharm Chemical Reagent Lt. D (Shanghai).
2.2 Preparation of TLL@apatite hybrid nanoflowers
The nanoflowers were synthesized in typical experiments: 100 mL CaCl2 (200 mM) was added to 5 mL of a phosphate buffered saline (PBS) solution (20 mM) containing 24 mg/mL TLL at pH 6.7, and the mixture was kept at low temperature (4°C) for 24 h. In the following experiments, the conditions remained the same as in typical experiments, unless otherwise indicated. After 24 hours, the mixture was removed and transferred to a centrifuge tube, centrifuged at 4°C and 10,000 rpm for 10 min. The liquid was drained to obtain TLL@apatite hNFs (seen ).
2.3 Synthesis of vitamin A palmitate catalyzed by TLL@apatite hNFs
Vitamin A palmitate was synthesized by transesterification of vitamin A acetate, and palmitic acid. Typically, vitamin A acetate (0.3 mmol) and Palmitic acid (0.9 mmol) were first dissolved in a mixed solvent of 8 mL of petroleum ether (PE) and 2 mL of distilled water, and then TLL@apatite hNFs (containing 20 mg TLL) was added to start the reaction at 35°C in a shaking incubator with an agitation speed of 200 rpm for 12 hours (). The enzymatic process was carried out in a temperature-controlled incubator shaken at 200 rpm for a certain period and the content of the target product in the reaction mixture was monitored by HPLC.
2.4 Characterization of enzyme-inorganic hybrid nanoflowers and vitamin A palmitate
Scanning electron microscope (SEM) (S-4800, HITACHI) was employed to examine the morphology of hNFs.1H NMR spectra and 13CNMR were recorded through a Brucker DPX 300 spectrometer at 500 MHz and 126 MHz, respectively. NMR experiment was performed in deuterated chloroform (CDCl3) containing a proper concentration of the sample, a total of 128 scans was recorded. Data were collected and analyzed through MestReNova software. Proton chemical shifts were referenced to tetramethylsilane (TMS) at 0.00 pm. High-Performance Liquid Chromatography (HPLC) (Agilent, 1100 series) was employed to analyze the nature of the product.
3. Results and discussion
3.1 The characterization of TLL@apatite hNFs
The samples were synthesized using PBS (20 mM, pH 6.7) containing 0.25 mg/mL enzyme at 4°C for 24 h and SEM was used to examine the morphology and structure of the obtained TLL@apatite hNFs. As shown in , the SEM image of the lipase-hNFs () had the flower-shape. The results displayed that the morphology of the TLL@apatite hNFs was more like flower than the pictures of the Burkholderia cepacia lipase (BCL) ( Citation26) and the petals can be seen more obviously. This different morphology as SEM images of hNFs showed may probably attributed to the different nucleation, growth, structure and orientation of apatite ( Citation33), which derived from the various binding sites of carboxyl and amino groups with Ca2+ cation and [PO4]3−, respectively ( Citation34–36). When TLL proteins dispersed in the aqueous solution containing Ca2+ cation, [PO4]3−, [HPO4]2−, and [H2PO4]−, the cations could bind to the carboxyl groups such as Asp and the anions to amino groups such as Lys ( Citation36, Citation37). Both the two type of binding sites all could function as the prenucleation and nucleation sites to perform the self-assembly of protein and apatite and further form the hNFs.
3.2 Effect of substrate molar ratio on vitamin A palmitate
Effect of substrate molar ratio on the yield of vitamin A palmitate was also investigated in our work. As shown in , the yield of target product catalyzed using free enzyme reached the highest when the molar ratio of substrates is 1:3. However, in the TLL@apatite hNFs catalyzed reaction, when the molar ratio of was 1:2, the yield can also be up to 90.4%, very close to that from the molar ratio of 1:3. This indicated that the amount of palmitic acid could be reduced by one-third using hNFs catalyst compared with free TLL. In addition, under different conditions of molar ratios, the yield of products using hNFs catalyst was always higher than that of free enzyme. The characterization of the product was shown in the supporting information (see Figure S1–Figure S3).
3.3 Effect of reaction medium on yield of vitamin A palmitate
The effect of medium on the yield of vitamin A palmitate is shown in . According to the yield in the different media, petroleum ether was found to be suitable for the enzymatic synthesis of the target product. When the ratio of PE (V/V) to water was 8:2, the yield catalyzed using TLL@apatite hNFs was up to 90.39%, about 1.5 folds of that using free TLL (61.31%). Moreover, the yield in this medium was not only higher than that in N, N-Dimethylformamide (DMF) but also less toxic. In the synthesis of vitamin A palmitate, vitamin A acetate and palmitic acid are hydrophobic with low polarity. When DMF was used as a medium, it can present good solubility and the yield of product can also reach a certain level for hNFs catalysis. However, DMF is highly harmful to environment and toxic to human body and should be used with caution. Among the remaining solvents, n-hexane was also a slightly suitable medium for higher catalytic property for hNFs, and this solvent with less polarity may also be a good reaction medium.
3.4 Effect of water content on yield of vitamin A palmitate
shows that appropriate content of water in the aqueous-PE medium is advantageous for the enzymatic synthesis of vitamin A palmitate catalyzed by TLL preparations. When the water content is 20% (V/V), both free TLL and hNFs presented the highest catalytic synthetic activity to yield the vitamin A palmitate. The yield of the target product using hNFs as biocatalyst was 88.3% when the water content was 20%, 2.4 folds of that in full aqueous medium. In our work, calcium phosphate and hydroxyapatite around enzyme in the hNFs are hydrophilic and they might form multiple hydrogen bonds with enzyme protein and mimic the activating effect of water, then loosen up the structure of enzyme and activate enzyme ( Citation38) in the alkane solvent of high concentration. In addition, the higher content of PE was more suitable than the lower one for the TLL catalytic hydrolysis in the water-PE system. For the polar solvent such as water, the polarity in the system increased as the elevation of the content and the high polarity would lead to the denaturation of enzyme protein ( Citation39, Citation40). However, the hNFs were supposed to create macromolecular crowding by their nanopores which is usually found in a cell ( Citation41, Citation42), which could accelerate the refolding of enzyme protein ( Citation43, Citation44) and increase the enzymatic activity ( Citation45, Citation46).
3.5 Effect of reaction temperature on vitamin A palmitate yield in enzymatic synthesis
shows the effect of reaction temperature on the yield of vitamin A palmitate. There is no doubt that temperature has a significant effect on the conversion. At 35°C, the optimum product yield reached 90.4% when TLL@apatite hNFs was used as catalyst, about 1.5 folds of that catalyzed by TLL. Though TLL is a thermophilic enzyme, temperature between 30 and 40°C is suitable for its catalysis for long time. When the temperature rises to more than 40°C, the yield of product using free TLL decreased as temperature elevated. On the contrary, the hNFs present higher catalytic synthetic activity, which resulted from their higher stability at high temperature. The catalytic activity of hNFs was 70.1%, 3.8 times higher than that of free TLL at 70°C. Moreover, At 80°C, free TLL loses full of its activity, however, hNFs still remains its most of the initial catalytic synthetic activity and the yield of product was up to 69.5%. The comparison of activity between TLL and hNFs in the synthesis of vitamin A palmitate was agreement with the previous report about their thermal stability in hydrolyzing 4-Nitrophenyl acetate ( Citation27).
3.6 Effect of reaction time on vitamin A palmitate yield
Effect of reaction time on vitamin A palmitate yield was determined by performing reaction at 30°C for the different time period. The results are shown in indicates that the yield of target product increased as the reaction time went whether the catalyst was TLL or TLL@apatite hNFs. After 12 hours of reaction, the yield was up to 90.4%. Even if reaction time went towards, the yield was not found to change clearly. In addition, the yields of product using the hNFs were always higher than that using free TLL, which may be resulted from the lower thermal stability of free TLL than the hNFs ( Citation27). Free TLL would lose its most activity within the long period of reaction time and could not catalyze the transesterification of vitamin A acetate and palmitic acid any longer. However, the hNFs along with high thermal stability could tolerate the temperature environment in the reaction and remain its catalytic activity well. Moreover, the highest catalytic efficiency (Kcat/Km) of the hNFs was 38.52 mM−1 s−1 in the enzyme activity assay, 21.7 folds than that of free enzyme ( Citation27). Moreover, the strong interaction of much Ca2+ ions in the hNFs with the carboxyl groups of substrates would enrich the substrate in the inner compact layer and near diffusion layer of the hNFs and further improve the reaction rate and the yield of product.
3.7 Reusability of TLL@apatite hNFs in the synthesis of vitamin A palmitate
presents the catalytic performance of TLL@apatite hNFs for its reusability. Every cycle was carried out for 12 h in a PE to water ratio of 8:2, and that initial yield was set at 100%. After 11 cycles, the catalytic yield was still as high as 65.8% of the initial yield, which was higher than that of the first feeding reaction of free enzyme. Moreover, it was found that the catalytic synthetic activity of TLL@apatite hNFs remained 85.2% of its initial activity after 8 cycles. However, the immobilized lipase CALB after 8 cycles remained only 81.9% ( Citation47) and about 63.0% ( Citation14) of its initial activity in the same catalytic synthesis of vitamin A palmitate with each cycle of only 6 h. The results show that the obtained TLL@apatite hNFs presents a good reusability in the catalytic synthesis of vitamin A palmitate. In the pilot study of the catalytic synthesis, TLL@apatite hNFs was separated from the reaction mixture by centrifugation, which would result in the loss of some hNFs and decrease of the observed catalytic activity. In the coming large-scale synthesis of the vitamin A palmitate, reactor combining with the hollow fiber membrane will be used and expected to overcoming the above problem.
4. Conclusion
In summary, we have developed a facile method to assemble TLL with apatite to form TLL@apatite hNFs to achieve the immobilized TLL and catalyze the synthesis of vitamin A palmitate under mild condition. Confinement from the apatite promotes can improve the catalytic activity of the immobilized enzyme due to macromolecular crowding. Moreover, the hNFs immobilized enzyme with enhanced stability can be used in the synthesis of vitamin A palmitate for catalytic cycles, which would decrease the cost of the synthesis of the compound. Furthermore, biocompatible calcium ions are harmless to the environment and human body unlike other heavy metals, and both Ca2+ and [PO4]3− in the hNFs can also be recovered and reused. The prepared biocatalyst using self-assembly of enzyme and biocompatible inorganic components is environment-friendly and greener, and can be used in the synthesis of other chemical compounds of high value in pharmaceutical, foodstuff, cosmetics and fine chemicals industries.
Supplemental_Material.docx
Download MS Word (419.7 KB)Disclosure statement
No potential conflict of interest was reported by the authors.
Notes on contributors
Liqing Xu is studying in College of Materials, Chemistry and Chemical Engineering (Hangzhou Normal University, Hangzhou, China). Her research has focused on the biocatalysis and biotransformation.
Jianyun Yu, female, born in 1992, is studying as a Master candidate in College of Materials, Chemistry and Chemical Engineering (Hangzhou Normal University, Hangzhou, China). Her research is focused on the enzyme immobilization.
Anming Wang, male, born in 1975, associated professor. He is working in College of Materials, Chemistry and Chemical Engineering (Hangzhou Normal University, Hangzhou, China). His research interest is biocatalysis and green chemical engineering, mainly engaged in the enzyme immobilization and its catalysis application in the synthesis and development of chiral drugs.
Chengyi Zuo is studying in College of Materials, Chemistry and Chemical Engineering (Hangzhou Normal University, Hangzhou, China). Her research has focused on the biocatalysis and biotransformation.
Huimin Li is studying as a Master candidate in College of Materials, Chemistry and Chemical Engineering (Hangzhou Normal University, Hangzhou, China). Her research has focused on the biocatalysis and biotransformation.
Xinxin Chen is studying as a Master candidate in College of Materials, Chemistry and Chemical Engineering (Hangzhou Normal University, Hangzhou, China). Her research has focused on the biocatalysis and biotransformation.
Xiaolin Pei, male, born in 1982, associated professor. He is working in College of Materials, Chemistry and Chemical Engineering (Hangzhou Normal University, Hangzhou, China). His research interest is biotechnology and bioengineering, mainly engaged in the directed evolution of enzymes and its catalysis application in the synthesis of chiral drugs.
Pengfei Zhang, male, born in 1965, professor. He obtained his Ph.D. degree in 2003. Recently, his research has focused on the synthesis of chiral compounds, green chemistry and medicinal chemistry.
Additional information
Funding
References
- Saeterdal, I.; Mora, J.O.; De-Regil, L.M. Fortification of Staple Foods with Vitamin A for Preventing Vitamin A Deficiency. Cochrane Database Syst Rev 2012. Art. No.: CD010068. doi:10.1002/14651858.CD010068.
- Imdad, A.; Mayo-Wilson, E.; Herzer, K.; Bhutta, Z.A. Vitamin A Supplementation for Preventing Morbidity and Mortality in Children From Six Months to Five Years of Age. Cochrane Database Syst Rev 2017, 3. Art. No.: CD008524. doi:10.1002/14651858.CD008524.pub3.
- Singer, J.R.; Bakall, B.; Gordon, G.M.; Reddy, R.K. Treatment of Vitamin A Deficiency Retinopathy with Sublingual Vitamin A Palmitate. Doc Ophthalmol 2016, 132, 137–45. doi: 10.1007/s10633-016-9533-2
- Cui, X.; Xiang, J.; Zhu, W.; Wei, A.; Le, Q.; Xu, J.; Zhou, X. Vitamin A Palmitate and Carbomer Gel Protects the Conjunctiva of Patients With Long-Term Prostaglandin Analogs Application. J Glaucoma 2016, 25, 487–92. doi: 10.1097/IJG.0000000000000316
- Penkert, R.R.; Iverson, A.; Rosch, J.W.; Hurwitz, J.L. Prevnar-13 Vaccine Failure in a Mouse Model for Vitamin A Deficiency. Vaccine 2017, 35, 6264–6268. doi: 10.1016/j.vaccine.2017.09.069
- Loveday, S.M.; Singh, H. Recent Advances in Technologies for Vitamin A Protection in Foods. Trends Food Sci. Technol 2008, 19, 657–668. doi: 10.1016/j.tifs.2008.08.002
- Moccand, C.; Martin, F.; Martiel, I.; Gancel, C.; Michel, M.; Fries, L.; Sagalowicz, L. Vitamin A Degradation in Triglycerides Varying by Their Saturation Levels. Food Res Int 2016, 88, 3–9. doi: 10.1016/j.foodres.2016.06.001
- Zhao, H.X.; Cui, F.J.; Li, Y.H.; Sun, W.J.; Zhou, Q.; Yu, S.L.; Dong, Y.; Zong, L.; Huang, H. Research Progress of Vitamin Esters Derivatives Synthesis. China Food Addit. 2013, 6, 176–183.
- Liu, T.; Yin, C.H.; Tan, T.W. Lipase Catalyzed Synthesis of Vitamin A Esters. Mod. Chem. Ind 2005, 25, 37–40.
- Muthusamy, K.; Sridharan, V.; Maheswari, C.U.; Nagarajan, S. Lipase Catalyzed Synthesis of Fluorescent Glycolipids: Gelation Studies and Graphene Incorporated Self-Assembled Sheet Formation for Semiconductor Applications. Green Chem 2016, 18, 3722–3731. doi: 10.1039/C6GC00347H
- Dwivedee, B.P.; Ghosh, S.; Bhaumik, J.; Banoth, L.; Chand Banerjee, U. Lipase-catalyzed Green Synthesis of Enantiopure Atenolol. RSC Adv 2015, 5, 15850–15860. doi: 10.1039/C4RA16365F
- Banerjee, R.; Proshlyakov, Y.; Lipscomb, J.D.; Proshlyakov, D.A. Structure of the Key Species in the Enzymatic Oxidation of Methane to Methanol. Nature 2015, 518, 431–4. doi: 10.1038/nature14160
- Zheng, L.; Xie, X.; Wang, Z.; Zhang, Y.; Wang, L.; Cui, X.; Huang, H.; Zhuang, H. Fabrication of a Nano-Biocatalyst for Regioselective Acylation of Arbutin. Green Chem. Lett. Rev 2018, 11, 55–61. doi: 10.1080/17518253.2018.1437226
- Yao, C.; Lin, W.; Yue, K.; Ling, X.; Jing, K.; Lu, Y.; Tang, S.; Fan, E. Biocatalytic Synthesis of Vitamin A Palmitate Using Immobilized Lipase Produced by Recombinant Pichia Pastoris. Eng. Life Sci 2017, 17, 768–774. doi: 10.1002/elsc.201600178
- Barbosa, O.; Ortiz, C.; Berenguer-Murcia, A.; Torres, R.; Rodrigues, R.C.; Fernandez-Lafuente, R. Strategies for the one-Step Immobilization-Purification of Enzymes as Industrial Biocatalysts. Biotechnol. Adv 2015, 33, 435–456. doi: 10.1016/j.biotechadv.2015.03.006
- Chen, L.Y.; Luque, R.; Li, Y.W. Controllable Design of Tunable Nanostructures Inside Metal-Organic Frameworks. Chem. Soc. Rev 2017, 46, 4614–4630. doi: 10.1039/C6CS00537C
- Lian, X.Z.; Fang, Y.; Joseph, E.; Wang, Q.; Li, J.L.; Banerjee, S.; Lollar, C.; Wang, X.; Zhou, H.C. Enzyme-MOF (Metal-Organic Framework) Composites. Chem Soc Rev 2017, 46, 3386–3401. doi: 10.1039/C7CS00058H
- Sheldon, R.A.; van Pelt, S. Enzyme Immobilisation in Biocatalysis: Why, What and How. Chem Soc Rev 2013, 42, 6223–6235. doi: 10.1039/C3CS60075K
- Massaro, M.; Lazzara, G.; Milioto, S.; Noto, R.; Riela, S. Covalently Modified Halloysite Clay Nanotubes: Synthesis, Properties, Biological and Medical Applications. J Mater Chem B 2017, 5, 2867–2882. doi: 10.1039/C7TB00316A
- Song, G.S.; Chen, Y.Y.; Liang, C.; Yi, X.; Liu, J.J.; Sun, X.Q.; Shen, S.D.; Yang, K.; Liu, Z. Catalase-Loaded TaOx Nanoshells as Bio-Nanoreactors Combining High-Z Element and Enzyme Delivery for Enhancing Radiotherapy. Adv. Mater 2016, 28, 7143–7148. doi: 10.1002/adma.201602111
- Tully, J.; Yendluri, R.; Lvov, Y. Halloysite Clay Nanotubes for Enzyme Immobilization. Biomacromolecules 2016, 17, 615–621. doi: 10.1021/acs.biomac.5b01542
- Van Langen, L.M.; Janssen, M.H.; Oosthoek, N.H.; Pereira, S.R.; Svedas, V.K.; van Rantwijk, F.; Sheldon, R.A. Active Site Titration as a Tool for the Evaluation of Immobilization Procedures of Penicillin Acylase. Biotechnol Bioeng 2002, 79, 224–228. doi: 10.1002/bit.10280
- Ge, J.; Lei, J.; Zare, R.N. Protein-inorganic Hybrid Nanoflowers. Nat Nanotechnol 2012, 7, 428–32. doi: 10.1038/nnano.2012.80
- Kumar, P.; Kim, K.-H.; Bansal, V.; Lazarides, T.; Kumar, N. Progress in the Sensing Techniques for Heavy Metal Ions Using Nanomaterials. J Ind Eng Chem 2017, 54, 30–43. doi: 10.1016/j.jiec.2017.06.010
- Liu, S.J.; Guo, Y.P.; Yang, H.Y.; Wang, S.; Ding, H.; Qi, Y. Synthesis of a Water-Soluble Thiourea-Formaldehyde (WTF) Resin and its Application to Immobilize the Heavy Metal in MSWI fly ash. J Environ Manage 2016, 182, 328–334. doi: 10.1016/j.jenvman.2016.07.086
- Ke, C.; Fan, Y.; Chen, Y.; Xu, L.; Yan, Y. A New Lipase–Inorganic Hybrid Nanoflower with Enhanced Enzyme Activity. RSC Adv 2016, 6, 19413–19416. doi: 10.1039/C6RA01564F
- Yu, J.; Wang, C.; Wang, A.; Li, N.; Chen, X.; Pei, X.; Zhang, P.; Wu, S.G. Dual-cycle Immobilization to Reuse Both Enzyme and Support by Reblossoming Enzyme–Inorganic Hybrid Nanoflowers. RSC Adv 2018, 8, 16088–16094. doi: 10.1039/C8RA02051E
- Nudelman, F.; Sommerdijk, N.A. Biomineralization as an Inspiration for Materials Chemistry. Angew Chem, Int Ed Engl 2012, 51, 6582–96. doi: 10.1002/anie.201106715
- Ma, J.; Wang, J.; Ai, X.; Zhang, S. Biomimetic Self-Assembly of Apatite Hybrid Materials: From a Single Molecular Template to bi-/Multi-Molecular Templates. Biotechnol Adv 2014, 32, 744–760. doi: 10.1016/j.biotechadv.2013.10.014
- Li, C.; Born, A.-K.; Schweizer, T.; Zenobi-Wong, M.; Cerruti, M.; Mezzenga, R. Amyloid-Hydroxyapatite Bone Biomimetic Composites. Adv.Mater 2014, 26, 3207–3212. doi: 10.1002/adma.201306198
- Dey, A.; Bomans, P.H.H.; Müller, F.A.; Will, J.; Frederik, P.M.; de With, G.; Sommerdijk, N.A.J.M. The Role of Prenucleation Clusters in Surface-Induced Calcium Phosphate Crystallization. Nat. Mater 2010, 9, 1010–1014. doi: 10.1038/nmat2900
- Hatanaka, T.; Matsugami, A.; Nonaka, T.; Takagi, H.; Hayashi, F.; Tani, T.; Ishida, N. Rationally Designed Mineralization for Selective Recovery of the Rare Earth Elements. Nat Commun 2017, 8, 15670. doi: 10.1038/ncomms15670
- Wang, Y.; Azaïs, T.; Robin, M.; Vallée, A.; Catania, C.; Legriel, P.; Pehau-Arnaudet, G.; Babonneau, F.; Giraud-Guille, M.-M.; Nassif, N. The Predominant Role of Collagen in the Nucleation, Growth, Structure and Orientation of Bone Apatite. Nat. Mater 2012, 11, 724–733. doi: 10.1038/nmat3362
- Smeets, P.J.M.; Cho, K.R.; Kempen, R.G.E.; Sommerdijk, N.A.J.M.; De Yoreo, J.J. Calcium Carbonate Nucleation Driven by Ion Binding in a Biomimetic Matrix Revealed by in Situ Electron Microscopy. Nat. Mater 2015, 14, 394–399. doi: 10.1038/nmat4193
- Xiao, Z.; Que, K.; Wang, H.; An, R.; Chen, Z.; Qiu, Z.; Lin, M.; Song, J.; Yang, J.; Lu, D.; Shen, M.; Guan, B.; Wang, Y.; Deng, X.; Yang, X.; Cai, Q.; Deng, J.; Ma, L.; Zhang, X.; Zhang, X. Rapid Biomimetic Remineralization of the Demineralized Enamel Surface Using Nano-Particles of Amorphous Calcium Phosphate Guided by Chimaeric Peptides. Dent Mater 2017, 33, 1217–1228. doi: 10.1016/j.dental.2017.07.015
- Wu, L.N.; Genge, B.R.; Wuthier, R.E. Analysis and Molecular Modeling of the Formation, Structure, and Activity of the Phosphatidylserine-Calcium-Phosphate Complex Associated with Biomineralization. J Biol Chem 2008, 283, 3827–38. doi: 10.1074/jbc.M707653200
- Kim, D.; Lee, B.; Thomopoulos, S.; Jun, Y.S. The Role of Confined Collagen Geometry in Decreasing Nucleation Energy Barriers to Intrafibrillar Mineralization. Nat Commun 2018, 9, 962. doi: 10.1038/s41467-018-03041-1
- Klibanov, A.M. Improving Enzymes by Using Them in Organic Solvents. Nature 2001, 409, 241–246. doi: 10.1038/35051719
- Wang, Q.G.; Gao, Q.M.; Shi, J.L. Enhanced Catalytic Activity of Hemoglobin in Organic Solvents by Layered Titanate Immobilization. J Am Chem Soc 2004, 126, 14346–14347. doi: 10.1021/ja047224s
- Bindhu, L.V.; Abraham, T.E. Preparation and Kinetic Studies of Surfactant-Horseradish Peroxidase Ion Paired Complex in Organic Media. Biochem Eng J 2003, 15, 47–57. doi: 10.1016/S1369-703X(02)00173-0
- Ellis, R.J.; Minton, A.P. Cell Biology-Join the Crowd. Nature 2003, 425, 27–28. doi: 10.1038/425027a
- Cheung, M.S.; Thirumalai, D. Nanopore-Protein Interactions Dramatically Alter Stability and Yield of the Native State in Restricted Spaces. J. Mol. Biol 2006, 357, 632–643. doi: 10.1016/j.jmb.2005.12.048
- Wang, X.Q.; Lu, D.N.; Austin, R.; Agarwal, A.; Mueller, L.J.; Liu, Z.; Wu, J.Z.; Feng, P.Y. Protein Refolding Assisted by Periodic Mesoporous Organosilicas. Langmuir 2007, 23, 5735–5739. doi: 10.1021/la063507h
- Du, F.; Zhou, Z.; Mo, Z.Y.; Shi, J.Z.; Chen, J.; Liang, Y. Mixed Macromolecular Crowding Accelerates the Refolding of Rabbit Muscle Creatine Kinase: Implications for Protein Folding in Physiological Environments. J. Mol. Biol 2006, 364, 469–482. doi: 10.1016/j.jmb.2006.09.018
- Jiang, M.; Guo, Z.H. Effects of Macromolecular Crowding on the Intrinsic Catalytic Efficiency and Structure of Enterobactin-Specific Isochorismate Synthase. J. Am. Chem. Soc 2007, 129, 730–731. doi: 10.1021/ja065064+
- Moran-Zorzano, M.T.; Viale, A.; Munoz, F.; Alonso-Casajus, N.; Eydallin, G.; Zugasti, B.; Baroja-Fernandez, E.; Pozueta-Romero, J. Escherichia Coli AspP Activity is Enhanced by Macromolecular Crowding and by Both Glucose-1,6-Bisphosphate and Nucleotide-Sugars. FEBS Lett 2007, 581, 1035–1040. doi: 10.1016/j.febslet.2007.02.004
- Liu, Y.; Ni, H.; Cai, H.; Xiao, A.; Su, W. Study on Reaction Conditions for Immobilized Lipase Synthesizing Retinol Palmitate by Transesterification. J. Chin. Inst. Food Sci. Technol. 2012, 12, 75–83.