ABSTRACT
Magnetic nano catalysts are widely used in various organic reactions due to their characteristics of magnetic separation and recycling. Magnetic Fe3O4@SiO2 nano catalyst modified by 1, 8-diazabicyclo [5.4.0]-7-undecene (DBU) was prepared and applied in the Knoevenagel reaction. The results showed that the catalyst has an excellent effect on the Knoevenagel reaction, which not only improves the reaction rate and yield, but also it can be easily separated from the reaction mixture driven by external magnetic force. Furthermore, the catalyst can be recycled 10 cycles with no loose of the catalytic activity.
GRAPHICAL ABSTRACT
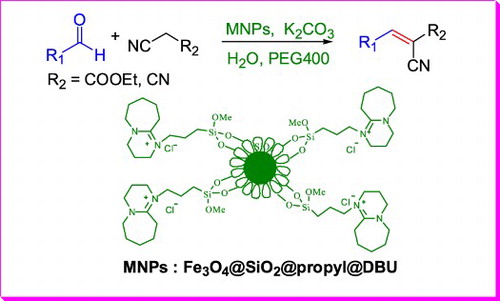
1. Introduction
Knoevenegal reaction is the condensation reaction of a reactive methylene compounds with aldehydes or ketones under weak base conditions, which resulting in α, β- unsaturated carbonyl compounds (Citation1). In recent years, to improve the Knoevenegal reaction, protic ionic liquids (Citation2), mesoporous materials (Citation3), amine-functionalized ionic liquid-based periodic mesoporous organosilica (Citation4), carbamic acid ammonium salt (Citation5), zeolite (Citation6), MOFs (Citation7–13), melamine-derived graphitic carbon nitride (Citation14), molybdenum carbide (Citation15), amines functionalized C60 (Citation16), quinine(Citation17), polyoxometalate (POM) cluster organic frameworks (Citation18–20), Pickering emulsion (Citation21), Fe3O4@ZIF-8 (Citation22) and nanohybrid material (Citation23) have been reported as catalysts to catalyze this reaction. Although these catalysts have good catalytic effect, most of them suffer from harsh recovery conditions, low yield, long reaction time or poor reuse effect. Even to satisfy the need of certain catalysts, some environmentally harmful solvents are often used. Therefore, in line with the concept of green chemistry, it is necessary to develop a green catalyst with high efficiency, easy recovery, and high reuse rate.
In recent years, magnetic nanoparticles have been widely studied as catalysts used for various reactions (Citation24, Citation25). Among them, Maleki’s group developed a series of magnetic nano catalysts, such as SiO2-supported Fe3O4 (Citation26, Citation27), palladium-loaded magnetic material(Citation28), copper-loaded magnetic agar (Cu2O/Agar@Fe3O4) (Citation29), cellulose-based magnetic nanocomposite (Citation30), biological macromolecule chitosan magnetic nanometer catalyst (Citation31), chromium-based magnetic composite nano catalyst (Citation32) and the Fe3O4@SiO2-OSO3H catalyst (Citation33), which were used in the synthesis of heterocyclic compounds, α-aminonitriles and the oxidation reaction of alcohols, excellent catalytic effects were obtained. In addition, Zhang’s group developed the molybdenum-supported graphene oxid/Fe3O4 for the construction of spiro-oxindole dihydropyridines (Citation34). Gao’s group demonstrated the synthesis of cyclohexanone derivatives catalyzed by a magnetic metal organic framework material (Citation35). All these catalysts not only have the advantages of easy separation and excellent catalytic effect, but also most of the magnetic nano catalysts are supported by Fe3O4 and carry organic molecules on the surface of the support. The spherical magnetic nanoparticles have a high specific surface, organic molecules can be loaded onto the body surface as much as possible to provide more active sites (Citation36–39). Although some typical magnetic nanoparticle Fe3O4 combining with organic catalyst have been developed and can efficiently catalyze the C–C bond coupling reactions (Citation40–42), the development of magnetic nano catalysts still needs further exploration.
DBU, an organic base with steric hindrance, can efficiently catalyze the Knoevenegal reaction, but the reuse and separation of DBU is difficult (Citation43). To address the above questions and to continue our research interests (Citation44, Citation45), a green and efficient Knoevenegal reaction catalyst, Fe3O4@SiO2 modified by DBU (), was developed and used in the Knoevenegal reaction, the high reaction yield and short reaction times were obtained. Since the catalyst itself was supported by magnetic Fe3O4, it is easy to be separated and retains its original activity after 10 times of cyclic tests.
2. Materials and methods
2.1. Materials and products
All chemical reagents used are analytically pure and have not been further purified. For the NMR spectra of Knoevenagel reaction products, see Supplementary Materials.
2.2. Methods
Transmission electron microscopy (TEM) images were obtained from a FEI TECNAI G20 instrument. The X-ray diffraction (XRD) images were obtained by using an XRD-6100 device with Cu Kα radiation (λ = 0.15418 nm). The infrared spectra (FTIR) were obtained from IR Affinit-IS Fourier Transform Spectrometer by dispersing samples in KBr disks. The thermogravimetric analysis images (TG) were obtained from the thermogravimetric analyzer STA 449F5 operating at 220 V and 16 A in the atmosphere of nitrogen. Scanning electron microscope (SEM) images were performed on FESEM FEI Quanta 400 FEG. The vibrating sample magnetometer (VSM) pattern was carried out with SQUID-VSM (America Quantum Design). Brunner-Emmet-Teller (BET) analysis was recorded by the automatic specific surface area and porosity analyzer TriStar II 3020 (America Micromeritics). 1H NMR (400 MHz) and 13C NMR (101 MHz) spectra were recorded on a Bruker Avance DPX 400 spectrometer using DMSO-d6 or CDCl3 as solvent. The elemental analyses were performed on a Vario EL Elemental Analyzer.
2.3. Preparation of the magnetic nano catalyst
As shown in , Nano Fe3O4 and Fe3O4@SiO2 were prepared by the method of chemical coprecipitation. Owing to the outer layer of Fe3O4@SiO2 provides a suitable graft site for subsequent functionalization, 3-chloropropyl trimethoxysilane was selected to condense with Fe3O4@SiO2 in toluene under nitrogen protection and the product 2 was obtained. Then, under nitrogen protection, the quaternization of DBU by product 2 was conducted in toluene and the final magnetic nano-catalyst 3 was achieved.
2.3.1. Synthesis of magnetic nanoparticles Fe3O4
Magnetic Fe3O4 particles were prepared by the method of coprecipitation (Citation46). FeCl3·6H2O (8.1 g, 30 mmol) and FeCl2·4H2O (3.4 g, 17 mmol) were added into a 250 mL round bottom flask containing 100 mL distilled water. Under the protection of nitrogen, the reaction mixture was stirred at 30°C. When all ingredients have dissolved, 13.7 mL NH3·H2O was quickly added, and the color of solution changed from orange to black rapidly. The solid product was collected and washed with deionized water until the solution remains neutral. Then it was washed repeatedly with acetone and ethanol in turn, the residual solvent was removed by vacuum method and the black crystalline particles Fe3O4 were obtained.
2.3.2. Synthesis of magnetic nanoparticles Fe3O4@SiO2
The surface of Fe3O4 was coated with a layer of silica by sol–gel method (Citation47, Citation48). With the ultrasound assist, the magnetic nanoparticle Fe3O4 was dispersed into 150 mL ethanol and 30 mL water, the mixture was stirred for 30 min. Then the concentrated ammonia water and ethyl n-silicate were added, and the reaction continued to stir at room temperature for 24 h. The resulting magnetic solids were collected by external magnets and washed to neutral by using deionized water, then it was washed with ethanol and dried by rotary evaporation to obtain a gray solid powder.
2.3.3. Synthesis of alkyl modified magnetic nanoparticle
Under the ultrasonic assist, the grey solid powder of Fe3O4@SiO2 (3.0 g) was dispersed in toluene (50 mL), and under the protection of nitrogen, 3-chloropropyl trimethoxysilane (3.0 g) was added, the reaction mixture was stirred at 130°C for 24 h. The resulting magnetic solids were collected by external magnets and washed to neutral with deionized water. Then it was washed with ethanol and dried by rotary evaporation to obtain a brown solid powder Fe3O4@SiO2@propylchloride.
2.3.4. Synthesis of DBU modified magnetic nanoparticles (MNPs)
The brown solid powder Fe3O4@SiO2@propylchloride (2.0 g) was dispersed in toluene (50 mL) with the ultrasound assist, then DBU (2.0 g) was added. Under the protection of N2, the reaction was reflux for 24 h at 130°C. The resulting magnetic nanoparticles were collected by external magnets and washed to neutral with deionized water. The crude product was further purified by ethanol and the target magnetic nano-catalyst MNPs was obtained after vacuum drying.
2.4. General procedure for Knoevenagel reaction
P-chlorobenzaldehyde (0.7 g, 5 mmol) was added the 50 mL round bottom flask containing ethyl cyanoacetate (0.6 mL, 6 mmol), K2CO3 (1.0 g) and MNPs (0.3 g) mixed in the complex solvent (6 mL H2O + 2 mL PEG-400), then the reaction mixture was stirred at 40°C and TLC was used to monitor the reaction. when the substrate was completely consumed or the reaction is in equilibrium, 50 mL water was added, and the MNPs was separated from the reaction system using a permanent magnet, the solid crude product was collected and recrystallized with 90% ethanol, and the almost pure target product was obtained. All desired products were characterized by melting point determination and NMR spectroscopy, the spectral data of all products are listed as follows.
Ethyl-3-(4-chlorophenyl)-2-cyanoacrylate (, Entry 1)
White crystal. 1H NMR (400 MHz, CDCl3) δ 8.18 (s, 1H, CH=), 7.92 (d, J = 8.4 Hz, 2H, ArH), 7.46 (d, J = 8.4 Hz, 2H, ArH), 4.38 (q, J = 7.1 Hz, 2H, CH2), 1.39 (t, J = 7.1 Hz, 3H, CH3). 13C NMR (101 MHz, CDCl3) δ 162.15, 153.27, 139.50, 132.18, 129.89, 129.63, 115.22, 103.53, 62.83, 14.13.
Ethyl-2-cyano-3-(4-nitrophenyl)acrylate (, Entry 2).
Yellow solid. 1H NMR (400 MHz, CDCl3) δ 8.36 (d, J = 8.8 Hz, 2H, ArH), 8.33 (s, H, CH=), 8.16 (d, J = 8.8 Hz, 2H, ArH), 4.45 (q, J = 7.1 Hz, 2H, CH2), 1.44 (t, J = 7.1 Hz, 3H, CH3).
Ethyl-2-cyano-3-phenylacrylate (, Entry 3).
White crystal. 1H NMR (400 MHz, CDCl3) δ 8.26 (s, 1H, CH=), 8.00 (m, 2H, ArH), 7.51 (m, 3H, ArH), 4.41 (q, J = 7.1 Hz, 2H, CH2), 1.41 (t, J = 7.1 Hz, 3H, CH3).
Ethyl-2-cyano-3-(2, 4-dichlorophenyl)acrylate(, Entry 4).
White solid; 1H NMR (400 MHz, CDCl3) δ 8.63 (s, 1H, CH=), 8.23 (d, J = 8.6 Hz, 1H, ArH), 7.55 (d, J = 1.9 Hz, 1H, ArH), 7.42 (d, J = 8.6 Hz, 1H, ArH), 7.29 (s, 2H), 4.43 (q, J = 7.1 Hz, 2H, CH2), 1.43 (t, J = 7.1 Hz, 3H, CH3).
Ethyl-2-cyano-3-(4-cyanophenyl)acrylate (, Entry 5)
White crystal; 1H NMR (400 MHz, CDCl3) δ 8.25 (s, 1H, CH=), 8.07 (d, J = 8.4 Hz, 2H, ArH), 7.80 (d, J = 8.3 Hz, 2H, ArH), 4.42 (q, J = 6.8 Hz, 2H, CH2), 1.42 (t, J = 7.5 Hz, 3H, CH3).
Ethyl-2-cyano-3-(4-hydroxyphenyl)acrylate (, Entry 8)
Yellow solid. 1H NMR (400 MHz, DMSO-d6) δ 10.83 (s, 1H, OH), 8.24 (s, 1H, CH=), 8.00 (d, J = 8.3 Hz, 2H, ArH), 6.95 (d, J = 8.3 Hz, 2H, ArH), 4.29 (q, J = 7.0 Hz, 2H, CH2), 1.29 (t, J = 7.0 Hz, 3H, CH3). 13C NMR (101 MHz, DMSO-d6) δ 163.32, 163.03, 155.10, 134.40, 122.95, 116.88, 116.81, 97.51, 62.38, 14.48.
Ethyl-2-cyano-3-(4-phenylphenyl)acrylate (, Entry 9)
Green solid. 1H NMR (400 MHz, DMSO-d6) δ 8.45 (s, 1H, CH=), 8.17 (d, J = 8.0 Hz, 2H, ArH), 7.93 (d, J = 8.0 Hz, 2H, ArH), 7.80 (d, J = 7.6 Hz, 2H, ArH), 7.54–7.45 (m, 3H, ArH), 4.34 (q, J = 7.0 Hz, 2H, CH2), 1.32 (t, J = 7.0 Hz, 3H, CH3). 13C NMR (101 MHz, DMSO-d6) δ 162.34, 154.87, 145.13, 138.91, 132.03, 130.78, 129.57, 129.11, 127.80, 127.43,116.29, 102.40, 62.80, 14.30. Anal. calcd for C18H15NO2: C, 77.96; H, 5.45; N, 5.05. found: C, 77.98; H, 5.49. N, 4.98.
Ethyl-2-cyano-3-(4-fluorophenyl)acrylate (, Entry 10)
White solid. 1H NMR (400 MHz, CDCl3) δ 8.22 (s, 1H, CH=), 8.05 (t, J = 7.7 Hz, 2H, ArH), 7.21 (t, J = 7.7 Hz, 2H, ArH), 4.39 (q, J = 7.1 Hz, 2H, CH2), 1.41 (t, J = 7.1 Hz, 3H, CH3).
Diethyl-3, 3'-(1, 4-phenylene)-bis(2-cyanoacrylate) (, Entry 11)
White crystalline solid. 1 H-NMR (400 MHz, CDCl3) δ 8.27 (s, 2H, CH=), 8.11 (s, 4H, ArH), 4.42 (q, J = 7.1 Hz, 4H, CH2), 1.42 (t, J = 7.1 Hz, 6H, CH3).
Ethyl-2-cyano-3-(2, 4-dimethoxyphenyl)acrylate (, Entry 12)
Yellow crystal. 1H NMR (400 MHz, DMSO-d6) δ 8.52 (s, 1H, CH=), 8.22 (d, J = 8.9 Hz, 1H, ArH), 6.77 (d, J = 9.0 Hz, 1H, ArH), 6.73 (s, 1H, ArH), 4.29 (q, J = 7.0 Hz, 2H, CH2), 3.91 (d, J = 11.2 Hz, 6H. OCH3), 1.29 (t, J = 7.0 Hz, 3H, CH3). 13C NMR (151 MHz, DMSO-d6) δ 166.07, 163.12, 161.79, 148.15, 130.48, 117.00, 113.16, 107.74, 98.76, 97.81, 62.41, 56.78, 56.40, 14.48.
Ethyl-2-cyano-3-(4-dimethylaminephenyl)acrylate (, Entry 13)
Yellow solid. 1H NMR (400 MHz, DMSO-d6) δ 8.11 (s, 1H,CH=), 7.96 (d, J = 8.6 Hz, 2H,ArH), 6.84 (d, J = 8.6 Hz, 2H,ArH), 4.26 (q, J = 7.0 Hz, 2H,CH2), 3.09 (s, 6H,CH3), 1.28 (t, J = 7.0 Hz, 3H,CH3). 13C NMR (151 MHz, DMSO-d6) δ 169.85, 163.90, 154.57, 154.14, 134.21, 118.72, 117.98, 112.12, 111.47, 92.45, 61.87, 14.57.
Ethyl 2-cyano-3-anthracenylacrylate (, Entry 14)
Yellow solid. 1H NMR (400 MHz, DMSO-d6) δ 9.41 (s, 1H,CH=), 8.89 (s, 1H, ArH), 8.27 (d, J = 7.9 Hz, 2H, ArH), 8.60 (d, J = 8.2 Hz, 2H, ArH), 7.70 (t, J = 7.3 Hz, 4H, ArH), 4.49 (q, J = 6.9 Hz, 2H, CH2), 1.45 (t, J = 6.9 Hz, 3H, CH3). 13C NMR (151 MHz, DMSO-d6) δ 161.17, 155.79, 130.80, 130.44, 129.43, 128.67, 127.78, 126.44, 125.33, 123.82, 114.82, 113.90, 62.98, 14.45.
2-Amino-4-(1-cyano-2-methoxy-2-oxoethyl)-4H-chromene-3-carboxylat (, Entry 15)
White solid. 1H NMR (400 MHz, CDCl3) δ 7.31 (s, 1H, ArH), 7.10 (d, J = 8.4 Hz, 3H, ArH), 4.74 (s, 1H, CH), 4.27 (d, J = 6.2 Hz, 4H, 2OCH2), 4.00 (s, 1H, CH), 1.32 (d, J = 8.6 Hz, 6H, 2CH3). 13C NMR (101 MHz, CDCl3) δ 168.09, 165.18, 16247, 150.17, 129.03, 128.17, 124.68, 120.07, 116.63, 115.26, 73.29, 62.72, 59.94, 46.81, 45.41, 37.27, 13.69.
Ethyl 2-cyano-3-(4-ammoniaphenyl)acrylate (, Entry 16)
Yellow solid. 1H NMR (400 MHz, DMSO-d6) δ 8.03 (s, 1H, CH=), 7.84 (d, J = 8.3 Hz, 2H, ArH), 6.73 (s, 2H, ArH), 6.66 (d, J = 8.3 Hz, 2H, ArH), 4.25 (q, J = 7.0 Hz, 2H, CH2), 1.27 (t, J = 7.0 Hz, 3H, CH3). 13C NMR (151 MHz, DMSO-d6) δ 164.01, 155.51, 154.83, 134.88, 118.82, 118.03, 113.97, 91.67, 61.82, 14.58.
Ethyl 2-cyano-3-(furan-2-yl) acrylate (, Entry 17)
Light yellow needle crystals. 1H NMR (400 MHz, CDCl3) δ 8.04 (s, 1H, =CH), 7.77 (s, 1H, -Fur-H), 7.40 (s, 1H, -Fur-H), 6.69 (s, 1H, -Fur-H), 4.38 (q, J = 7.1 Hz, 2H, CH2), 1.40 (t, J = 7.1 Hz, 3H, CH3).
Ethyl 2-cyano-3-(N-ethylcarbazol-3-yl) acrylate (, Entry 18)
Yellow crystals.1H NMR (400 MHz, CDCl3) δ 8.70 (s, 1H, =CH), 8.39 (s, 1H, ArH), 8.17 (dd, J = 21.2, 8.1 Hz, 2H, ArH), 7.43 (dt, J = 28.3, 21.0 Hz, 4H, ArH), 4.40 (td, J = 14.2, 7.0 Hz, 4H, 2CH2), 1.46 (dd, J = 16.4, 7.3 Hz, 6H, 2CH3).13C NMR (101 MHz, CDCl3) δ 163.66, 155.91, 142.76, 140.58, 129.04, 126.95, 125.38, 123.52, 122.78, 122.57, 120.95, 120.56, 117.02, 109.24, 109.29, 97.53, 62.30, 37.95, 14.34, 13.90. Anal. calcd for C20H18N2O2: C 75.45, N 8.80, H 5.70; found C 75.49, N 8.75, H 5.65.
2-(4-chlorobenzylidene) malononitrile (, Entry 19)
White solid. 1H NMR (400 MHz, CDCl3) δ 7.88 (d, J = 8.2 Hz, 2H, ArH), 7.77 (s, 1H, =CH), 7.54 (d, J = 8.3 Hz, 2H, ArH).
2-(4-nitrobenzylidene) malononitrile (, Entry 20)
Yellow solid. 1H NMR (400 MHz, CDCl3) δ 8.42 (d, J = 8.6 Hz, 2H, ArH), 8.10 (d, J = 8.6 Hz, 2H, ArH), 7.92 (s, 1H, =CH).
(4-methoxybenzylidene) malononitrile (, Entry 21)
Light yellow crystals. 1H NMR (400 MHz, CDCl3) δ 7.92 (d, J = 6.6 Hz, 2H, ArH), 7.67 (s, 1H, =CH), 7.03 (d, J = 6.6 Hz, 2H, ArH), 3.93 (s, 3H, CH3).
3. Results and discussion
3.1. Structure characterization
The morphologies of Fe3O4@SiO2@propyl@DBU and Fe3O4@SiO2 nonoparticales were analyzed by TEM. As shown in , two kinds of particles are spherical, and the diameters of them are between 10 and 20 nm. Combined with XRD analysis, it can be preliminarily confirmed that the SiO2, DBU and alkyl group are coated on the outside of the dark nano-Fe3O4 cores.
3.2. XRD analysis
The composition and structure of magnetic nanoparticles Fe3O4, Fe3O4@SiO2, Fe3O4@SiO2@propylchloride and Fe3O4@SiO2@propyl@DBU were confirmed by X-ray diffraction (XRD). As shown in the , the board peak at 18°–28° indicate that an amorphous silicon sell was formed around Fe3O4, the diffraction peaks 220, 311, 400, 422, 511 and 440 at 30.3°, 35.68°, 43.34°, 53.08°, 57.22° and 62.7° respectively represent the standard Fe3O4 diffraction card (JCPDS 89-0688) (Citation49). All diffraction peaks of magnetic nanoparticles conform to the peaks of standard Fe3O4, indicates that the surface modification of Fe3O4 does not cause phase transition. Additionally, the particle size of all magnetic nanoparticles can be calculated according to the Debye-Scherrer equation (D = kλ/βcosθ, where D is the crystal diameter, K is 0.89, λ is the wavelength of X-ray, θ is the Braggs angle in radians, and β is the full width at half maximum of the peak in radians). As show in , for the particle of Fe3O4, 2θ = 35.678, β = 0.598, D = 16.3 nm. the particle of Fe3O4@SiO2, 2θ = 35.64, β = 0.754, D = 12.9 nm. the particle of Fe3O4@SiO2@propylchloride, 2θ = 35.58, β = 0.696, D = 14.0 nm. And for the particle of Fe3O4@SiO2@propyl@DBU, 2θ = 35.76, β = 0.834, D = 11.7 nm. The diameter values of the magnetic nanoparticles are consistent with those obtained by TEM analysis.
3.3. FTIR analysis
In order to better make certain the functional groups and the structure of the magnetic nanoparticles, the FTIR spectroscopy was also performed. As shown in , the peak at 572 cm−1 is the characteristic peak of Fe-O bond stretching vibration, the broad peak at 1068 cm−1 is the characteristic peak of the Si-O bond stretching vibration. The characteristic peaks at 2897cm−1 and 2927cm−1 in curve (3) and curve (4) are associated with C–H bond stretching vibrations, suggesting that alkyl loads are on magnetic nanoparticles. The peak at 1639 cm−1 in curve (4) is the characteristic peak of C–N bond stretching vibration, which means the DBU molecule is attached to the magnetic nanoparticles.
3.4. TG analysis
To further investigate the structure, composition and thermal stability of the magnetic nanoparticles, the TG analysis was performed in the temperature range from 25°C to 1000°C. As can be seen from , Curve (1) is the mass loss diagram of Fe3O4, from which we can see that Fe3O4 has two obvious stages of mass loss. The first stage is from 25°C to 200°C with mass loss of 3.29%, possibly due to the evaporation of physically adsorbed water or residual solvent. The second stage is from 200°C to 700°C, with mass loss of 4.41%, which may be caused by hydroxyl group decomposition on the surface of Fe3O4. Adding the minimal loss of 0.06% above 700°C, the final mass loss is 7.76%. Curve (2) is the mass loss diagram of Fe3O4@SiO2. It can be seen from the figure that Fe3O4@SiO2 has two obvious mass loss stages. The first stage is from 25°C to 200°C with mass loss of 6.85%, which may be due to the evaporation of water or residual solvent physically adsorbed. The second stage is from 200°C to 600°C, the mass loss is 4.45%, which may be due to the silicon shell decomposition. There is a minimal loss of 0.03% above 600°C, and the final mass loss is 11.33%. Curve (3) is the mass loss diagram of Fe3O4@SiO2 modified by chloropropyl group. It can be seen from the that there are three stages of mass loss. The first stage is from 25 ° C to 120 ° C, with mass loss of 2.43%. which may be attributed to the residue water or other solvent on the surface of magnetic nanoparticle. The second stage was from 120°C to 540°C with mass loss of 6.55%, probably due to the decomposition of surface chloropropyl group and residual coupling agent. The third stage is from 540°C to 750°C with mass loss of 1.88%, which may be due to the decomposition of silicon shell. Adding the minimal loss of 0.59% above 750°C, the final mass loss is 11.45%. Curve (4) is the catalyst modified by DBU. It can be seen from the figure that there are three obvious mass loss stages. The first stage is from 25°C to 120°C, with mass loss of 1.7%, which may be due to the evaporation of water or residual solvent physically adsorbed. The second stage was from 120°C to 600°C, with mass loss of 9.02%, probably due to the cracking of DBU and chloropropyl group on the surface. The third stage is from 600°C to 1000°C, with mass loss of 2.36%, which may be due to the decomposition of silicon shell, and the final mass loss of 13.08%. Through TG analysis and comparison of the mass loss curves of the four kinds of magnetic nanoparticles, we may confirm that every step of preparation of magnetic nano catalyst is successful, and the DBU and alkyl group are indeed loaded on the surface of Fe3O4@SiO2 particles.
Figure 4. TG curves of nanoparticles. (1) Fe3O4, (2) Fe3O4@SiO2, (3) Fe3O4@SiO2@propylchloride, (4) Fe3O4@SiO2@propyl@DBU.
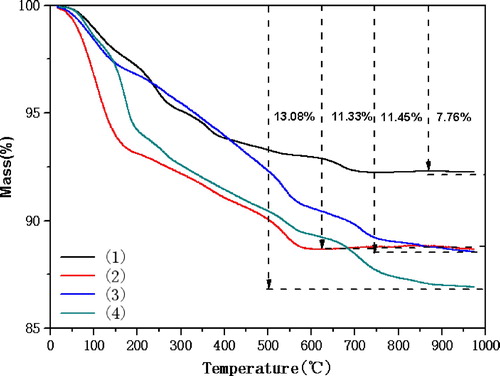
Combined with elemental analysis, it also performed to determine the amount of N in the catalyst and it was found to be 0.4215%. The loading of the DBU can be calculate and the value is about 2.29%.
3.5. VSM analysis
The magnetic measurement was carried out in the applied magnetic field at room temperature. The magnetic characteristics were measured by sweeping the magnetic field from −20000 to +20000 Oe using VSM and the results were shown in the . Not only the magnetic nano catalyst has good paramagnetism, but also it can be dispersed well in water. Under the external magnetic force, the dispersed nanoparticles can be aggregated very quickly. All of this provides a basis for the application and recovery of magnetic nano catalyst in water reaction.
3.6. SEM analysis
The morphology of catalyst was analyzed by scanning electron microscope (SEM). It can be seen from that the average size of the nanoparticles is between 10 and 15 nm, the shape of the catalyst particles is approximately spherical, and the agglomeration effect is produced due to their magnetic effect. The agglomeration effect makes the magnetic nano catalyst easily separated.
3.7. BET analysis
The N2 adsorption–desorption isotherm is as shown in , the apparent hysteresis loop in indicates that the catalyst belongs to mesoporous material. The surface area, pore volume and pore diameter of the catalyst were determined by BET test and the BET data is as shown in . According to the pore size and surface area of the catalyst, combined with the catalytic effect experiment, the nano catalyst can effectively catalyze the Knoevenagel reaction. The reason may be a comprehensive effect of ionic microenvironment, nanometer size, micropore effect and weak alkali environment.
Table 1. The results of BET surface area analysis.
3.8. Application of magnetic nanocatalyst in Knoevenagel reaction
In order to evaluate the catalytic activity of magnetic nano particle Fe3O4@SiO2@propyl@DBU (MNPs), the Knoevenagel reaction of p-chlorobenzaldehyde with ethyl cyanoacetate was selected as model reaction, and all kinds of reaction conditions were investigated, the experimental results are summarized in . As can be seen from , various factors influencing the reaction such as reaction temperature, base, solvents and amount of catalyst were investigated and optimized. Firstly, Fe3O4@SiO2@propyl@DBU (MNPs) was directly applied to the solvent-free Knovenagel reaction of p-chlorobenzaldehyde and ethyl cyanoacetate. Unfortunately, TLC showed no target product after 12 h (, entry 1), which may be caused by less contact between the MNPs and the reaction substrate in the solid–liquid two-phase state. Based on the good dispersion of MNPs in water, we added 2 mL H2O to the reaction system and found that the target product was obtained with a yield of 45% after 6 h (, entry 2). Since water can prompt the interaction of catalyst with reaction substrate, the amphiphilic PEG-400 might be better for the reaction. Indeed, when 2 mL PEG-400 was added to the aqueous reaction system, the target product was obtained with 50% yield after 3 h (, entry 3). Although the reaction time is greatly shortened, the yield is not ideal. Therefore, 1.0 g inorganic weak base K2CO3 was added into the reaction system. To our surprise, only after 30 min, the target product was obtained with 88% yield (, entry 5). At the same time, we also noticed that under the same reaction conditions, if there was no catalyst MNPs, only 70% yield of product was obtained after 40 min (, entry 11), and K2CO3 alone does not facilitate the reaction (, entry 6). In order to further evaluate the catalytic effect of MNPs, various reaction systems were used for the Knovenagel reaction between p-chlorobenzaldehyde and ethyl cyanoacetate (, entries 12–24). The results showed that the MNPs had an obvious catalytic effect, which not only accelerate the reaction rate, but also greatly improve the yield. In addition, other components such as K2CO3, H2O and PEG-400 can cooperate with the catalyst MNPs, which can effectively promote the Knovenagel reaction. In subsequent studies, organic base DABCO was used in the model reaction, but only the target product with moderate yield was obtained (, entries 12 and 13). In order to further optimize the catalytic effect of MNPs, the reaction temperature (, entries 14–17), the amount of catalyst (, entries 21–24) and the ratio of water to PEG-400 were further explored. It was found that the target product was obtained with 100% yield after 15 min when the reaction temperature is 40°C and the solvent catalyst system is MNPs (0.3 g) + H2O (6 mL) + PEG-400 (2 mL) + K2CO3 (1.0 g).
Table 2. Optimization of the reaction conditions.Table Footnotea
To further evaluate the scope and limitation of the catalyst MNPs on different substrates, all kinds of aromatic aldehydes were selected to react with ethyl cyanoacetate under the optimized conditions. As can be seen from , for various aromatic aldehydes attached to electron-withdrawing or electron-donating groups, the target product can be obtained almost at a quantitative yield within 1–40 min, and the reaction of aldehydes attached to electron-withdrawing groups is generally faster than that of aldehydes attached to electron-donating groups. Interestingly, the fused ring compound 9-anthraldehyde produced its target product at a 96% yield after 15 min, and for the terephthalaldehyde, both aldehyde groups undergo the Knovenagel reaction, 99% yield was achieved after 15 min. However, for the 4-dimethylaminobenzaldehyde and 4-aminobenzaldehyde attached to the strong electron-donating groups, there is a great difference in the reaction yield. The reason may be due to the instability of p-aminobenzaldehyde, as TLC analysis showed that there were multiple by-products produced during the reaction. It is particularly important to note that the reaction of 2-hydroxybenzaldehyde with ethyl cyanoacetate did not result in the normal Knovenagel product, Instead, a heterocyclic compound was obtained, which was consistent with the results reported in reference (Citation61). To our delight, heteroaryl aldehydes also underwent Knoevenagel reaction to give the corresponding adduct with a good yield (, entries 17, 18). To broaden the scope of the Knoevenagel reaction catalyzed by MNPs, we also carried out the MNPs induced Knoevenagel reaction of aldehydes with other active methylene compounds. When the malononitrile was selected as activated methylene compound, excellent yields were obtained (, entries19–20), unfortunately, when ethyl acetoacetate was used in the Knoevenagel reaction catalyzed by MNPs, almost no product was detected. In conclusion, magnetic nanocatalysts have excellent catalytic activity to the Knovenagel reaction of various aromatic aldehydes with ethyl cyanoacetate and malononitrile.
Table 3. Knoevenagel reaction of aldehydes with active methylene.Table Footnotea
Based on the excellent catalytic effect of magnetic nano-catalyst MNPs, its recyclability was further investigated, and the results are summarized in . Firstly, p-chlorobenzaldehyde (0.7 g, 5 mmol) were added to the solution of ethyl cyanoacetate (0.6 mL, 6 mmol), MNPs (0.3 g) and K2CO3 (1.0 g) in the composite solvent PEG400–H2O (6 mL H2O + 2 mL PEG400), then the reaction mixture was stirred at 40°C and the reaction progress was monitored by thin layer chromatography (TLC). After 15 min, TLC showed that p-chlorobenzaldehyde was consumed. When 50 mL water was added to the reaction mixture, a large number of flocculent solid products appeared. After the MNPs was separated from the reaction system by using the permanent magnet, the solid crude product was collected and recrystallized with 90% ethanol, and almost pure target product was obtained with quantitative yield. The separated MNPs were washed with ethyl acetate (10 mL × 2) and then used in the next cycle. It can be seen from that MNPs can be recycled, and there is almost no change in product yield and reaction time after 10 cycles.
Table 4. Reuse of the MNPs.Table Footnotea
4. Conclusion
In summary, organic base DBU was immobilized on the surface of silica-coated Fe3O4 nanoparticle to afford a functional magnetic nano catalyst Fe3O4@SiO2@propyl@DBU, which was used in the Knovenagel reaction of numerous aromatic aldehydes with ethyl cyanoacetate. The results showed that the nano catalyst MNPs have high catalytic activity, for most aldehydes, the reaction products were obtained at a nearly quantitative yield within 1–40 min. In addition to this, the catalyst MNPs can be easily recovered under external magnetic field, and reused for 10 runs with almost no loss of activity.
Supplemental Material
Download MS Word (1.2 MB)Acknowledgements
We are grateful for the financial support for this work from the Fund for ‘Shanxi 1331 Project’ Key Subjects Construction (2017-2021) and the Natural Science Foundation of Shanxi Province (No.201601D102015).
Disclosure statement
No potential conflict of interest was reported by the author(s).
Additional information
Funding
Notes on contributors
Xiaoyu Zhang
Xiaoyu Zhang is a graduate student at Shanxi Normal University, supervised by professor Sanhu Zhao, who is currently studying green chemistry.
Xiaoyan He
Xiaoyan He is a graduate student at Shanxi Normal University, supervised by professor Yongsheng Qiao and Sanhu Zhao, who is currently studying synthetic chemistry.
Sanhu Zhao
Sanhu Zhao received his MSc degree in organic chemistry at the Shanxi University of Taiyuan in 2005. His PhD work was in the field of green chemistry at the Shanxi University of Taiyuan, graduation in 2015. In 2012, he became a professor in chemistry in Xinzhou Teachers University. His interest is focused on green chemistry, organic synthesis, teaching and learning innovations.
References
- Freeman, F. Properties and Reactions of Ylidenemalononitriles. Chem. Rev. 1980, 80, 329–350.
- Zhao, S.; Wang, X.; Zhang, L. Rapid and Efficient Knoevenagel Condensation Catalyzed by a Novel Protic Ionic Liquid Under Ultrasonic Irradiation. RSC Adv. 2013, 3, 11691–11696.
- Wach, A.; Drozdek, M.; Dudek, B.; Biazik, M.; Lątka, P.; Kustrowski, P. Differences in Catalytic Activity of Poly(Vinylamine) Introduced on Surface of Mesoporous SBA-15 by Grafting from and Grafting onto Methods in Knoevenagel Condensation. J. Phys. Chem. C 2015, 119, 19954–19966.
- Elhamifar, D.; Kazempoor, S.; Karimi, B. Amine-functionalized Ionic Liquid-Based Mesoporous Organosilica as a Highly Efficient Nanocatalyst for The Knoevenagel Condensation. Catal. Sci. Technol. 2016, 6, 4318–4326.
- Mase, N.; Horibe, T. Organocatalytic Knoevenagel Condensations by Means of Carbamic Acid Ammonium Salts. T. Org. Lett.. 2013, 15, 1854–1857.
- Tran, U.P.N.; Le, K.A.; Phan, N.T.S. Expanding Applications of Metal-Organic Frameworks: Zeolite Imidazolate Framework ZIF-8 as an Efficient Heterogeneous Catalyst for the Knoevenagel Reaction. ACS Catal. 2011, 1, 120–127.
- Das, A.; Anbu, N.; M, S.K.; Dhakshinamoorthy, A.; Biswas, S. A Functionalized UiO-66 MOF for Turn-on Flfluorescence Sensing of Superoxide in Water and Efficient Catalysis for Knoevenagel Condensation. Dalton Trans. 2019, 48, 17371–17380.
- Gao, M.-L.; Qi, M.-H.; Liu, L.; Han, Z.-B. An Exceptionally Stable Core–Shell MOF/COF Bifunctional Catalyst for a Highly Efficient Cascade Deacetalization-Knoevenagel Condensation Reaction. Chem. Commun. 2019, 55, 6377–6380.
- He, Z.; Zhao, X.; Pan, X.; Li, Y.; Wang, X.; Xu, H.; Xu, Z. Ligand Geometry Controlling Zn-MOF Partial Structures for Their Catalytic Performance in Knoevenagel Condensation. RSC Adv. 2019, 9, 25170–25176.
- Hu, Y.; Zhang, J.; Huo, H.; Wang, Z.; Xu, X.; Yang, Y.; Lin, K.; Fan, R. One-pot Synthesis of Bimetallic Metal-Organic Frameworks (MOFs) as Acid-Base Bifunctional Catalysts for Tandem Reaction. Catal. Sci. Technol. 2020, 10, 315–322.
- Yao, C.; Zhou, S.; Kang, X.; Zhao, Y.; Yan, R.; Zhang, Y.; Wen, L. A Cationic Zinc-Organic Framework with Lewis Acidic and Basic Bifunctional Sites as an Efficient Solvent-Free Catalyst: CO2 Fixation and Knoevenagel Condensation Reaction. Inorg. Chem. 2018, 57, 11157–11165.
- Asgharnejad, L.; Abbasi, A.; Najafi, M.; Janczak, J. Synthesis and Structure of Three New Alkaline Earth Metal-Organic Frameworks with High Thermal Stability as Catalysts for Knoevenagel Condensation. Cryst. Growth Des. 2019, 19, 2679–2686.
- Zhang, Y.; Wang, Y.; Liu, L.; Wei, N.; Gao, M.-L.; Zhao, D.; Han, Z.-B. Robust Bifunctional Lanthanide Cluster Based Metal-Organic Frameworks (MOFs) for Tandem Deacetalization-Knoevenagel Reaction. Inorg. Chem. 2018, 57, 2193–2198.
- Shcherban, N.D.; Mäki-Arvela, P.; Aho, A.; Sergiienko, S.A.; Yaremov, P.S.; Eränenb, K.; Murzin, D.Y. Melamine-derived Graphitic Carbon Nitride as a New Effective Metal-Free Catalyst for Knoevenagel Condensation of Benzaldehyde with Ethylcyanoacetate. Catal. Sci. Technol. 2018, 8, 2928–2937.
- Tavakolian, M.; Najafpour, M.M. Molybdenum Carbide as an Efficient and Durable Catalyst for Aqueous Knoevenagel Condensation. New J. Chem. 2019, 43, 16437–16440.
- Sun, Y.; Cao, C.; Huang, P.; Yang, S.; Song, W. Amines Functionalized C60 as Solid Base Catalysts for Knoevenagel Condensation with High Activity and Stability. RSC Adv. 2015, 5, 86082–86087.
- Jain, K.; Chaudhuri, S.; Palc, K.; Das, K. The Knoevenagel Condensation Using Quinine as an Organocatalyst Under Solvent-Free Conditions. New J. Chem. 2019, 43, 1299–1304.
- Dou, M.-Y.; Zhong, D.-D.; Huang, X.-Q.; Yang, G.-Y. Imidazole-induced Self-Assembly of Polyoxovanadate Cluster Organic Framework for Efficient Knoevenagel Condensation Under Mild Conditions. Cryst. Eng. Comm. 2020, 22, 4147–4153.
- Kong, H.; He, P.; Yang, Z.; Xu, Q.; Wang, J.; Ban, R.; Ma, P.; Wang, J.; Niu, J. Selenotungstates Incorporating Organophosphonate Ligands and Metal Ions: Synthesis, Characterization, Magnetism and Catalytic Efficiency in Knoevenagel Condensation Reaction. Dalton Trans. 2020, 49, 7420–7425.
- Li, C.; Zhong, D.; Huang, X.; Shen, G.; Li, Q.; Du, J.; Wang, S.; Li, J.; Dou, J. Two Organic-Inorganic Hybrid Polyoxovanadates as Reusable Catalysts for Knoevenagel Condensation. New J. Chem. 2019, 43, 5813–5819.
- Qian, B.; Wang, F.; Li, D.; Li, Y.; Zhang, B.; Zhu, J. Preparation of Pickering Emulsion by Modification of Amine-Functionalized Graphene Oxide Surface with Organosilane: Efficient Catalyst for Knoevenagel Condensation of Malononitrile with Aldehydes at Mild Temperature. New J. Chem. 2020, 44, 5995–6002.
- Schejn, A.; Mazet, T.; Falk, V.; Balan, L.; Aranda, L.; Medjahdi, G.; Schneider, R. Fe3O4@ZIF-8: Magnetically Recoverable Catalysts by Loading Fe3O4 Nanoparticles Inside a Zinc Imidazolate Framework. Dalton Trans. 2015, 44, 10136–10140.
- Yu, Y.; Zhu, W.; Shi, B.; Lü, C. Construction of Thermo-Responsive Polymer Brushes Decorated Fe3O4@Catechol-Formaldehyde Resin Core-Shell Nanospheres Stabilized Carbon Dots/PdNPs Nanohybrid and its Application as an Efficient Catalyst. J. Mater. Chem. A 2020, 8, 4017–4029.
- Grass, R.N.; Athanassiou, E.K.; Stark, W.J. Covalently Functionalized Cobalt Nanoparticles as a Platform for Magnetic Separations in Organic Synthesis. Angew. Chem. Int. Ed. 2007, 46, 4909–4912.
- Liew, K.H.; Rocha, M.; Pereira, C.; Pires, A.L.; Pereira, A.M.; Yarmo, M.A.; Juan, J.C.; Yusop, R.M.; Peixoto, A.F.; Freire, C. Highly Active Ruthenium Supported on Magnetically Recyclable Chitosan-Based Nanocatalyst for Nitroarenes Reduction. Chem. Cat. Chem. 2017, 9, 3930–3941.
- Maleki, A. One-pot Multicomponent Synthesis of Diazepine Derivatives Using Terminal Alkynes in The Presence of Silica-Supported Superparamagnetic Iron Oxide Nanoparticles. Tetrahedron Lett. 2013, 54, 2055–2059.
- Maleki, A. Fe3O4/SiO2 Nanoparticles: An Efficient and Magnetically Recoverable Nanocatalyst for The One-Pot Multicomponent Synthesis of Diazepines. Tetrahedron 2012, 68, 7827–7833.
- Maleki, A.; Kari, T. Novel Leaking-Free, Green, Double Core/Shell, Palladium-Loaded Magnetic Heterogeneous Nanocatalyst for Selective Aerobic Oxidation. Catal. Lett. 2018, 148, 2929–2934.
- Maleki, A.; Panahzadeh, M.; Reza, E.-K. Agar: a Natural and Environmentally-Friendly Support Composed of Copper Oxide Nanoparticles for The Green Synthesis of 1,2,3-Triazoles. Green Chem. Lett. Rev. 2019, 12, 395–406.
- Maleki, A.; Akhlaghi, E.; Paydar, R. Design, Synthesis, Characterization and Catalytic Performance of a New Cellulose-Based Magnetic Nanocomposite in the One-Pot Three-Component Synthesis of α-Aminonitriles. . Appl. Organometal. Chem. 2016, 30, 382–386.
- Maleki, A.; Aghaei, M.; Ghamari, N. Facile Synthesis of Tetrahydrobenzoxanthenones via a One-Pot Three-Component Reaction Using an Eco-Friendly and Magnetized Biopolymer Chitosan-Based Heterogeneous Nanocatalyst. Appl. Organometal. Chem. 2016, 30, 939–942.
- Maleki, A.; Rahimi, R.; Maleki, S. Efficient Oxidation and Epoxidation Using a Chromium (VI)-Based Magnetic Nanocomposite. Environ. Chem. Lett. 2016, 14, 195–199.
- Maleki, A. One-pot Three-Component Synthesis of Pyrido [2′,1′:2,3] Imidazo[4,5-c] Isoquinolines Using Fe3O4@SiO2–OSO3H as an Efficient Heterogeneous Nanocatalyst. RSC Adv. 2014, 4, 64169–64173.
- Zhang, M.; Liu, Y.-H.; Shang, Z.-R.; Hu, H.-C.; Zhang, Z.-H. Supported Molybdenum on Graphene Oxide/Fe3O4 : An Efficient, Magnetically Separable Catalyst for One-Pot Construction of Spiro-Oxindole Dihydropyridines in Deep Eutectic Solvent Under Microwave Irradiation. Catal. Commun. 2017, 88, 39–44.
- Gao, G.; Di, J.-Q.; Zhang, H.-Y.; Mo, L.-P.; Zhang, Z.-H. A Magnetic Metal Organic Framework Material as A Highly Efficient and Recyclable Catalyst for Synthesis of Cyclohexenone Derivatives. J. Catal. 2020, 387, 39–46.
- Baig, R.B.; Varma, R.S.; Organic Synthesis via Magnetic Attraction: Benign and Sustainable Protocols Using Magnetic Nanoferrites. Green Chem. 2013, 15, 398–417.
- Zhu, Y.; Fang, Y.; Kaskel, S. Folate-Conjugated Fe3O4@SiO2 Hollow Mesoporous Spheres for Targeted Anticancer Drug Delivery. J. Phys. Chem. C 2010, 114, 16382–16388.
- Shao, M.; Ning, F.; Zhao, J.; Wei, M.; Evans, D.G.; Duan, X. Preparation of Fe3O4@SiO2@Layered Double Hydroxide Core-Shell Microspheres for Magnetic Separation of Proteins. J. Am. Chem. Soc. 2012, 134, 1071–1077.
- Liu, Y.; Chen, T.; Wu, C.; Qiu, L.; Hu, R.; Li, J.; Cansiz, S.; Zhang, L.; Tan, W. Facile Surface Functionalization of Hydrophobic Magnetic Nanoparticles. J. Am. Chem. Soc. 2014, 136, 12552–12555.
- Wang, B.G.; Ma, B.C.; Wang, Q.; Wang, W. Superparamagnetic Nanoparticle-Supported (S)-Diphenylprolinol Trimethylsilyl Ether as a Recyclable Catalyst for Asymmetric Michael Addition in Water. Adv. Synth. Catal. 2010, 352, 2923–2928.
- Yang, H.; Li, G.; Ma, Z. Magnetic Core-Shell-Structured Nanoporous Organosilica Microspheres for The Suzuki-Miyaura Coupling of Aryl Chlorides: Improved Catalytic Activity and Facile Catalyst Recovery. J. Mater. Chem. 2012, 22, 6639–6648.
- Chakraborty, T.; Sarkar, A. Pd (0) Immobilized on Fe3O4@AHBA: An Efficient Magnetically Separable Heterogeneous Nanocatalyst for C-C Coupling Reactions. J. Coord. Chem. 2019, 72, 3430–3443.
- Zhao, G.-L.; Shi, Y.-L.; Shi, M. Synthesis of Functionalized 2H-1-Benzopyrans by DBU-Catalyzed Reactions of Salicylic Aldehydes with Allenic Ketones and Esters. Org. Lett. 2005, 7, 4527–4530.
- Zhao, S.; Meng, D.; Wei, L.; Qiao, Y.; Xi, F. Novel DBU-Based Hydroxyl Ionic Liquid for Efficient Knoevenagel Reaction in Water. Green Chem. Lett. Rev. 2019, 12, 271–277.
- Meng, D.; Qiao, Y.; Wang, X.; Wen, W.; Zhao, S. DABCO-catalyzed Knoevenagel Condensation of Aldehydes with Ethyl Cyanoacetate Using Hydroxy Ionic Liquid as a Promoter. RSC Adv. 2018, 8, 30180–30185.
- Gholizadeh, A. A Comparative Study of Physical Properties in Fe3O4 Nanoparticles Prepared by Coprecipitation and Citrate Methods. J. Am. Ceram. Soc. 2017, 100, 1–2.
- Bu, D.; Li, N.; Zhou, Y.; Feng, H.; Yu, F.; Cheng, C.; Li, M.; Xiao, L.; Ao, Y. Enhenced UV Stability of N-Halamine-Immobilized Fe3O4@SiO2@TiO2 Nanoparticles: Synthesis, Characteristics and Antibacterial Property. New J. Chem. 2020, 44, 10352–10358.
- Chae, H.S.; Kim, S.D.; Piao, S.H.; Choi, H.J. Core-Shell Structured Fe3O4@SiO2 Nanoparticles Fabricated by Sol-gel Method and Their Magnetorheology. Colloid Polym. Sci. 2016, 294, 647–655.
- Lu, D.; Fan, H.; Kondamareddy, K.K.; Yu, H.; Wang, A.; Hao, H.; Li, M.; Shen, J. Highly Efficient Visible-Light-Induced Photocatalytic Production of Hydrogen for Magnetically Retrievable Fe3O4@SiO2@MoS2/g-C3N4 Hierarchical Microspheres. ACS Sustain. Chem. Eng. 2018, 6, 9903–9911.
- Yue, C.; Mao, A.; Wei, Y.; Lue, M. Knoevenagel Condensation Reaction Catalyzed by Task-Specific Ionic Liquid Under Solvent-Free Conditions. Catal. Commun. 2008, 9, 1571–1574.
- Ren, Y.-M.; Cai, C. Knoevenagel Condensation of Aromatic Aldehydes with Active Methylene Compounds Using a Catalytic Amount of Iodine and K2CO3 at Room Temperature. Synth. Commun. 2007, 37, 2209–2213.
- Song, A.; Wang, X.; Lam, K.S. A Convenient Synthesis of Coumarin-3-Carboxylic Acids Via Knoevenagel Condensation of Meldrum’s Acid with Ortho-Hydroxyaryl Aldehydes or Ketones. Tetrahedron Lett. 2003, 44, 1755–1758.
- Ying, A.-G.; Wang, L.-M.; Chen, X.-Z.; Ye, W.-D. Green and Efficient Knoevenagel Condensation Catalysed by a DBU Based Ionic Liquid in Water. J. Chem. Res. 2010, 34, 30–33.
- Mowry, D. Nacin-Niacinamide Dintiation in the Microbiologcal Assay Procedrue, β, β-Dimesitylvinyl Alcohol, New Compounds: Ethyl α,4-Dicyanocinnamate. J. Am. Chem. Soc. 1943, 65, 992–993.
- Pal, R.; Yasmin, H.; Nahar, L.; Datta, B.; Chowdhury, A.; Kundu, J.; Bachar, S.; Sarker, S. Synthesis of 5,6-Dichloroindan-1-Acids and Their Tetrazolyl Derivatives as Analgesic and Anti-Inflammatory Agents. Med. Chem. 2012, 8, 874–882.
- Li, G.; Xiao, J.; Zhang, W. Knoevenagel Condensation Catalyzed by a Tertiary-Amine Functionalized Polyacrylonitrile Fifiber. Green Chem. 2011, 13, 1828–1836.
- Zhang, J.; Jiang, T.; Han, B.; Zhu, A.; Ma, X. Knoevenagel Condensation Catalyzed by 1,1,3,3-Tetramethylguanidium Lactate. Synth. Commun. 2006, 36, 3305–3317.
- Yasuda, H.; Midorikawa, H. The Knoevenagel Reaction Between Hydroxybenzaldehydes and Ethyl Cyanoacetate. Bull. Chem. Soc. Jpn. 1966, 39, 1754–1759.
- Lemek, T.; Mayr, H. Electrophilicity Parameters for Benzylidenemalononitriles. J. Org. Chem. 2003, 68, 6880–6886.
- Das, A.; Anbu, N.; M, S.K.; Dhakshinamoorthy, A.; Biswas, S. A Functionalized UiO-66 MOF for Turn-on Fluorescence Sensing of Superoxide in Water and Efficient Catalysis for Knoevenagel Condensation. Dalton Trans. 2019, 48, 17371–17380.
- Costantino, U.; Cnrini, M.; Montanari, F.; Nocchetti, M. Hydrotalcite-like Compounds as Heterogeneous Catalysts in Liquid Phase Organic Synthesis. II. Preparation of 4H-Chromenes Promoted by Hydrotalcite Doped with Hydrous Tin (IV) Oxide. Microporous Mesoporous Mater. 2008, 107, 16–22.
- Denmark, S.; Ibrahim, M.; Ambrosi, A. Room Temperature, Reductive Alkylation of Activated Methylene Compounds: Carbon-Carbon Bond Formation Driven by the Rhodium-Catalyzed Water-Gas Shift Reaction. ACS Catal. 2017, 7, 613–630.
- Oskooie, H.A.; Roomizadeh, E.; Heravi, M.M. Solvent-Free L-Proline Catalyzed Condensation of Ethyl Cyanoacetate with Aldehydes. J. Chem. Res. 2006, 4, 246–247.
- Ren, Z.; Cao, W.; Tong, W. The Knoevenagel Condensation Reaction of Aromatic Aldehydes with Malononitrile by Grinding in the Absence of Solvents and Catalysts. Synth. Commun. 2002, 32, 3475–3497.