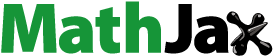
ABSTRACT
In this work, the bismuth-terephthalate frameworks (Bi-BDC) were prepared by a facile solvothermal approach. The prepared Bi-BDC MOFs were characterized by XRD, TGA, FT-IR, SEM, and UV-vis DRS analyses. The results indicated that the synthesis temperature affected the crystallinity and morphologies of the Bi-BDC frameworks. Notably, the produced Bi-BDC at low temperatures (80 and 100 oC) exhibited enhanced light absorption ability. The photodegradation experiment of rhodamine (RhB) showed that the prepared Bi-BDC-100 sample had the highest removal efficiency of ca. ∼ 98% after 270 min of visible LED light illumination. In addition, the HPLC-MS analysis revealed that RhB was photocatalytically degraded over the Bi-BDC via the carboxylation and dealkylation mechanism. Furthermore, the removal efficiency of the organic pollutant was optimized by monitoring the effects of catalyst dosage, dye concentration, and pH media. Finally, cyclic tests implied that the synthesized Bi-BDC MOF had good stability and reusability.
GRAPHICAL ABSTRACT

1. Introduction
Water polluted by textile dyes is considered the most severe environmental issue globally (Citation1–5). Rhodamine B (RhB) is widely used among known dyes, and a large amount is released into the environment. RhB is toxic and can cause skin irritation, allergic dermatitis, and cancer. Furthermore, a significant amount of RhB is released into the environment as metabolites, threatening the ecological environment and human health. Thus, removing RhB residues from the environment has received considerable attention (Citation6–9). Many strategies, such as absorption, biological, advanced-oxidation, and heterogeneous photocatalytic methods, have been developed to remove organic contaminants (Citation10–14). Among these methodologies, photocatalytic degradation is a promising route because it is simple, low-cost, and highly effective (Citation1,Citation15,Citation16). Furthermore, the contaminants can be decomposed into minor toxic inorganic matter, CO2, and water during the photocatalytic process (Citation17–19). Conventional photocatalysts, such as TiO2 (Citation20–22); ZnO (Citation23,Citation24); SiO2 (Citation25), MnFe2O4 (Citation26) or Fe3O4 (Citation27) have been extensively studied for photodegradation of organic dyes in water. Nonetheless, these materials showed particular limitations owing to their low porosities.
Recently, metal–organic frameworks (MOFs), a new porous class material, have emerged as potential photocatalysts owing to their attractive properties such as exceptional high porosity, high crystallinity, and tunable pore structures (Citation28–31). Many MOF structures have been applied for photocatalytic processes, including UiO-66(Zr) (Citation32); MIL-100(Fe) (Citation33), MIL100(Fe)/TNF (Citation34), MIL-53(Fe) (Citation35), MIL-125(Ti) (Citation36), MIL-125(Ti)/carbon nanotube (Citation37) and Ag/AgCl@CoFe2O4/NH2-MIL-125(Ti) (Citation38). Recently, bismuth-based MOFs with a high valence state of Bi and flexible coordination geometry are promising materials (Citation39). Vilela et al. (Citation40) synthesized the Bi-AzoBTC framework, showing using environmentally friendly water as the only solvent. Shamaila Iram et al. (Citation41) prepared several Bi-MOFs with different linkers, exhibiting good luminescence and gas adsorption properties. Vinh Huu Nguyen et al. (Citation42) synthesized a bismuth-terephthalate framework, which showed a good photocatalytic performance toward Rhodamine B under visible light irradiation. However, research has found that the synthesis of bismuth-based frameworks remains challenging because their structures are sensitive to experimental conditions, including solvent, temperature, and time (Citation43). Furthermore, it is hard to control crystal the nucleation and growth of Bi-MOF particles during the synthesis, challenging in optimizing their performances (Citation44–46).
Herein, the bismuth-terephthalate framework (Bi-BDC) was simply prepared by solvothermal method at varying reaction temperatures. It was realized that the synthesis temperature strongly affected the revolution of both morphology and crystallinity of the products. Furthermore, the reaction temperature also impacted the Bi-BDC band gap value, tuning their photocatalytic activities toward RhB textile dye under LED visible-light illumination. For the first time, the effects of synthesis conditions on Bi-MOF characteristics and their photo-reduction activities were investigated.
2. Experimental
2.1. Materials
1,4–benzene dicarboxylic acid (H2BDC, ≥ 98%) and bismuth (III) nitrate pentahydrate (Bi(NO3)3·5H2O, ≥ 99.5%) were obtained from Sigma-Aldrich Co., Ltd. N, N–dimethylformamide (DMF, C3H7NO, 99%), and rhodamine B dye (RhB, C28H31ClN2O3, 95%) were purchased from Xilong Scientific Co., Ltd. All chemicals were used as received without further purification.
2.2. Synthesis of Bi-BDC framework
The Bi-BDC framework was prepared by utilizing the solvothermal method. Typically, Bi(NO3)3.5H2O (6 mmol) and H2BDC (9 mmol) were dissolved in 60 ml DMF with constant stirring. The solution was transferred into a Teflon-lined autoclave (V = 100 mL) and sealed in a stainless-steel vessel. The reactor was heated at an assigned temperature (80 – 120 oC) for 48 h. After the reaction, the reactor was naturally cooled down to room temperature. The product was collected by centrifuged and washed with DMF (60 oC) and ethanol (60 oC) to eliminate the remaining reactants. The obtained solids were degassed at 120 oC under vacuum conditions for 12 h before use.
2.3. Characterizations
The crystallographic structures were analyzed using powder X-ray diffraction (LabX XRD-6100, Shimadzu, Japan). The morphology of the samples was analyzed by field-emission scanning electron microscopy (JEOL JSM 7401F, Tokyo, JAPAN). The functional groups were analyzed employing an FT-IR spectrometer (Tensor 27, Bruker, Germany). The synthesized catalysts’ thermal stability was measured in a nitrogen atmosphere at a heating rate of 10°C/min using a thermogravimetric analyzer (TA Instruments, Linseis, North, USA). Finally, the optical properties were analyzed using UV–Vis DRS (Shimazu UV-2450 UV−Vis spectrophotometer, Kyoto, Japan). Metal leading test was performed by ICP-OES analysis.
2.4. Photocatalytic degradation experiment
The photolytic degradation of RhB dye was processed under irradiation of visible LED light (Six Cree® Xlamp® XM-L2 LEDs, Cree, Inc., Durham, NC, USA) with a power of 10 W and a light output of up to 1052 lm. For each run, 30 mg of material was added to the solution and magnetically stirred for 90 min in the dark. After exposure to visible LED light illumination with continuous stirring, 5 mL of the reaction mixture was withdrawn and centrifuged to recover the clear solution. The content of RhB was measured employing a UV-visible spectrophotometer UV-Vis spectroscopy (Cary 60 UV–Vis, Agilent technologies, US) at the absorption wavelength λmax = 554 nm. The absorbance Ao was measured after stirring for 90 min in the dark and represented for the initial concentration Co of the solution. The absorbance At after variable periods of illumination was corresponded to the residual concentration Ct. The photodegradation efficiencies of Bi-BDC materials were calculated as following equation: . All experiments were performed in triplicate. The data are expressed as average values of three measurements.
For the effect of initial concentration and irradiation time to photodegradation efficiency, the 50 mL of RhB with concentrations in a range of 5–20 mg/mL were added to 30 mg Bi-BDC-100 under irradiation time of 0–360 min. The effect of photocatalyst dosage on the photodegradation of RhB was determined by using various amounts of photocatalyst ranging from 10 to 50 mg with 50 mL of 15 mg/mL RhB solution. The effect of pH on the photodegradation efficiency was examined by mixing 30 mg of Bi-BDC-100 and RhB 50 mL of 15 mg/mL, with the pH ranging from 3 to 11. The NaOH and HCl 0.1 M solutions were used to adjust the pH values.
For investigation of samples recyclability of catalyst, after irradiation time of 360 min, 3 mL of reaction solution was taken at the end of the photocatalytic process and the catalyst was separated through centrifugation. After each working cycle, a definite amount volume of RhB was added to the reaction system in order to keep the reaction solution at 50 mL and 15 mg/mL for using in the future experiment.
3. Results and discussion
3.1. Characterizations
depicts the SEM images of the prepared Bi-BDC-80, Bi-BDC-100, and Bi-BDC-120 samples. At a low temperature of 80 oC, the produced Bi-BDC were highly agglomerated particles (a). Further increasing to 100 and 120 oC, the samples were rod-shaped crystals of 2–3 µm in length (b and c). shows the XRD patterns of the synthesized materials. The XRD patterns of all samples exhibited the characteristic diffraction peaks at 2θ = 7.5, 9.0, 16.5, and 26.0 o, which were assigned to the Bi-BDC framework (Citation42,Citation47,Citation48). The FT-IR analyses of the samples were performed, as described in . The characteristic peaks at 1580 and 1400 cm–1 were attributed to the asymmetric and symmetric stretching vibration of the COO- groups in the terephthalate linker. Moreover, the vibrational modes at a lower wavenumber of ∼770, 650, and 500 cm−1 were assigned to Bi–O bonds (Citation49). The results implied that the Bi-BDC frameworks were successfully prepared under the investigated conditions.
Figure 2. XRD patterns of the Bi-BDC frameworks were prepared at temperatures of 80, 100, and 120 oC.
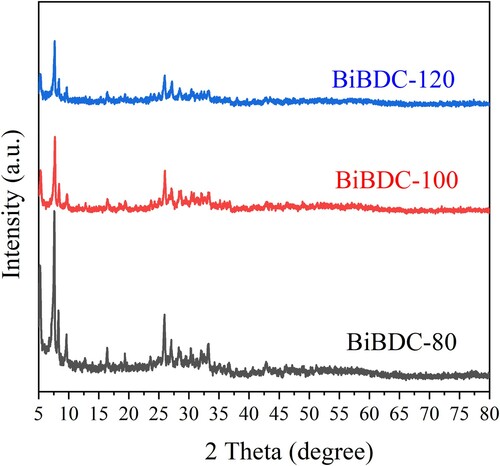
shows the TGA analyses of the synthesized Bi-BDC samples. As revealed, the first weight loss at low temperature (<100 oC) was caused by the evaporation of solvents (Citation41,Citation50,Citation51). The second weight loss occurs between 100 and 250°C was ascribable to the release of the solvent molecules adsorbed within the pores of Bi-BDC frameworks. Finally, the significant weight loss at around 380 oC was due to the ligand decompositions. These suggest that the resultant Bi-BDC MOFs are stable up to 380 oC, which is consistent with the previous reports (Citation52).
The optical properties of the prepared Bi-BDC-80, Bi-BDC-100, and Bi-BDC-120 samples were explored via their UV-Vis diffuse reflectance spectra, as described in . It can be observed that all samples had absorption edges in the range of 350- 400 nm, suggesting the materials were strongly stimulated under UV light. Notably, the absorption edge of the produced Bi-BDC-80 and Bi-BDC-100 slightly shifted toward a higher wavelength region, indicating their higher absorption light ability than their counterpart. We hypothesize that low temperature could generate more defect sites within the Bi-BDC framework, which enhanced the light response of the materials. The bandgap energy (Eg) values of the samples were obtained from the Tauc plots (b). Accordingly, the Eg value of Bi-BDC-80, Bi-BDC-100, and Bi-BDC-120 was 3.55, 3.58, and 3.62 eV, respectively.
3.2. Photocatalytic degradation of RhB over Bi-BDC frameworks
The photodegradation experiments were carried out under LED visible light illumination. shows the UV-vis absorption spectra of RhB as a function of sampling time. It can be seen clearly that the RhB absorption intensity decreased with increasing the sampling time. Moreover, the maximum absorption wavelength of RhB slightly shifted from 554 to 500 nm. This could be due to the decomposition of Rhodamine B to RhB N-demethylated during the photocatalytic process (Citation53). The removal efficiency of RhB over the prepared Bi-BDC MOFs was calculated, and the results are shown in . In the pre-adsorption stage, the adsorption of RhB onto the catalyst surfaces reached equilibrium for 90 min. After being exposed to light, the removal efficiency of RhB over the synthesized Bi-BDC catalysts rapidly increased and reached equilibrium after 360 min irradiation (a). It can be seen that the Bi-BDC MOF prepared at 100 oC exhibited the best photodegradation performance compared to the others. This could be because the Bi-BDC framework formed at a moderate temperature of 100 oC had optimal crystallinity and bandgap energy. After 360 min of visible light illumination, the total removal yield of RhB over Bi-BDC-80 and Bi-BDC-100 were ∼ 98 and 96%, respectively, which are comparable with many previously reported materials (see ). The kinetic study revealed that the degradation rate of RhB over the synthesized catalysts followed the order: Bi-BDC-100 (0.017 min−1) > Bi-BDC-80 (0.012 min–1) > Bi-BDC-120 (0.008 min−1) (b). The findings indicated that the RhB contaminant was more rapidly degraded over the Bi-BDC-100 catalyst. The reaction mixture was further analyzed by HPLC-MS analysis to reveal the formation of intermediate products during the photodegradation of RhB over the produced Bi-BDC-100 sample. As described in , the m/z values of 443, 399, and 355 were observed on the HPLC-MS spectrum. Under visible light irradiation, RhB initially formed a product with the m/z value of 399 via carboxylation, followed by dealkylation to form a product with the m/z value of 355. These intermediate products could further decompose to produce low molecular weight byproducts, CO2 and H2O.
Figure 6. Absorption spectra of RhB solution under various irradiation time (catalyst dosage: 30 mg/L and 50 mL RhB concentration of 15 mg/mL).
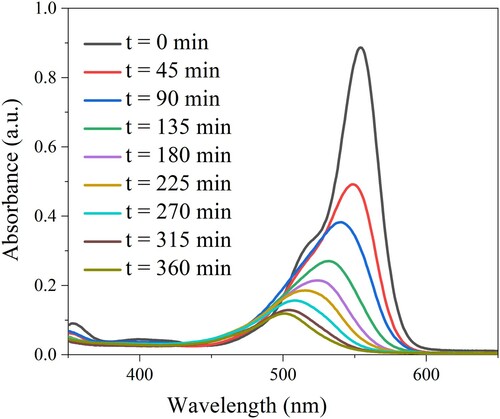
Figure 7. (a) Photodegradation profile of RhB under various irradiation time, and (b) photodegradation kinetics of pseudo-first-order (catalyst dosage: 30 mg/L and 50 mL RhB concentration of 15 mg/mL).
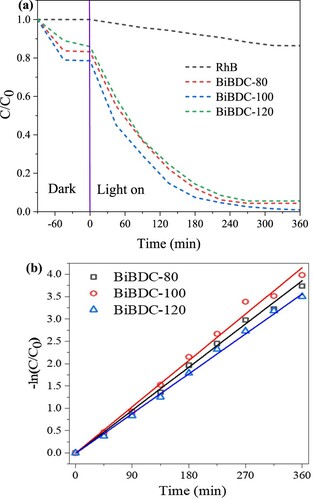
Figure 8. The proposed photodegradation mechanism of RhB dye over the prepared Bi-BDC under visible LED light irradiation.
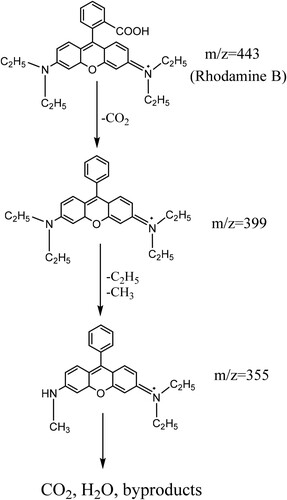
Table 1. The photodegradation of RhB dye over various materials.
3.3. Effects of experimental conditions
show the effects of internal parameters on the RhB removal efficiency over the synthesized Bi-BDC-100 catalyst. As revealed in a, the removal efficiency increased with the catalyst dosage, suggesting more active sites for adsorption and photocatalytic process. b shows that the initial contaminant concentration affected the adsorption and photodegradation of the Bi-BDC-100 catalyst. At low concentrations, RhB was decolorized by both adsorption and photodegradation. Contrarily, the adsorption and photooxidation were slowed down when loading a high content of dye owing to the saturation of active sites of the catalyst (Citation7,Citation54). c demonstrates that media pH strongly impacted the photocatalytic activity of the sample. Accordingly, the photodegradation of RhB onto the catalyst was favored in the acidic media but reduced in the alkaline media. As RhB is known as a cation dye, the accumulation of RhB onto the catalyst would be accelerated at high media pH (pH >7) because of the electrostatic interaction. However, the adsorption of a high content of organic contaminants on the catalyst's surface could shield and suppress the catalyst's absorption light, causing a decrease in photocatalytic performance.
3.4. Reusability tests
Reusability is one of the crucial aspects of the catalyst. After each removal experiment, the spent catalyst was collected and washed several times with deionized water, then dried at 120 oC for eight hours before reusing as a regenerated catalyst. a shows the removal efficiency of RhB over Bi-BDC-100 after three cyclic experiments. As presented, the removal yield of RhB remained ∼93% after three cycles, meaning a slight loss compared to the as-prepared catalyst. b shows the XRD pattern of the Bi-BDC-100 catalyst before and after the reaction. As revealed, the crystallinity of Bi-BDC-100 remained unchanged during the photocatalysis process. Moreover, the metal ion leading tests at pH = 3, pH = 7, and pH = 11 revealed no trace of Bi in the solution. These findings suggest that the synthesized Bi-BDC MOF was highly stable.
4. Conclusions
In conclusion, the Bi-BDC frameworks were simply synthesized by solvothermal synthesis at various temperatures. The results indicated that the synthesis temperature strongly impacted the morphology, crystallite size, and bandgap energy of the produced materials. With increasing the temperature from 80 to 120 oC, the bandgap of the sample increased from 3.55–3.62 eV, respectively. The Bi-BDC prepared at 100 oC exhibited the most excellent photo-reduction rate of ∼ 0.017 min−1 toward RhB dye under visible LED light conditions. After 360 min irradiation, the photodegradation efficiency over the Bi-BDC catalyst reached ∼ 98%. Moreover, the synthesized Bi-BDC maintained high photodegradation efficiency and good stability after four cycles. The findings implied that the photocatalytic activities of the Bi-BDC frameworks could be tuned by controlling the synthesis temperature.
Author contributions
Material preparation, data collection and analysis were performed by Pham Hoang Ai Le, Trinh Duy Nguyen, and Huynh Huu Khanh. Writing-original draft, writing-review and editing were conducted by The Ky Vo, Thai Son Pham Quynh and Van-Cuong Nguyen. Data curation, software, and validation were performed by The Ky Vo and Van-Cuong Nguyen. All authors have read and agreed to the published version of the manuscript.
Disclosure statement
No potential conflict of interest was reported by the author(s).
Additional information
Funding
References
- Liu, H.; Wang, C.; Wang, G. Photocatalytic Advanced Oxidation Processes for Water Treatment: Recent Advances and Perspective. Chem - An Asian J 2020, 15, 3239–3253. doi:10.1002/asia.202000895. Cited: in:: PMID: 32860468.
- Bedia, J.; Muelas-Ramos, V.; Peñas-Garzón, M.; Gómez-Avilés, A.; Rodríguez, J.J.; Belver, C. A Review on the Synthesis and Characterization of Metal Organic Frameworks for Photocatalytic Water Purification. Catalysts 2019, 9. doi:10.3390/catal9010052.
- Wen, Y.; Zhang, P.; Sharma, V.K.; Ma, X.; Zhou, H.C. Metal-organic Frameworks for Environmental Applications. Cell Reports Phys Sci 2021, 2, 100348. doi:10.1016/j.xcrp.2021.100348.
- Mahmoodi, N.M.; Hayati, B.; Arami, M. Textile Dye Removal from Single and Ternary Systems Using Date Stones: Kinetic, Isotherm, and Thermodynamic Studies. J. Chem. Eng. Data 2010, 55, 4638–4649. doi:10.1021/je1002384.
- Hayati, B.; Mahmoodi, N.M. Modification of Activated Carbon by the Alkaline Treatment to Remove the Dyes from Wastewater: Mechanism, Isotherm and Kinetic. Desalin Water Treat 2012, 47, 322–333. doi:10.1080/19443994.2012.696429.
- Thakur, A.; Kaur, H. Response Surface Optimization of Rhodamine B dye Removal Using Paper Industry Waste as Adsorbent. Int J Ind Chem 2017, 8, 175–186. doi:10.1007/s40090-017-0113-4.
- Duy Trinh, N.; Hoang, H.H.; Xuan Linh, N.; Huu Vinh, N.; Thi Vu, H.; Thanh Nguyen, H.; Do, S.T.; Viet N-Vo, D. Visible Light Induced Enhanced Photocatalytic Degradation of Industrial Effluents (Rhodamine B) Using BiVO4 Nanoparticles. IOP Conf Ser Mater Sci Eng 2019, 542. doi:10.1088/1757-899X/542/1/012060.
- Cui, Y.; Goldup, S.M.; Dunn, S. Photodegradation of Rhodamine B Over Ag Modified Ferroelectric BaTiO3 Under Simulated Solar Light: Pathways and Mechanism. RSC Adv. 2015, 5, 30372–30379. doi:10.1039/c5ra00798d.
- Mahmoodi, N.M.; Taghizadeh, M.; Taghizadeh, A. Mesoporous Activated Carbons of low-Cost Agricultural bio-Wastes with High Adsorption Capacity: Preparation and Artificial Neural Network Modeling of dye Removal from Single and Multicomponent (Binary and Ternary) Systems. J. Mol. Liq. 2018, 269, 217–228. doi:10.1016/j.molliq.2018.07.108.
- Yang, X.; Chen, Z.; Zhao, W.; Liu, C.; Qian, X.; Zhang, M.; Wei, G.; Khan, E.; Hau Ng, Y.; Sik Ok, Y. Recent Advances in Photodegradation of Antibiotic Residues in Water. Chem. Eng. J. 2021, 405, 126806. doi:10.1016/j.cej.2020.126806.
- Zajda, M.; Aleksander-Kwaterczak, U. Wastewater Treatment Methods for Effluents from the Confectionery Industry-An Overview. J Ecol Eng 2019, 20, 293–304. doi:10.12911/22998993/112557.
- Tang, J.; Liu, H.; Zhao, C.; Rao, T.; Hu, L.; Hu, C.; Zhang, L.; Li, T. A Novel ZrGeO4 Catalyst for Degradation of Organic dye Pollutants at Room Temperature Without Light Illumination. Green Chem Lett Rev 2020, 13, 215–222. doi:10.1080/17518253.2020.1804625.
- Nguyen, T.H.A.; Tran, T.D.M.; Ky Vo, T.; Nguyen, Q.T.; Nguyen, V.-C. Facile Synthesis of low-Cost Chitosan/Fe3O4@C Composite for Highly Efficient Adsorption of Levofloxacin Antibiotic. Chem. Eng. Commun. 2022, 1–13. doi:10.1080/00986445.2022.2053680.
- Mahmoodi, N.M.; Saffar-Dastgerdi, M.H. Clean Laccase Immobilized Nanobiocatalysts (Graphene Oxide - Zeolite Nanocomposites): From Production to Detailed Biocatalytic Degradation of Organic Pollutant. Appl Catal B Environ 2020, 268, 118443. doi:10.1016/j.apcatb.2019.118443.
- Hoque, M.A.; Guzman, M.I. Photocatalytic Activity: Experimental Features to Report in Heterogeneous Photocatalysis. Materials (Basel) 2018, 11. doi:10.3390/ma11101990.
- Ahmed, S.N.; Haider, W. Heterogeneous Photocatalysis and its Potential Applications in Water and Wastewater Treatment: A Review. Nanotechnology 2018, 29. doi:10.1088/1361-6528/aac6ea.
- Boyjoo, Y.; Sun, H.; Liu, J.; Pareek, V.K.; Wang, S. A Review on Photocatalysis for Air Treatment : From Catalyst Development to Reactor Design A Review on Photocatalysis for air Treatment : From Catalyst Development to Reactor Design. Chem. Eng. J. 2016, 310, 537–559. doi:10.1016/j.cej.2016.06.090.
- Yang, X.; Wang, D. Photocatalysis: From Fundamental Principles to Materials and Applications. ACS Appl Energy Mater 2018, 1, 6657–6693. doi:10.1021/acsaem.8b01345.
- Takanabe, K. Photocatalytic Water Splitting: Quantitative Approaches Toward Photocatalyst by Design. ACS Catal. 2017, 7, 8006–8022. doi:10.1021/acscatal.7b02662.
- Chen, D.; Cheng, Y.; Zhou, N.; Chen, P.; Wang, Y.; Li, K.; Huo, S.; Cheng, P.; Peng, P., Zhang, R., et al. Photocatalytic Degradation of Organic Pollutants Using TiO2-Based Photocatalysts: A Review. J. Clean. Prod. 2020, 268, 121725. doi:10.1016/j.jclepro.2020.121725.
- Riaz, S.; Park, S.-J. An Overview of TiO2-Based Photocatalytic Membrane Reactors for Water and Wastewater Treatments. J. Ind. Eng. Chem. 2020, 84, 23–41. doi:10.1016/j.jiec.2019.12.021.
- Mahmoodi, N.M. Photocatalytic Degradation of Dyes Using Carbon Nanotube and Titania Nanoparticle. Water, Air, Soil Pollut. 2013, 224, 1612. doi:10.1007/s11270-013-1612-3.
- Sheikh, M.; Pazirofteh, M.; Dehghani, M.; Asghari, M.; Rezakazemi, M.; Valderrama, C.; Cortina, J.-L. Application of ZnO Nanostructures in Ceramic and Polymeric Membranes for Water and Wastewater Technologies: A Review. Chem. Eng. J. 2020, 391, 123475. doi:10.1016/j.cej.2019.123475.
- Ong, C.B.; Ng, L.Y.; Mohammad, A.W. A Review of ZnO Nanoparticles as Solar Photocatalysts: Synthesis, Mechanisms and Applications. Renew. Sustain. Energy Rev. 2018, 81, 536–551. doi:10.1016/j.rser.2017.08.020.
- Dong, C.; Ji, J.; Yang, Z.; Xiao, Y.; Xing, M.; Zhang, J. Research Progress of Photocatalysis Based on Highly Dispersed Titanium in Mesoporous SiO2. Chinese Chem Lett 2019, 30, 853–862. doi:10.1016/j.cclet.2019.03.020.
- Mahmoodi, N.M. Manganese Ferrite Nanoparticle: Synthesis, Characterization, and Photocatalytic dye Degradation Ability. Desalin Water Treat 2015, 53, 84–90. doi:10.1080/19443994.2013.834519.
- Teixeira, S.; Mora, H.; Blasse, L.-M.; Martins, P.M.; Carabineiro, S.A.C.; Lanceros-Méndez, S.; Kühn, K.; Cuniberti, G. Photocatalytic Degradation of Recalcitrant Micropollutants by Reusable Fe3O4/SiO2/TiO2 Particles. J. Photochem. Photobiol. A. Chem. 2017, 345, 27–35. doi:10.1016/j.jphotochem.2017.05.024.
- Wang, Q.; Gao, Q.; Al-Enizi, A.M.; Nafady, A. Ma S. Recent Advances in MOF-Based Photocatalysis: Environmental Remediation Under Visible Light. Inorg. Chem. Front. Royal Society of Chemistry 2020, 7, 300–339.
- Nasalevich, M.A.; Van Der Veen, M.; Kapteijn, F.; Gascon, J. Metal-organic Frameworks as Heterogeneous Photocatalysts: Advantages and Challenges. CrystEngComm 2014, 16, 4919–4926. doi:10.1039/c4ce00032c.
- Li, Y.; Xu, H.; Ouyang, S.; Ye, J. Metal-organic Frameworks for Photocatalysis. Phys. Chem. Chem. Phys. Royal Society of Chemistry 2016, 18, 7563–7572.
- Piątek, J.; Budnyak, T.M.; Monti, S.; Barcaro, G.; Gueret, R.; Grape, E.S.; Jaworski, A.; Inge, A.K.; Rodrigues, B.V.M.; Slabon, A. Toward Sustainable Li-Ion Battery Recycling: Green Metal–Organic Framework as a Molecular Sieve for the Selective Separation of Cobalt and Nickel. ACS. Sustain. Chem. Eng. 2021, 9, 9770–9778. doi:10.1021/acssuschemeng.1c02146.
- Vo, T.K.; Kim, J. Facile Synthesis of Magnetic Framework Composite MgFe2O4@UiO-66(Zr) and its Applications in the Adsorption–Photocatalytic Degradation of Tetracycline. Environ Sci Pollut Res 2021, 28, 68261–68275. doi:10.1007/s11356-021-15423-y. Cited: in:: PMID: 34268686.
- Mahmoodi, N.M.; Abdi, J.; Oveisi, M.; Alinia Asli, M.; Vossoughi, M. Metal-organic Framework (MIL-100 (Fe)): Synthesis, Detailed Photocatalytic dye Degradation Ability in Colored Textile Wastewater and Recycling. Mater. Res. Bull. 2018, 100, 357–366. doi:10.1016/j.materresbull.2017.12.033.
- Oveisi, M.; Mahmoodi, N.M.; Asli, M.A. Facile and Green Synthesis of Metal-Organic Framework/Inorganic Nanofiber Using Electrospinning for Recyclable Visible-Light Photocatalysis. J Clean Prod. 2019, 222, 669–684. doi:10.1016/j.jclepro.2019.03.066.
- Liu, N.; Jing, C.; Li, Z.; Huang, W.; Gao, B.; You, F.; Zhang, X. Effect of Synthesis Conditions on the Photocatalytic Degradation of Rhodamine B of MIL-53 (Fe). Mater. Lett. 2019, 237, 92–95.
- Fatima, R.; Park, S.; Kim, J.-O. Effect of Molar Ration of Ti/Ligand on the Synthesis of MIL-125 (Ti) and its Adsorption and Photocatalytic Properties. J. Ind. Eng. Chem. 2020, 90, 166–177.
- Oveisi, M.; Alinia Asli, M.; Mahmoodi, N.M. Carbon Nanotube Based Metal-Organic Framework Nanocomposites: Synthesis and Their Photocatalytic Activity for Decolorization of Colored Wastewater. Inorganica Chim Acta [Internet] 2019, 487, 169–176. doi:10.1016/j.ica.2018.12.021.
- Mahmoodi, N.M.; Taghizadeh, A.; Taghizadeh, M.; Abdi, J. In Situ Deposition of Ag/AgCl on the Surface of Magnetic Metal-Organic Framework Nanocomposite and its Application for the Visible-Light Photocatalytic Degradation of Rhodamine dye. J. Hazard. Mater. 2019, 378, 120741. doi:10.1016/J.JHAZMAT.2019.06.018. Cited: in:: PMID: 31200227.
- Xu, B.; An, Y.; Liu, Y.; Huang, B.; Qin, X.; Zhang, X.; Dai, Y.; Whangbo, M.-H. An Efficient Visible-Light Photocatalyst Made from a Nonpolar Layered Semiconductor by Grafting Electron-Withdrawing Organic Molecules to its Surface. Chem. Commun. 2016, 52, 13507–13510.
- Vilela, S.M.F.; Devic, T.; Várez, A.; Salles, F.; Horcajada, P. A new Proton-Conducting Bi-Carboxylate Framework. Dalt Trans 2019, 48, 11181–11185.
- Iram, S.; Imran, M.; Kanwal, F.; Iqbal, Z.; Deeba, F.; Iqbal, Q.J. Bismuth(III) Based Metal Organic Frameworks: Luminescence. Gas Adsorption, and Antibacterial Studies. Zeitschrift fur Anorg und Allg Chemie 2019, 645, 50–56. doi:10.1002/zaac.201800383.
- Nguyen, V.H.; Nguyen, T.D.; Van Nguyen, T. Microwave-Assisted Solvothermal Synthesis and Photocatalytic Activity of Bismuth(III) Based Metal–Organic Framework. Top. Catal. 2020, 63, 1109–1120. doi:10.1007/s11244-020-01271-6.
- Seetharaj, R.; Vandana, P.V.; Arya, P.; Mathew, S. Dependence of Solvents, pH, Molar Ratio and Temperature in Tuning Metal Organic Framework Architecture. Arab. J. Chem 2019, 12, 295–315.
- Köppen, M.; Dhakshinamoorthy, A.; Inge, A.K.; Cheung, O.; Ångström, J.; Mayer, P.; Stock, N. Synthesis, Transformation, Catalysis, and Gas Sorption Investigations on the Bismuth Metal–Organic Framework CAU-17. Eur. J. Inorg. Chem. 2018, 2018, 3496–3503. doi:10.1002/ejic.201800321.
- Ye, F.; Wei, Z.X.; Song, J.F.; Wu, X.H.; Yue, P. Synthesis, Characterization, and Photocatalytic Properties of Bismuth (III)-Benzene-1,3,5-Tricarboxylate. Zeitschrift fur Anorg und Allg Chemie 2017, 643, 669–674. doi:10.1002/zaac.201700096.
- Wang, G.; Sun, Q.; Liu, Y.; Huang, B.; Dai, Y.; Zhang, X.; Qin, X. A Bismuth-Based Metal-Organic Framework as an Efficient Visible-Light-Driven Photocatalyst. Chem - A Eur J 2015, 21, 2364–2367. doi:10.1002/chem.201405047. Cited: in:: PMID: 25487284.
- Zhao, X.; Zhong, J.; Hu, J.; Wu, L.; Chen, X. Bismuth Terephthalate Induced Bi(0) for Enhanced RhB Photodegradation and 4-Nitrophenol Reduction. J. Phys. Chem. Solids 2017, 111, 431–438. doi:10.1016/j.jpcs.2017.08.034.
- Zhao, X.; Xiong, X.; Chen, X.; Hu, J.; Li, J. Synthesis of Halide Anion-Doped Bismuth Terephthalate Hybrids for Organic Pollutant Removal. Appl. Organomet. Chem. 2016, 30, 304–310. doi:10.1002/aoc.3432.
- Yang, J.; Xie, T.; Liu, C.; Xu, L. Facile Fabrication of Dumbbell-Like β-Bi2O3/Graphene Nanocomposites and Their Highly Efficient Photocatalytic Activity. Materials (Basel) 2018, 11. doi:10.3390/ma11081359.
- Imran, M.; Kanwal, F.; Latif, S.; Iqbal, Z.; Mitu, L. Heteronuclear Metal-Organic Frameworks; Adsorption and Luminescence Aspects. Rev. Chim. 2020, 71, 38–46. doi:10.37358/RC.20.4.8041.
- Vo, T.K. Spray Pyrolysis Synthesis and UV-Driven Photocatalytic Activity of Mesoporous Al2O3@TiO2 Microspheres. Environ. Sci. Pollut. Res. Int. 2022, 29, 42991–43003.
- Iram, S.; Imran, M.; Kanwal, F.; Latif, S.; Iqbal, Z. Bismuth and Lead Based Metal Organic Frameworks: Morphological, Luminescence and Brunauer-Emmett-Teller (BET) Studies. Mater Sci Pol 2020, 38, 132–137. doi:10.2478/msp-2020-0021.
- Wang, P.; Cheng, M.; Zhang, Z. On Different Photodecomposition Behaviors of Rhodamine B on Laponite and Montmorillonite Clay Under Visible Light Irradiation. J. Saudi Chem. Soc. 2014, 18, 308–316. doi:10.1016/j.jscs.2013.11.006.
- Vo, T.K.; Kim, J. Facile Synthesis of Magnetic Framework Composite MgFe2O4@UiO-66 (Zr) and its Applications in the Adsorption-Photocatalytic Degradation of Tetracycline. Environ. Sci. Pollut. Res. Int. 2021, 28, 68261–68275.
- Sha, Z.; Sun, J.; On Chan, H.S.; Jaenicke, S.; Wu, J. Bismuth Tungstate Incorporated Zirconium Metal-Organic Framework Composite with Enhanced Visible-Light Photocatalytic Performance. RSC Adv. 2014, 4, 64977–64984. doi:10.1039/c4ra13000f.
- Nguyen, V.H.; Van Tan, L.; Lee, T.; Nguyen, T.D. Solvothermal Synthesis and Photocatalytic Activity of Metal-Organic Framework Materials Based on Bismuth and Trimesic Acid. Sustain Chem Pharm 2021, 20, 100385. doi:10.1016/j.scp.2021.100385.
- Chen, G.; Qian, S.; Tu, X.; Wei, X.; Zou, J.; Leng, L.; Luo, S. Enhancement Photocatalytic Degradation of Rhodamine B on NanoPt Intercalated Zn-Ti Layered Double Hydroxides. Appl. Surf. Sci. 2014, 293, 345–351. doi:10.1016/j.apsusc.2013.12.165.
- Thi Mai Tho, N.; The Huy, B.; Nha Khanh, D.N.; Quoc Thang, N.; Thi Phuong Dieu, N.; Dai Duong, B.; Thi Kim Phuong, N. Mechanism of Visible-Light Photocatalytic Mineralization of Indigo Carmine Using ZnBi2O4-Bi2S3 Composites. ChemistrySelect 2018, 3, 9986–9994. doi:10.1002/slct.201802151.
- Nha Khanh, D.N.; Lin, H.N.; Mai Tho, N.T.; Nhat Ha, H.N.; Huy, V.Q.; Phuong Dieu, N.T.; Huy, D.M.; Dat, D.P.; Kim Phuong, N.T. Influence of Ammonia on Properties of TiO2-MgFe2 O4 as High Visible-Light Active Photocatalysts for the Degradation of Rhodamine B. Vietnam J Chem 2018, 56, 798–803. doi:10.1002/vjch.201800090.
- Jonjana, S.; Phuruangrat, A.; Thongtem, T.; Kuntalue, B.; Thongtem, S. Decolorization of Rhodamine B Photocatalyzed by Ag3PO4/Bi2WO6 Nanocomposites Under Visible Radiation. Mater. Lett. 2018, 218, 146–149. doi:10.1016/J.MATLET.2018.01.176.
- Wang, C.Y.; Wu, T.; Lin, Y.W. Preparation and Characterization of Bismuth Oxychloride/Reduced Graphene Oxide for Photocatalytic Degradation of Rhodamine B Under White-Light Light-Emitting-Diode and Sunlight Irradiation. J. Photochem. Photobiol. A. Chem. 2019, 371, 355–364. doi:10.1016/J.JPHOTOCHEM.2018.11.043.
- Campos, W.E.O.; Lopes, A.S.C.; Monteiro, W.R.; Filho, G.N.R.; Nobre, F.X.; Luz, P.T.S.; Nascimento, L.A.S.; Costa, C.E.F.; Monteiro, W.F., Vieira, M.O., et al. Layered Double Hydroxides as Heterostructure LDH@Bi2WO6 Oriented Toward Visible-Light-Driven Applications: Synthesis, Characterization, and its Photocatalytic Properties. React Kinet Mech Catal 2020, 131, 505–524. doi:10.1007/s11144-020-01830-8.
- Onwumere, J.; Pia̧tek, J.; Budnyak, T.; Chen, J.; Budnyk, S.; Karim, Z.; Thersleff, T.; Kuśtrowski, P.; Mathew, A.P.; Slabon, A. CelluPhot: Hybrid Cellulose−Bismuth Oxybromide Membrane for Pollutant Removal. ACS Appl. Mater. Interfaces 2020, 12, 42891–42901. doi:10.1021/acsami.0c12739.