ABSTRACT
In this context, we have demonstrated a highly efficient and green process for the preparation of various potentially biologically active functionalized 4-(5-hydroxybenzo[a]phenazin-6-yl)-5-phenyl-1,3-dihydro-2H-imidazol-2-one derivatives via a one-pot, the four-component cyclo-condensation reaction in the presence of catalytic amounts of phosphotungstic acid supported on TiO2 as a nontoxic, highly reactive, and eco-friendly solid acid catalyst under MWI in solvent-free conditions. The crystallinity and the crystallite size nano-TiO2 were fabricated and characterized by X-ray diffraction (XRD), Transmission electron microscopy (TEM), scanning electron microscopy (FESEM), Thermogravimetric Analysis (TGA), Fourier transform infrared (FT-IR), Brunauer-Emmett-Teller (BET), and atomic force microscopy (AFM). We believe that this work can provide new insights into the fabrication of high-performance photocatalysts and facilitate their practical application in environmental issues.
GRAPHICAL ABSTRACT
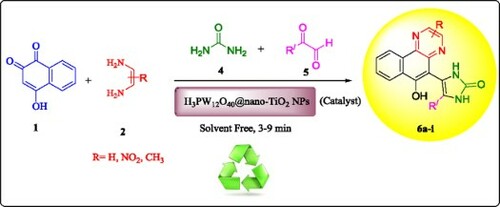
1. Introduction
Multi-component reactions (MCRs) have considerable ecological interest for use in the synthesis of biologically important compounds. The design of novel MCRs for the synthesis of diverse heterocycles has become an important topic for medicinal and organic chemists (Citation1). Reducing laboratory time and costs is an important benefit of multicomponent reactions (MCRs) (Citation2,Citation3). In most multi-component reactions, the largest number of atoms participating in the reaction are present in the product. One of the most important aspects of multi-component reactions is their high synthetic efficiency (Citation4–7).
In addition to their wide application in pharmaceutical sciences and drug synthesis, multi-component reactions are used in other fields, including materials science and the synthesis of environmentally friendly compounds, or the preparation of chiral stationary phases in chromatography (Citation8–11). Multi-component reactions can also be used for the artificial synthesis of amino acids and peptide macromolecules or in the polymer industry and the production of a variety of polymers such as polysaccharides (Citation12–14).
Since 1986, materials have been synthesized using the microwave (Citation15). Microwave heating is one of the most important applications of these waves. This is an application that has been used well in recent decades to perform various chemical reactions and has led to the emergence of a branch of chemistry called microwave chemistry (Citation16–18). The microwave method has become increasingly used in the synthesis of various reactions due to its properties such as uniform heat distribution, selective heating, increasing reaction speed and reducing time, the energy required to walk, and improving the physical and chemical properties of synthesized particles (Citation19–21).
In biology, the active site is the region of an enzyme where substrate molecules bind and undergo a chemical reaction. The active site consists of amino acid residues that form temporary bonds with the substrate (binding site) and residues that catalyze a reaction of that substrate (catalytic site). Although the active site occupies only ∼10–20% of the volume of an enzyme, is the most important part as it directly catalyzes the chemical reaction (Citation22).
Due to its chemical and physical stability under reaction conditions and non-toxicity, titanium dioxide, in addition to its various applications in pigments and ceramics, has been widely used as a photocatalyst in the removal of environmental pollutants (Citation23,Citation24). TiO2 has many functional properties in the crystalline phase of anatase. The form of anatase has a high photocatalytic activity and therefore has been used to eliminate organic and inorganic pollutants in the environment (Citation25–27).
The application and efficiency of TiO2 are strongly influenced by its crystal structure, shape, and particle size. Therefore, many efforts have been made to produce TiO2 nanoparticles with controlled size, shape, and porosity for use in thin films, ceramics, composites, and catalysts (Citation28–30).
TiO2 is used in a variety of fields such as paints, plastics, cosmetics, inks, papers, and sensors. The increasing use of TiO2 nanoparticles in the field of catalysts, photocatalysts, and sensors has intensified the need to use precision equipment for their synthesis (Citation31–33).
Phenazines are a large group of nitrogen-containing heterocyclic compounds that differ in chemical and physical properties. Phenazines are a class of redox-active secondary metabolites produced by a few clades of bacteria, most notably pseudomonads,20,22 but not cyanobacteria. Bacteria are the only known source of phenazines, and more than 100 types of these compounds have been identified. Their potential impact on bacterial interactions and biotechnological processes has been considered () (Citation34,Citation35). Natural phenazines are considered biologically active and are used in pigments and have many biological properties such as antioxidants (Citation36), anti-AIDS (HIV) (Citation37,Citation38), anti-tumor (Citation39,Citation40), and the treatment of Alzheimer's and schizophrenia (Citation40,Citation41).
Herein, we report here the results of our study on the reaction between 2-hydroxy-1,4-naphthoquinone 1, benzene-1,2-diamine 2, urea 4, and Arylglyoxals 5 in the presence of H3PW12O40@nano-TiO2 as a nanocatalyst recyclable using microwave irradiation under solvent-free is reported ().
2. Experimental section
2.1. General Procedures
All reagents and solvents were purchased from Merck and Aldrich and used without further purification. Melting points were taken in open capillaries and are uncorrected. IR spectra were recorded on a Perkin-Elmer-1420 spectrophotometer. 1H NMR spectra (DMSO) were recorded on a Gemini-500 MHz spectrophotometer with TMS as the internal standard. Elemental analyses for C, H, and N were performed using a Costech ECS 4010 CHNS-O analyzer. Mass spectra were recorded on an Agilent Technology (HP) spectrometer operating at an ionization potential of 70 eV.
2.1.1. Preparation of nanocomposite
TTIP (2 mL) and different amounts of H3PW12O40 were added into isopropanol, respectively, and then mixed under vigorous stirring. After that, diluted hydrochloric acid was added to adjust the pH value. The mixture was stirred at room temperature until the gelatin was formed. After aging for 12 h, the resulting gelatin was transferred into a Teflon-lined stainless-steel autoclave and maintained at 200 0C for 2 h with a heating rate of 2 0C /min. The obtained white product was denoted as x-H3PW12O40/TiO2, in which x represented the H3PW12O40 loading amount (wt%) in the composite photocatalyst (Citation42,Citation43).
2.1.2. General procedure for the preparation of 4-(5-hydroxybenzo[a]phenazin-6-yl)-5-phenyl-1, 3-dihydro-2H-imidazol-2-one derivatives under microwave irradiation
Initially, 2-hydroxynaphthalene-1,4-dione 1 (1 mmol) and benzene-1,2-diamine 2 (1 mmol) were mixed at (300 W, max. 100 0C) (under thermal or microwave irradiation and solvent-free conditions) until in less than 3-5 min an orange solid of benzo[a]phenazine 3 was formed (Citation44,Citation45).
A 25 mL reaction flask was charged with a mixture of Arylglyoxals 4 (1 mmol), urea 5 (1 mmol), and 0.03 gr of H3PW12O40@nano-TiO2 nanoparticles Then, this mixture was stirred under thermal conditions at 100 °C or it was transferred to a microwave oven at 300 W (max. 100 °C) for a specific time. Upon completion of the reaction, monitored by TLC, the reaction mixture was allowed to cool to room temperature. Then, 10 mL of water was added to the mixture and filtered for separation of the crude product (). The separated product was washed twice with water (2×5 mL) (Citation46).
Table 1. synthesis of 4-(5-hydroxybenzo[a]phenazin-6-yl)-5-phenyl-1, 3-dihydro-2H-imidazol-2-one derivatives.
The structures of these new compounds were deduced from their satisfactory elemental and spectral (IR, 1H, 13C NMR, and MS) studies. The mass spectra of these compounds displayed molecular ion peaks at the appropriate m/z values.
2.1.3. 4-(5-hydroxybenzo[a]phenazin-6-yl)-5-phenyl-1, 3-dihydro-2H-imidazol-2-one (6a)
Brown powder; yield 0.386 g (71%), m.p 269–271 °C; IR (KBr) (νmax cm−1): 3130, 3030, 2165, 1673, 1631, 1582, 1513, 1430, 1405, 1338, 1313, 1283, 1218, 1140, 1068, 1046, 954, 906, 824, 763 cm–1; 1H NMR (500 MHz, DMSO-d6) δ 7.37–7.65 (m, 10H, Ar-H), 7.94 (ddd, J = 6.9, 2.4, 1.4 Hz, 2H, Ar-H), 7.98–8.04 (m, 1H, Ar-H), 8.95 (s, 1H, Ar-H), 9.00 (s, 1H, OH), 9.96 (s, 1H, NH) ppm; 13C NMR (125 MHz, DMSO-d6) δ 110.7, 118.9, 124.3, 124.5, 124.8, 126.7, 127.3, 127.6, 128.3, 128.6, 128.7, 128.8, 128.9, 129.2, 129.3, 129.4, 129.5, 134.3, 136.5, 137.7, 140.3, 142.6; 152.8, 153.6; MS (m/z, %): 404 (M+, 65); Anal. Calcd for C25H16N4O2: C, 74.25; H, 3.99; N, 13.85%. Found: C, 74.15; H, 3.90; N, 13.81%.
2.1.4. 4-(4-bromophenyl)-5-(5-hydroxy-10-nitrobenzo[a]phenazin-6-yl)-1, 3-dihydro-2H-imidazole-2-one (6b)
Brown powder; yield 0.385 g (68%), m.p 263–265 °C; IR (KBr) (νmax cm−1): 3175, 3030, 1674, 1585, 1521, 1439, 1408, 1338, 1275, 1212, 1141, 1081, 1058, 1023, 973, 945, 833, 759 cm–1; 1H NMR (500 MHz, DMSO-d6) δ 7.32 (dd, J = 7.5, 1.6 Hz, 1H, Ar-H), 7.44 (td, J = 7.5, 1.6 Hz, 1H, Ar-H), 7.57–7.48 (m, 5H, Ar-H), 8.00 (dd, J = 7.5, 1.5 Hz, 1H, Ar-H), 8.27 (dd, J = 7.5, 1.5 Hz, 1H, Ar-H), 8.40 (d, J = 7.5 Hz, 1H, Ar-H), 9.00–8.94 (m, 2H, Ar-H), 9.03 (s, 1H, OH), 9.96 (s, 1H, NH) ppm; 13C NMR (125 MHz, DMSO-d6) δ 110.5, 119.2, 123.9, 124.3, 124.8, 125.0, 125.9, 126.5, 127.6, 127.7, 127.9, 128.6, 129.2, 129.3, 131.2, 134.13, 137.6, 137.8, 141.2, 142.1, 148.2, 152.8, 153.8; MS (m/z, %): 527 (M+, 85); Anal. Calcd for C25H14BrN5O4: C, 56.84; H, 2.67; N, 13.26%. Found: C, 56.95; H, 2.76; N, 13.35%.
2.1.5. 4-(5-hydroxy-10-nitrobenzo[a]phenazin-6-yl)-5-(p-tolyl)-1,3-dihydro-2H-imidazol-2-one (6c)
Brown powder; yield 0.387 g (69%), m.p 278–280 °C; IR (KBr) (νmax cm−1): 3195, 3040, 1658, 1575, 1503, 1435, 1403, 1307, 1237, 1217, 1177, 1140, 1068, 1007, 956, 892, 824, 734 cm–1; 1H NMR (500 MHz, DMSO-d6) δ 2.37 (s, J = 0.9 Hz, 3H, CH3), 7.22–7.31 (m, 4H, Ar-H), 7.34 (dd, J = 7.5, 1.5 Hz, 1H, Ar-H), 7.49 (td, J = 7.5, 1.6 Hz, 1H, Ar-H), 7.55-7.58 (td, J = 7.4, 1.6 Hz, 1H, Ar-H), 8.00 (dd, J = 7.4, 1.6 Hz, 1H, Ar-H), 8.19 (d, J = 7.4 Hz, 1H, Ar-H), 8.27 (dd, J = 7.5, 1.6 Hz, 1H, Ar-H), 8.94 (s, 1H, Ar-H), 8.98 (d, J = 1.4 Hz, 1H, Ar-H), 9.02 (s, 1H, OH), 9.96 (s, 1H, NH) ppm; 13C NMR (125 MHz, DMSO-d6) δ 21.3, 110.5, 119.1, 123.4, 124.26, 124.99, 125.88, 126.25, 126.47, 127.42, 127.62, 128.5, 128.8, 129.2, 129.3, 134.1, 137.7, 137.8, 139.3, 141.2, 142.0, 148.2, 152.8, 153.6; MS (m/z, %): 463 (M+, 73); Anal. Calcd for C26H17N5O4: C, 67.38; H, 3.70; N, 15.11%. Found: C, 67.25; H, 3.75; N, 15.02%.
2.1.6. 4-(5-hydroxy-10-nitrobenzo[a]phenazin-6-yl)-5-(4-methoxyphenyl)-1,3-dihydro-2H-imidazol -2-one (6d)
Brown powder; yield 0.372 g (86%), m.p 274–276 °C; IR (KBr) (νmax cm−1): 3030, 2825, 1664, 1636, 1586, 1510, 1433, 1403, 1368, 1310, 1260, 1227, 1162, 1068, 1046, 1001, 961, 855, 825, 758, 715 cm–1; 1H NMR (500 MHz, DMSO-d6) δ 3.83 (s, 3H, OCH3), 6.93–6.99 (m, 2H, Ar-H), 7.27–7.35 (m, 3H, Ar-H), 7.44 (td, J = 7.5, 1.5 Hz, 1H, Ar-H), 7.54 (td, J = 7.4, 1.5 Hz, 1H, Ar-H), 7.98 (dd, J = 7.4, 1.6 Hz, 1H, Ar-H), 8.28 (dd, J = 7.5, 1.5 Hz, 1H, Ar-H), 8.40 (d, J = 7.5 Hz, 1H, Ar-H), 8.93 (m, 2H, Ar-H), 8.94–9.00 (s, 1H, OH), 9.96 (s, 1H, NH) ppm; 13C NMR (125 MHz, DMSO-d6) δ 55.3, 110.5, 112.8, 119.02, 120.2, 124.2, 125.0, 125.9, 126.4, 127.5, 127.6, 127.7, 128.3, 129.2, 129.3, 134.1, 137.5, 137.8, 141.2, 142.0, 148.2, 152.8, 153.6, 161.0; MS (m/z, %): 479 (M+, 56); Anal. Calcd for C26H17N5O5: C, 65.13; H, 3.57; N, 14.61%. Found: C, 65.25; H, 3.45; N, 14.71%.
2.1.7. 4-(5-hydroxybenzo[a]phenazin-6-yl)-5-(p-tolyl)-1, 3-dihydro-2H-imidazol-2-one (6e)
Brown powder; yield 0.395 g (75%), m.p 223–225 °C; IR (KBr) (νmax cm−1): 3310, 3115, 2905, 1673, 1629, 1527, 1508, 1429, 1405, 1337, 1311, 1284, 1222, 1178, 1140, 1067, 1008, 955, 900, 830, 758 cm–1; 1H NMR (500 MHz, DMSO-d6) δ 2.40 (s, J = 1.0 Hz, 3H, CH3), 7.19–7.24 (m, 2H, Ar-H), 7.29–7.35 (m, 2H, Ar-H), 7.45–7.56 (m, 3H, Ar-H), 7.58–7.65 (m, 2H, Ar-H), 7.94 (ddd, J = 6.6, 2.7, 1.9 Hz, 2H, Ar-H), 7.98–8.06 (m, 1H, Ar-H), 8.93 (s, 1H, Ar-H), 9.02 (s, 1H, OH), 9.95 (s, 1H, NH) ppm; 13C NMR (125 MHz, DMSO-d6) δ 21.3, 110.9, 119.1, 123.3, 124.3, 124.8, 126.3, 127.4, 127.6, 128.7, 128.8, 128.9, 129.1, 129.2, 129.3, 129.5, 134.3, 136.5, 137.7, 139.3, 140.3, 142.6, 152.8, 153.6; MS (m/z, %): 418 (M+, 45); Anal. Calcd for C26H18N4O2: C, 74.63; H, 4.34; N, 13.39%. Found: C, 74.58; H, 4.45; N, 13.28%.
2.1.8. 4-(5-hydroxybenzo[a]phenazin-6-yl)−5-(4-methoxyphenyl)−1,3-dihydro-2H-imidazol-2-one (6f)
Brown powder; yield 0.375 g (89%), m.p 228–230 °C; IR (KBr) (νmax cm−1): 3255, 3030, 2170, 1664, 1633, 1586, 1510, 1433, 1403, 1409, 1368, 1310, 1260, 1162, 1068, 1001, 961, 825, 758 cm–1; 1H NMR (500 MHz, DMSO-d6) δ 3.83 (s, 3H, OCH3), 6.92–6.98 (m, 2H, Ar-H), 7.27–7.33 (m, 2H, Ar-H), 7.43–7.48 (m, 1H, Ar-H), 7.49 (td, J = 7.4, 1.6 Hz, 1H, Ar-H), 7.55 (td, J = 7.3, 1.8 Hz, 1H, Ar-H), 7.58–7.66 (m, 2H, Ar-H), 7.90–7.98 (m, 2H, Ar-H), 8.10-8.93 (m, J = 7.3, 1.6 Hz, 3H, Ar-H), 9.05 (s, 1H, OH), 9.95 (s, 1H, NH) ppm; 13C NMR (125 MHz, DMSO-d6) δ 55.3, 110.9, 112.5, 119.1, 120.4, 124.3, 125.0, 127.5, 127.6, 127.7, 128.5, 128.7, 128.9, 129.3, 129.4, 129.5, 134.3, 136.5, 137.0, 140.3, 142.6, 152.8, 153.6, 161.0; MS (m/z, %): 434 (M+, 91); Anal. Calcd for C26H18N4O3: C, 71.88; H, 4.18; N, 12.90%. Found: C, 71.78; H, 4.25; N, 12.98%.
2.1.9. 4-(5-hydroxy-10-methylbenzo[a]phenazin-6-yl)−5-(p-tolyl)−1,3-dihydro-2H-imidazol-2-one (6g)
Brown powder; yield 0.318 g (58%), m.p 277–279 °C; IR (KBr) (νmax cm−1): 3030, 2880, 1679, 1627, 1582, 1513, 1479, 1437, 1403, 1372, 1310, 1279, 1221, 1180, 1149, 1069, 1003, 958, 816, 761 cm–1; 1H NMR (500 MHz, DMSO-d6) δ 2.39 (s, J = 0.7 Hz, 3H, CH3), 2.43 (s, J = 0.9 Hz, 3H, CH3), 7.19–7.24 (m, 2H, Ar-H), 7.29–7.34 (m, 2H, Ar-H), 7.37 (dq, J = 7.8, 1.3 Hz, 1H, Ar-H), 7.43–7.52 (m, 2H, Ar-H), 7.55 (td, J = 7.3, 1.8 Hz, 1H, Ar-H), 7.64 (q, J = 0.7 Hz, 1H, Ar-H), 7.81 (d, J = 7.6 Hz, 1H, Ar-H), 8.00 (dd, J = 7.3, 1.6 Hz, 1H, Ar-H), 8.94 (s, 1H, Ar-H), 9.02 (s, 1H, OH), 9.95 (s, 1H, NH), ppm; 13C NMR (125 MHz, DMSO-d6) δ 21.3, 21.3, 110.9, 119.1, 123.5, 124.3, 125.0, 126.3, 127.4, 127.4, 127.6, 128.5, 128.8, 129.4, 130.3, 133.9, 136.5, 137.7, 138.0, 138.9, 139.3, 142.6, 152.8, 153.6; MS (m/z, %): 432 (M+, 93); Anal. Calcd for C27H20N4O2: C, 74.98; H, 4.68; N, 12.95%. Found: C, 74.86; H, 4.75; N, 13.06%.
2.1.10. 4-(4-bromophenyl)-5-(5-hydroxy-10-methylbenzo[a]phenazin-6-yl)-1,3-dihydro-2H-imidazol -2-one (6h)
Brown powder; yield 0.432 g (94%), m.p 256–258 °C; IR (KBr) (νmax cm−1): 3035, 2890, 1668, 1581, 1550, 1509, 1480, 1440, 1408, 1382, 1339, 1265, 1211, 1162, 1061, 1022, 976, 944, 874, 819, 758 cm–1; 1H NMR (500 MHz, DMSO-d6) δ 2.42 (s, J = 0.9 Hz, 3H, CH3), 7.37 (ddd, J = 7.4, 1.5, 0.8 Hz, 1H, Ar-H), 7.44 (dd, J = 7.4, 1.7 Hz, 1H, Ar-H), 7.46–7.51 (m, 1H, Ar-H), 7.48–7.57 (m, 3H, Ar-H), 7.55–7.62 (m, 3H, Ar-H), 7.83 (d, J = 7.5 Hz, 1H, Ar-H), 8.00 (dd, J = 7.3, 1.6 Hz, 1H, Ar-H), 8.95 (s, 1H, Ar-H), 9.03 (s, 1H, OH), 9.95 (s, 1H, NH) ppm; 13C NMR (125 MHz, DMSO-d6) δ 21.3, 110.9, 119.1, 124.0, 124.3, 124.7, 125.0, 127.4, 127.5, 127.6, 127.7, 127.9, 128.6, 129.5, 130.3, 131.2, 133.9, 136.5, 137.6, 138.0, 138.9, 142.6, 152.8, 153.6; MS (m/z, %): 496 (M+, 87); Anal. Calcd for C26H17BrN4O2: C, 62.79; H, 3.45; N, 11.27%. Found: C, 62.85; H, 3.56; N, 11.35%.
2.1.11. 4-(4-chlorophenyl)-5-(5-hydroxy-10-methylbenzo[a]phenazin-6-yl)-1, 3-dihydro-2H-imidazole-2-one (6i)
Brown powder; yield 0.397 g (91%), m.p 259–261 °C; IR (KBr) (νmax cm−1): 3015, 2895, 1669, 1584, 1507, 1482, 1408, 1382, 1338, 1272, 1210, 1159, 1079, 1022, 975, 943, 818, 758 cm–1; 1H NMR (500 MHz, DMSO-d6) δ 2.43 (s, J = 0.9 Hz, 3H), 7.31–7.40 (m, 3H, Ar-H), 7.43–7.58 (m, 4H, Ar-H), 7.62–7.66 (m, 1H, Ar-H), 7.81 (d, J = 7.6 Hz, 1H, Ar-H), 8.00-8.25 (m, J = 7.3, 1.6 Hz, 3H, Ar-H), 8.94 (s, 1H, OH), 9.95 (s, 1H, NH) ppm; 13C NMR (125 MHz, DMSO-d6) δ 21.3, 110.9, 119.1, 124.1, 124.3, 125.0, 127.4, 127.4, 127.5, 127.6, 127.7, 128.4, 128.5, 129.4, 130.3, 133.9, 136.5, 137.4, 137.5, 138.0, 138.9, 142.6, 152.8, 153.6; MS (m/z, %): 452 (M+, 66); Anal. Calcd for C26H17ClN4O2: C, 68.95; H, 3.78; N, 12.37%. Found: C, 68.91; H, 3.67; N, 12.47%.
3. Results and Discussion
The phase structure, crystal size, and crystallinity are the essential factors of the composite catalyst. shows the XRD patterns of the catalysts. Peaks at angles 25.3°, 37.8°, 48.0°, 54.4°, 62.7°, 69.3°, and 75.2°, respectively (JCPDS 21-1272). As for HPA@Nano-TiO2, the same characteristic peaks as those of pristine TiO2 were also observed, indicating that the anatase phase of TiO2 was preserved well in the composite photocatalyst. No diffraction peaks of the H3PW12O40 appeared, which presumably was due to the low content incorporation of H3PW12O40 (Citation38).
The FT-IR spectra of H3PW12O40/TiO2 were characterized and presented in . For the pristine H3PW12O40, the characteristic vibrational frequencies related to the Keggin unit appeared at 1083, 991, 895, and 815 cm−1, which were assigned to the vibrations of the P-O bonds of the PO4 units, W = O bonds, and two W-O-W bonds. After the TiO2 introduction, the vibrational frequencies changed to 1076, 976, 892, and 813 cm−1, respectively () (Citation35).
The FESEM and TEM images of the TiO2, and H3PW12O40/nano TiO2 samples are shown in . In (a), TiO2 particles are shown in the form of brass with an approximate size of 30-45 nm. while H3PW12O40/nano-TiO2 particles show a well-distributed spherical shape with diameters of about 20-30 nm ((c)). (d) gives the TEM micrograph of nanocrystalline H3PW12O40/TiO2, whose crystallite size was in the range of 10–20 nm. As can be seen in ((b)), TiO2 nanoparticles present spherical shapes being agglomerated in asymmetric formations. The TEM and HRTEM images reveal that the HPW/TiO2 sample possesses disordered microporous structures, and the macropores are preserved throughout the entire material ((c–e)). (c) displays that partly macropores are connected by the holes on the wall of the macropores. This result could be due to the shrinkage of the HPW/TiO2 composite during calcination. (c) evidences the existence of ordered mesopores in the macropore walls. The mesopore and macropore structures are further confirmed by transmission electron microscopy (TEM) micrographs, showing that the HPW/TiO2 sample represents macropores, which are interconnected through hexagonally ordered mesopores of ca. 10-20 nm.
Figure 4. FESEM images of prepared TiO2 (a), TEM image TiO2 (b) and H3PW12O40/nano-TiO2 (c), TEM and HRTEM image (d, e).
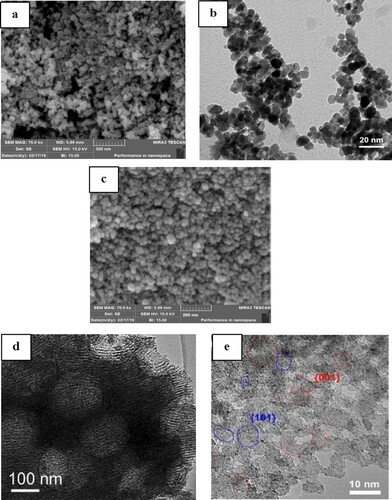
Atomic force microscopy (AFM) analysis of the nanocatalyst confirms the presence of spherical particles in the final catalyst (). The higher the effective level, the higher the catalyst activity or efficiency in product synthesis. The AFM image showed the presence of nanoparticles whose particle size and morphology were close to SEM images.
The thermal behavior of representative samples H3PW12O40/nano-TiO2 sample was investigated via TG analyses (). A decrease in the weight percentage of the catalyst of about 100 °C is due to the removal of water molecules and another decrease of 280 °C is observed, which indicates the high thermal stability of the catalyst.
To investigate the surface areas and pore size distributions of H3PW12O40@nano-TiO2 composite photocatalyst, the N2 adsorption-desorption isotherms were analyzed, as presented in . The pore size distributions indicated that TiO2 presented a relatively narrow distribution ranging from 35 nm to 45 nm. Taking into account the morphology of the material observed by TEM, the small pores should be the intra nanoparticle pores. The BET surface area of the prepared TiO2 nanoparticles was 186.25 m2.g−1 and the BET surface area of the commercial was 50 m2.g−1. A larger surface area provides more surface-active sites for the adsorption of the reactive molecules, which leads the photocatalytic process to be more efficient. We can conclude that the nanoparticles prepared by us might have good photocatalytic activities. BET surface area (177.9 m2.g−1), pore volume (0.4390 cm3.g−1), and average pore size (3.84 nm) of H3PW12O40/nano-TiO2 were higher than those of TiO2 (158.6 m2.g−1; 0.4240 cm3.g−1) due to the formation of H3PW12O40/nano-TiO2 framework via Ti-O-W bonds (Citation35–37).
Figure 7. Nitrogen adsorption-desorption isotherms and the corresponding pore size distributions of the samples (a) TiO2; (b) H3PW12O40/TiO2.
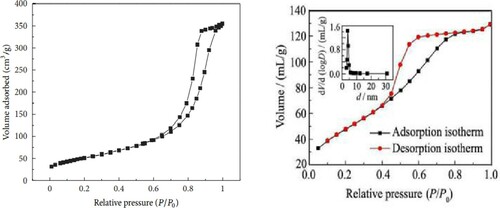
Using the relation σ = 1/ρ the electrical conductivity of the material can be obtained, as shown in . The electrical conductivity increases with temperature from σ = 3.78·10−8 S cm−1 at 145 K to σ = 6.04·10−7 S cm−1 at 300 K.
Figure 8. The conductivity σ (T) of H3PW12O40/nano-TiO2 core-shell nanoparticles obtained by hydrothermal method.
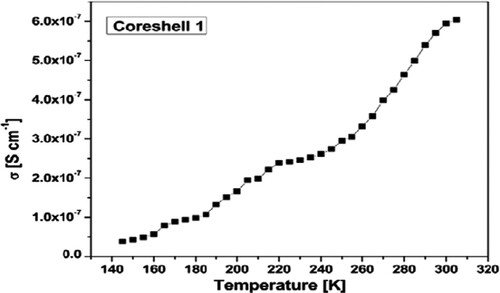
XPS analysis is applied to investigate the chemical state of the H3PW12O40@nano-TiO2 catalyst. As shown in (a), the survey scan XPS spectrum displays that the composites contain Ti, O, P, and W, Peaks appeared at approximately 464.4 and 458.8 eV can be attributed to the binding energies of O 1s, Ti 2p1/2, and Ti 2p3/2, originated from lattice oxygen of TiO2 ((b and c)) Moreover, the characteristic 4f5/2 and 4f7/2 peak of W atom in ) can be found at 37.6 and 35.6 eV, suggesting the WVI oxidation state. The high-resolution P 2p and W 4f XPS spectra also indicate the introduction of HPW in the catalyst ()).
3.1. Catalyst recyclability
We also examined catalyst recycling using an option (). After the reaction, 5 ml of water was added and filtered to separate the precipitate. After complete washing of the solid product, the water evaporates under pressure and is used again. The results showed that the catalyst can be reused 6 consecutive times (). One of the advantages of heterogeneous catalysts is their reusability. The recycled catalyst was reused six times in similar reaction conditions. The results show that recyclability has not significantly decreased the catalytic performance.
Figure 10. The investigation of the recycling of H3PW12O40@nano-TiO2 (b) and XRD analysis of fresh and recycled catalysts (a).
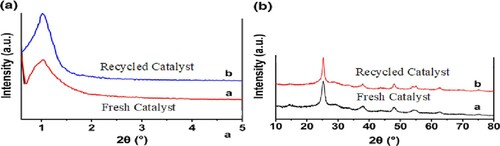
Table 2. Reusability of H3PW12O40-nano-TiO2 for Compound 6 h.
A hot filtration test was performed by separating the H3PW12O40/TiO2 catalyst from the reaction mixture after 5 min in the first cycle of the reaction. The recovered nanocatalyst was collected and then dried at 100 0C. This may lead to a slight increase of the yield to 59% from 55% after the hot filtration test. Furthermore, disruptions, such as hot filtration, may deactivate an existing active dissolved metal, leading to the incorrect conclusion that there are no active homogeneous catalytic species before the filtration ().
displays the UV-Vis spectra of TiO2, H3PW12O40/TiO2. The absorption spectra of all the catalysts exhibit strong absorption in the range of 200–400 nm, this finding indicates that the introduction of the rare earth element in the H3PW12O40/TiO2 framework results in the changes in the coordination environment of H3PW12O40/TiO2 crystalline (Citation38).
3.2. Effect of tungstophosphoric acid loading on TiO2
After extensive screening, we found that the optimized best yields and time profiles were obtained when the reaction was carried out in the presence of 0.03 g of 60% H3PW12O40@ nano-TiO2 under MWI at 300 W under solvent-free, which afforded the corresponding 4-(5-hydroxybenzo[a]phenazin-6-yl)−5-phenyl-1, 3-dihydro-2H-imidazol-2-one in 10 min with 95% of yield (, entry 12). Increasing the amount of 60% H3PW12O40@ nano-TiO2 to more than 0.06 g showed no substantial improvement in the yield, whereas the yield decreased by decreasing the amount of the catalyst to 0.015 g. Moreover, the reaction did not proceed efficiently in the absence of H3PW12O40@nano-TiO2 even after 60 min under MWI. In this experiment, no other solvents were tested because of the green chemistry concept.
Table 3. Optimization of reaction conditions of compound 6 h.
In this context, we have demonstrated a highly efficient and green process for the preparation of various potentially biologically active functionalized 4-(5-hydroxybenzo[a]phenazin-6-yl)-5-phenyl-1, 3-dihydro-2H-imidazol-2-one derivatives via a single-pot, two-step, four-component cyclo-condensation reaction using H3PW12O40@nano-TiO2, highly reactive, and eco-friendly solid acid catalyst under MWI in an aqueous medium. To determine the catalytic behavior of H3PW12O40-nano-TiO2, the suggested mechanism for the formation of the products is shown in . Based on this mechanism, at first, 2-hydroxynaphthalene-1,4-dione 1 tautomerizes to intermediate 11. The primary condensation of 4-hydroxy-1,2-naphthoquinone 11 with benzene-1,2-diamine 2 obtain benzo[a]phenazin-5-ol 3. A reaction pathway involves the formation of an intermediate (A) medium when reacted with the urea group after being added to the carbonyl group or the replacement of the side-chain hydroxy group. Based on the second route, Arylglyoxal and Urea developed the open shaft (B), which is made after reacting with the intermediate middle (A) benzo [a] phenazine-5-ol, which is caused by loss of water during the ringing process the desired product is obtained.
4. Conclusion
In summary, we have described a simple, efficient, and environment-friendly one-pot procedure for the synthesis of 4-(5-hydroxybenzo[a]phenazin-6-yl)-5-phenyl-1, 3-dihydro-2H-imidazol-2-one derivatives by using catalytic amount under microwave irradiation conditions. Short reaction times, high yields, high atom economy, excellent chemoselectivity, simple experimental procedure, and the easily work-up procedure associated with the efficiency of the synthesized nanocatalyst are the highlighted points of the present method. Moreover, this sequential protocol includes some important aspects like the use of MWI as a partially renewable energy source for the direct heating of the reaction mixture and the application of H3PW12O40@nano-TiO2 as a reusable and recoverable catalyst.
Acknowledgments
We gratefully acknowledge financial support from the Yazd Branch, Islamic Azad University, Yazd.
Disclosure statement
No potential conflict of interest was reported by the author(s).
References
- Zhang, G.P.; Chen, D.Y. Fabrication of Bi2MoO6/ZnO Hierarchical Heterostructures with Enhanced Visible-Light Photocatalytic Activity. Appl. Catal. B. Environ. 2019, 250, 313–324.
- Thangavel, S.; Krishnamoorthy, K.; Kim, S.J.; Venugopal, G. Designing ZnS Decorated Reduced Graphene-Oxide Nanohybrid via Microwave Route and Their Application in Photocatalysis. J. Alloy. Comp. 2016, 683, 456–462.
- Moussa, H.; Girot, E.; Mozet, K. ZnO Rods/Reduced Graphene Oxide Composites Prepared via a Solvothermal Reaction for Efficient Sunlight-Driven Photocatalysis. Appl. Catal B. Environ. 2016, 185, 11–21.
- Taheri , M; Mohebat, R. A magnetically-separable TiO2-H3PW12O40@Fe3O4/EN as magnetic core-shell nanoparticles on metal-organic framework MIL-101(Cr). J Mater Science: Mater Electro. 2021, 32, 3104–3115.
- Zhang, M.; Liu, Y.H.; Shang, Z.R.; Hu, H.C.; Zhang, Z.H. Supported Molybdenum on Graphene Oxide/Fe3O4: An Efficient, Magnetically Separable Catalyst for one-pot Construction of Spiro-Oxindole Dihydropyridines in Deep Eutectic Solvent Under Microwave Irradiation. Cat. Commun. 2017, 88, 39–44.
- Chen, M.N.; Mo, L.P.; Cui, Z.S.; Zhang, Z.H. Magnetic Nanocatalysts: Synthesis and Application in Multicomponent Reactions. Curr. Opin. Gree. Sustain. Chem 2019, 15, 27–37.
- Alberti, S.; Caratto, V.; Peddis, D.; Belviso, C.; Ferretti, M. Synthesis and Characterization of a new Photocatalyst Based on TiO2 Nanoparticles Supported on a Magnetic Zeolite Obtained from Iron and Steel Industrial Waste. J. Alloy Comp. 2019, 797, 820–825.
- Deng, C.; Hong, R.J.; Jing, M. Photocatalytic Performance of TiO2 Thin Film Decorated with Cu2O Nanoparticles by Laser Ablation. Opt. Mater. 2019, 94, 130–137.
- Chiarello, G.L.; Dozzi, M.V.; Selli, E. TiO 2 -Based Materials for Photocatalytic Hydrogen Production. J. Energy. Chem. 2017, 26, 250–258.
- Zhang, K.; Liu, H.F.; Nie, C.H. Controllable Synthesis of Honeycomb-Structured ZnO Nanomaterials for Photocatalytic Degradation of Methylene Blue. Mater. Lett. 2019, 253, 30–33.
- Ye, Y.H.; Li, X.; Ynterma, D.; Rijnaarts, H.H.M. Significant Enhancement of Micropollutant Photocatalytic Degradation Using a TiO2 Nanotube Array Photoanode Based Photocatalytic Fuel Cell. Chem. Eng. J. 2018, 354, 553–562.
- Yu, J.G.; Yu, X.X. Hydrothermal Synthesis and Photocatalytic Activity of Zinc Oxide Hollow Spheres. Environ. Sci. Technol. 2008, 42, 4902–4907.
- Ma, S.S.; Xue, J.J.; Zhou, Y.M.; Zhang, Z.W. Photochemical Synthesis of ZnO/Ag2O Heterostructures with Enhanced Ultraviolet and Visible Photocatalytic Activity. J. Mater. Chem. A 2014, 2, 7272–7280.
- Niknam, K.; Saberi, D.; Sadegheyan, M.; Deris, A. Silica-bonded S-Sulfonic Acid: An Efficient and Recyclable Solid Acid Catalyst for the Synthesis of 4,4′-(Arylmethylene)bis(1H-Pyrazol-5-ols). Tetrahed. Lett. 2010, 51, 692–4.
- Giguere, R.J.; Bray, T.L.; Duncan, S.M.; Majetich, G. Application of Commercial Microwave Ovens to Organic Synthesis. Tetrahedron Lett. 1986, 27, 4945–8.
- Xin, Y.; Nagata, T.; Kato, K.; Shirai, T. Microwave-Assisted Synthesis of Pt Nanoparticles via Liquid-Phase Polyol Reaction for Catalytic Volatile Organic Compound Elimination. ACS. Applied Nano. Mater. 2022, 5, 4305–15.
- Wu, H.; She, Y.; Fan, L.Y. Stereoselective Synthesis of β-Amino Ketones via Direct Mannich-Type Reaction Catalyzed with Silica Sulfuric Acid. Tetrahedron 2007, 63, 2404–8.
- Bloxham, J.; Dell, C.P.; Smith, C. Preparation of Some New Benzylidenemalononitriles by an SNAr Reaction: Application to Naphtho[1,2-b]Pyran Synthesis. Heterocycles 1994, 38, 399–08.
- Pan, L.; Olson, D.H.; Ciemnolonski, L.R.; Heddy, R.; Li, J. Separation of Hydrocarbons with a Microporous Metal-Organic Framework. Angew. Chem. Int. Ed. 2006, 118, 632–635.
- Jhung, S.H.; Lee, J.-H.; Yoon, J.W.; Serre, C.; Chang, J.S. Microwave Synthesis of Chromium Terephthalate MIL-101 and its Benzene Sorption Ability. Adv. Mater. 2006, 19, 121–124.
- Tong, M.; Liu, D.; Yang, Q.; Devautourvinot, S.; Maurin, G.; Zhong, C. Influence of Framework Metal Ions on the dye Capture Behavior of MIL-100 (Fe, Cr) MOF Type Solids. J. Mater. Chem. A. 2013, 1, 8534–8537.
- Zhou, M.; Wu, Y.N.; Qiao, J.; Zhang, J.; Mcdonald, A.; Li, G.; Li, F. The Removal of Bisphenol A from Aqueous Solutions by MIL-53(Al) and Mesostructured MIL-53(Al). J. Colloid. Interface. Sci. 2013, 405, 157–163.
- Eskandari, K.; Pourshojaei, Y.; Haghani, F.; Shabani, M.; Asadipour, A. Synthesis, and Molecular Modeling of bis(3-(Piperazine-1-yl)Propyl)Tungstate (BPPT) Nanoparticles, and its First Catalytic Application for one-pot Synthesis of 4H-Chromene Derivatives. Heliyon 2019, 5, e02426–2430.
- Ohata, Y.; Tomonaga, M.; Watanabe, Y.; Tomura, K.; Kimura, K.; Akaki, T.; Adachi, K.; Kodama, E.N.; Matsuzaki, Y.; Hayashi, H. Antiviral Activity and Resistance Profile of the Novel HIV-1 Non-Catalytic Site Integrase Inhibitor JTP-0157602. J. Virology 2022, 96, 1843–21.
- Maes, M.; Schouteden, S.; Alaerts, L.; Depla, D.; Vos, D.E.D. Extracting organic contaminants from water using the metal-organic framework Cr (OH)${OCCH-CO}. Phys. Chem. Chem. Phys. 2011, 13, 5587–5589.
- Veisi, V.; Ozturk, T.; Karmakar, B.; Tamoradi, T.; Hemmati, S. In Situ Decorated Pd NPs on Chitosan-Encapsulated Fe3O4/SiO2-NH2 as Magnetic Catalyst in Suzuki-Miyaura Coupling and 4-Nitrophenol Reduction. Carbohydrate. Polymers. (Basel) 2020, 235, 115966–115974.
- Zhang, W.; Veisi, H.; Sharifi, R.; Salamat, D.; Karmakar, B. Fabrication of Pd NPs on Pectin-Modified Fe3O4 NPs: A Magnetically Retrievable Nanocatalyst for Efficient C–C and C–N Cross Coupling Reactions and an Investigation of its Cardiovascular Protective Effects. Int. J. Biol. Macromol. 2020, 160, 1252–1262.
- Li, Y.; Song, Y., Ma, A., et al. Surface Immobilization of TiO2 Nanotubes with Bone Morphogenetic Protein-2 Synergistically Enhances Initial Preosteoblast Adhesion and Osseointegration. Biomed. Res. Int. 2019, 23, 1206–1223.
- Veisi, H.; Hemmati, S.; Safarimehr, P. In Situ Immobilized Palladium Nanoparticles on Surface of Polymethyldopa Coated-Magnetic Nanoparticles (Fe3O4@PMDA/Pd): A Magnetically Recyclable Nanocatalyst for Cyanation of Aryl Halides with K4[Fe(CN)6]. J. Cat. 2018, 365, 204–212.
- Ghorbani Shahna, F.; Bahrami, A.; Alimohammadi, I. Chlorobenzene Degeradation by non-Thermal Plasma Combined with EG-TiO2/ZnO as a Photocatalyst: Effect of Photocatalyst on CO2 Selectivity and Byproducts Reduction. J. Hazar. Mater 2017, 324, 544–553.
- Jafari, S.; SaeidAzizian, S.; Jaleh, B. Enhancement of Methyl Violet Removal by Modification of TiO2 Nanoparticles with AgI. J. Indus. Engin. Chem. 2012, 18, 2124–2128.
- FeiziMohazzab, B.; Jaleh, B.; Nasrollahzadeh, M. Laser Ablation-Assisted Synthesis of GO/TiO2/Au Nanocomposite: Applications in K3[Fe(CN)6] and Nigrosin Reduction. Mole. Cat 2019, 473, 110401–110410.
- Eslamipanah, M.; Jaleh, B.; Feizi Mohazzab, B. Facile Synthesis and Electrochemical Hydrogen Storage of Bentonite/TiO2/Au Nanocomposite. Int. J. Hydrog. Energy 2020, 45, 33771–33788.
- Jaleh, B.; Sheikhi Shahidzadeh, E.; Azizian, S.; Shokoufeh Ghahri Saremi, S. Effects of UV Irradiation Treated Polycarbonate Substrates on Properties of Nanocrystalline TiO2 sol-gel Derived Thin Films. J. Inter. 2018, 2, 73–79.
- Veisi, H.; Mohammadi, L.; Hemmati, S.; Tamoradi, T.; Mohammadi, P. n Situ Immobilized Silver Nanoparticles on Rubia tinctorum ExtractCoated Ultrasmall Iron Oxide Nanoparticles: An Efficient Nanocatalyst with Magnetic Recyclability for Synthesis of Propargylamines by A3 Coupling Reaction. ACS. Omega. doi: 10.1021/acsomega.9b01720.
- Veisia, H.; Tamoradia, T.; Rashtiania, A.; Hemmatia, S.; Karmakar, B. Palladium Nanoparticles Anchored Polydopamine-Coated Graphene oxide/Fe3O4 nanoparticles (GO/Fe3O4@PDA/Pd) as a Novel Recyclable Heterogeneous Catalyst in the Facile Cyanation of Haloarenes Using K4[Fe(CN)6] as Cyanide Source. J. Ind. Eng. Chem 2020, 90, 379–388.
- Clifford, E.R.; Bradley, R.W.; Wey, L.T. New Phenazine Based Anolyte Material for High Voltage Organic Redox Flow Batteries. Chem. Comuni 2021, 57, 2986–2989.
- Khurana, J.M.; Chaudhary, A.; Lumb, A.; Nand, B. An Expedient Four-Component Domino Protocol for the Synthesis of Novel Benzo[a]Phenazine Annulated Heterocycles and Their Photophysical Studies. Green. Chem. 2012, 14, 2321–2327.
- Saluja, P.; Chaudhary, A.; Khurana, J.M. Synthesis of Novel Fluorescent Benzo[a]Pyrano[2,3-c]Phenazine and Benzo[a]Chromeno[2,3-c]Phenazine Derivatives via Facile Four-Component Domino Protocol. Tetra. Lett. 2014, 55, 3431–3435.
- Rajeswari, M.; Khanna, G.; Chaudhary, A.; Khurana, J.M. Multicomponent domino process for the synthesis of some novel benzo[a]chromenophenazine fused ring systems using H2SO4, phosphotungstic acid, and[NMP]H2PO4. Synth. Commun. 2015, 45, 1426–1432.
- Yazdani-Elah-Abadi, A.; Mohebat, R.; Maghsoodlou, M.T.; Heydari, R. One-Pot, Sequential Four-Component Synthesis of Benzo[a]chromeno[2,3-c]phenazine Derivatives Using SiO2–SO3H as an Efficient and Recoverable Catalyst Under Conventional Heating and Microwave Irradiation. Poly. Arom. Com. 2018, 38, 92–101.
- Taheri, M.; Mohebat, R.; Moslemin, M.H. Multi-Component Reaction Synthesis of Novel 3-phenyl-3,4-dihydro-2H-benzo[a][1,3]oxazino[5,6-c]Phenazine Derivatives Catalyzed by Reusable ZnO-PTA@Fe3O4/EN-MIL-101(Cr) Nano Powder at Room Temperature. Green. Chem. Lett. Rev. 2020, 13, 179–191.
- Taheri, M.; Mohebat, R.; Moslemin, M.H. Synthesis of benzo[a]furo[2, 3-c]Phenazine Derivatives Through an Efficient, Rapid and via Microwave Irradiation Under Solvent-Free Conditions Catalyzed by H3PW12O40@Fe3O4-ZnO for High-Performance Removal of Methylene Blue. Arti. Cell. Nano. Biotech. 2021, 49, 250–260.
- Ma, J.; Zhu, C.; Xu, Y. Photocatalytic Degradation of Gaseous Benzene with H3PW12O40/TiO2/Palygorskite Composite Catalyst. J. Sau. Chem. Soc. 2017, 21, 132–142.
- Taheri, M.; Mohebat, R.; Moslemin, M.H. Synthesis of one-pot pyrazolo[4’,3':5,6]pyrano[2,3-c] phenazin-15-yl) methanone derivatives via a multi-component using Fe3O4@TiO2-SO3H as a recoverable magnetic catalyst under microwave irradiation. Green. Chem. Lett. Rev. 2020, 13, 165–178.
- Taheri, M.; Mohebat, R.; Moslemin, M.H. Microwave-Assisted Multi-Component Green Synthesis of Benzo[α]Furo[2, 3-c]Phenazine Derivatives via a Magnetically-Separable Fe3O4@rGO@ZnO-HPA Nanocatalyst Under Solvent-Free Conditions. Poly. Arom. Com. 2021, in press.