ABSTRACT
Six samples of bio-capped copper oxide nanoparticles were made from two bio-waste extracts: Punica granatum L. peels and Psidium guajava Linn. leaves. Extraction methods included soaking in water or hydroethanol and boiling in water. The samples were characterized using FT-IR, XRD, SEM, and TGA. Results showed organic residue capping on CuO nanoparticles varied based on the extract and capping medium used. The thermal stability of all CuO samples was observed to be high, as recorded from TGA patterns and confirmed by EDX analysis, which showed a high content of copper ranging from 10.1 to 36.1%. TEM analysis revealed an average particle size of less than 20 nm for all six samples, suggesting a similarity in size. The soaking technique produced the most stable bio-capped CuO nanoparticles with a high negative zeta potential value. According to the study, the CuO samples synthesized from aqueous extracts obtained through soaking showed the highest antibacterial activity. This could be attributed to the high oxygen ratio, which was confirmed via EDX analysis. The bio-capped CuO was effective against multidrug- resistant gram-positive bacteria MRSA, and C. albicans. A mechanism was proposed to explain how the capping media affected the antimicrobial activity of the bio-capped CuO nanoparticles.
GRAPHICAL ABSTRACT
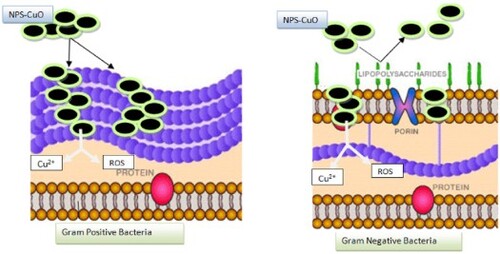
1. Introduction
Nowadays, the biosynthesis of metals and metal oxide nanoparticles is increasingly replacing traditional physical and chemical methods due to their low energy consumption, reduced toxicity, and environmental sustainability. Green synthesis relies on plant extracts or microorganisms, making it an eco-friendly approach. Recently, researchers have used non-edible plant waste precursors (Citation1) to prepare biogenic metal oxides (Citation2,Citation3) making green synthesis both economically and environmentally efficient. The process of biosynthesis is influenced by a number of factors, with the choice of extraction method and solvent medium being crucial in determining the success of the process. These two factors play a key role in influencing the outcome of the biosynthesis process. However, the quality of the extract ultimately depends on several factors, such as the type of plant material, the extraction conditions, and the intended use of the extract (Citation4).
Psidium guajava Linn., commonly known as guava, which belongs to the Myrtaceae family (Citation5), and is known for its antioxidant, antibacterial, and anti-inflammatory properties (Citation6). Guava leaves contain phenolic compounds and flavonoids that exhibit potent antioxidant activity. The primary bioactive components in guava leaves include gallic acid, caffeic acid, guaijaverin (Citation7), tannins, carotenoids, and triterpenoids (Citation8). The extract from its leaves is used as a natural source for producing copper oxide nanoparticles (NPs) owing to their rich content of polyphenols, flavonoids, and tannins found in both the leaves and fruit (Citation9). These compounds act as natural reducing and stabilizing agents in the biosynthesis process. Guava leaves derived compounds of flavonoids can inhibit bacterial pathogens of Gram + ve and Gram -ve due to the presence of terpinene and pinene in the plant leaf extract (Citation10). Another promising bio-waste precursor is Punica granatum L., commonly known as pomegranate, which belongs to the punicacea family (Citation11), is one of the oldest edible fruits widely cultivated in many tropical and subtropical regions, including the Middle East (Citation12). Pomegranate peel extract, a natural reducing agent, has been used in the biosynthesis of copper oxide NPs due to its unique chemical composition and properties. The peel extract of pomegranate contains high levels of polyphenols, which are known to have reducing and stabilizing properties (Citation13). These properties make pomegranate extracts a suitable candidate for reducing copper ions and stabilizing the formed copper oxide nanoparticles (Citation14).
Several studies have investigated the chemical composition of pomegranate extracts obtained by boiling. These studies have identified a wide range of compounds, including phenolic acids, flavonoids, ellagitannins, and other polyphenolic compounds (Citation15). Soaking is another method commonly used to extract bioactive compounds from pomegranate, including flavonoids, hydrolyzable tannins, ascorbic acid, and polyphenols. Furthermore, the aqueous extraction of pomegranate peel was observed to effectively control both gram-positive and gram-negative bacteria (Citation8). The hydroethanol extracts obtained from pomegranate peel exhibited a similar pattern to the soaking process. Nevertheless, the concentration of flavonoids and total phenols was found to decrease (Citation12). On the contrary, boiling guava leaves resulted in the presence of various components such as glycosides, flavonoids, saponins, phenols, terpenoids, and tannins (Citation9). However, the phenolic compound content extracted using hydroethanolic solvent was higher than water (Citation8). The use of ethanol and methanol solvents for extracting guava leaves exhibited a strong inhibitory effect against gram-positive bacteria (Citation16).
The emergence of multidrug-resistant bacteria and their role in increasing mortality rates is a growing concern worldwide (Citation17). Methicillin-resistant Staphylococcus aureus (MRSA) is a gram-positive bacterium that has recently developed resistance to a broad range of antibiotics (Citation18). In addition, there is an increasing threat from infections caused by carbapenemase-producing bacteria, such as Klebsiella pneumoniae, Pseudomonas aeruginosa, and Acinetobacter baumannii (Citation19).
The primary objective of this study is to evaluate the influence of various extraction methods on the effectiveness of two bio-wastes, guava leaves, and pomegranate peel, in the synthesis of copper oxide nanoparticles. Additionally, the study aims to investigate the comparative antimicrobial efficacy of the resulting bio-capped nanoparticles against a range of multidrug-resistant bacteria and fungi. Finally, the study seeks to propose a plausible mechanism underlying the antimicrobial properties of the bio-capped CuO nanoparticles.
2. Experimental methods
2.1 Chemicals and materials
Both pomegranate peel powder and guava leaves were purchased from the Egyptian market (Figure 1 and 2 in the supplementary file). They were washed several times with deionized water and dried in open air at room temperature (25° ± 2) before use. Copper sulfate (CuSO4.5H2O) and absolute ethanol were purchased from S.d. Fine-chemicals. Itd, India. None of the chemicals used were purified before usage.
2.2 Preparation of extract from Punica granatum L. peel and Psidium guajava Linn. leaves
The extraction process of both pomegranate peels and guava leaves has been done using three different media (boiling, soaking in water, and hydroethanol 1:1) to produce six different extracts. Forty grams of pomegranate peel powder were added to 500 ml of deionized water. After boiling the mixture for 30 min, it was filtered. The filtrate is used in the preparation of copper oxide nanoparticles and was given the name PP- extract-1. Another forty grams of pomegranate peel have been soaked in 500 ml of hydroethanol solution (1:1) for 24 h. The filtrate of this mixture was given the name PP-extract-2. A third portion of Forty grams pomegranate peel was soaked in 500 ml deionized water for 24 h. The mixture was filtrated, and the filtrate was given the name PP-extract-3. Applying the same previous procedures to prepare another 3 extracts with replacing the pomegranate peel by guava leaves to produce GL-extracts 1, 2, and 3 (Citation3,Citation14).
2.3 The synthesis of bio-capped copper oxide nanoparticles (bio-capped CuO-NPs)
An amount of 50 ml of PP-extract-1 has been added to 40 mmol of CuSO4.5H2O (dropwise). For one hour, the mixture was heated to 120°C while being constantly stirred. After isolating a black precipitate (bio-capped CuO-NPs) from the previous step, the precipitate was washed with D.W. several times. Finally, the product was dried in an oven for a whole night at 60 °C. This product was given the name PP-CuO-1. The same procedure was followed to prepare the series PP-CuO-2 and PP-CuO-3 by replacing PP-extract-1 by PP-extract-2 and PP-extract-3, respectively. The other series GL-CuO-1, 2 and 3 were prepared by applying the same previous method by substituting PP-extracts by GL-extracts (Citation14).
2.4 Physicochemical analysis
The functional groups of the bio-capped CuO-NPs were investigated by FT-IR examination using a spectrophotometer (Shimadzu, 8400S) in range spectra from 400 to 4000 cm−1. The measurement is carried out through the traditional disc method, which is prepared from KBr and the tested sample. While the crystalline solid structure of biosynthesis nanoparticles was examined through Shimadzu XRD. The diffractometer with Cu Kα radiation (λ = 1.5418 Å) at a scanning speed of 0.2 S with applied operating voltage and current of 40 kV and 40 mA, respectively. The surface charge of all bio-capped CuO-NPs was tested by the Zeta potential technique, which was done via the Zetasizer Malvern Instruments, UK, at 633 nm. The TGA-DTA7300, Exstar instrument was used to determine the thermal stability of all nanomaterials that were biosynthesized in this paper. Finally, the morphology of the surface, the particle size and the percent composition for all bio-capped CuO-NPs were tested via SEM,TEM and EDX techniques using FEI, ISPECT S50, Czech Republic; FEI, Morgagni 268, Czech Republic; and Energy Dispersive X-Ray Fluorescence and Spectrometer, EDX-8000, and Shimadzu, USA, respectively. For SEM, the samples were mounted onto metallic stubs with double-sided carbon tape, while TEM samples were prepared by depositing the drops of sample dispersions onto TEM copper grids with carbon support film. SEM was operated at an accelerating voltage of 20 kV and TEM at 80 kV in bright-field imaging mode. Particle size was measured via TEM images using the image J software.
2.5 Evaluation activity
The antibacterial and anti-fungal activity of bio-capped CuO-NPs was investigated against gram-positive methicillin-resistant Staphylococcus aureus (MRSA), gram-negative multidrug-resistant Pseudomonas aeruginosa (MDR-PA), multidrug-resistant Acinetobacter baumannii (MDR-AB), and fungi, Candida albicans. The bacterial cultures were grown in Luria Bertani broth at 37 °C, while C. albicans was grown in Sabouraud Dextrose broth (SDB) at 28°C, respectively, for 24 h in a shaker incubator at 150 rpm. The bacterial culture was then washed with phosphate buffer saline. The pellet was then collected and re-suspended in brand-new LB broth.
2.6 Minimum inhibitory concentration (MIC)
In 96-well microtiter plates, the usual two-fold microbroth dilution technique was used to calculate the MIC values of bio-capped CuO-NPs at different concentrations (0.125–20 mg/ml) as per the protocol described previously. MICs are the lowest concentration of synthesized NPs at which no detectable growth of tested pathogens were seen (Citation20).
2.7 Minimum bactericidal and fungicidal concentration (MBC and MFC)
Further, after determining the MIC values, MBC and MFC values were examined as described by (Citation21). The detection of MBCs and MFCs was done by using a ten microliter suspension of bacteria or candida culture from microtitre plate wells that had no growth (i.e. MIC and above MIC values) and spreading it on an MHA and SDA plate for an additional 24 h at 37 °C. The values of MBC and MFC of bio-capped CuO-NPs could be defined as the minimum concentration of tested nanomaterials at which no microbial growth or less than 3 CFUs were detected (Citation20).
3. Results and discussion
3.1 Characterization studies
3.1.1 XRD analysis
The XRD patterns of the PP-CuO and GL-CuO nanoparticles in different capping media are illustrated in . The findings with respect to XRD patterns were used to recommend the chemical content and crystal structure of the synthesized samples. The existence of the 2θ peak at 43°in the samples synthesized from pomegranate extract suggests that the CuO-NPs are bio-capped and possess a monoclinic structure, and it is in a good match with JCPDS card no. 48–1548 (Citation22) and with literature results (Citation23). PP-CuO-1 is characterized by more diffraction 2θ peaks at 31.6°, 47, 56 and 74° which confirm the formation of CuO in this sample. The presence of an amorphous hump in case of PP-CuO-2 and PP-CuO-3 could be assigned to the presence of organic matter from the capping agent (high percentage of carbon confirmed from EDX results) which dominates over the CuO dissembling their diffraction peaks. In the other samples synthesized from the guava leaves extract, it can be observed that GL-CuO-3 has diffraction 2θ peaks at 25°, 32.5, 35.8 and 48° which are corresponding to crystal planes (110), (002), (111), and (202) respectively. The GL-CuO-3 nanoparticles are shown to be crystalline. It can be noticed that the position and the relative intensity of the diffraction peaks recorded match well with the standard phase CuO-NPs (JCPDS-80-1916)(2). On the other hand, the two samples GL-CuO-1 and GL-CuO-2 exhibit only a few weak peaks in their diffraction patterns at 2θ, which may be attributed to residual materials capping the CuO-NPs(7).
3.1.2 FT-IR analysis
FT-IR analysis was conducted on all bio-capped CuO-NPs samples. shows the FT-IR patterns of PP-CuO-1, PP-CuO-2, and PP-CuO-3, which closely resemble the pattern of the native pomegranate peel (Citation24). A broad band at 3409 cm−1 indicates the presence of stretching vibrations of O-H of carboxylic acid, phenol, or alcohol. A clear peak appears at approximately1700cm−1 indicating the presence of the C = O group for PP-CuO-1 and PP-CuO-3 only. The bands at 1600, 1225cm−1 represent the stretching vibration of O-H bond of polyphenol of both PP-CuO-1 and PP-CuO-3. Two adjacent bands at 1440 and 1320 cm−1 indicate the vibration of aromatic ring for PP-CuO-1 and PP-CuO-3. Finally, there are three significant peaks for all three bio-capped -NPs (PP-CuO-1, PP-CuO-2, and PP-CuO-3) at the range 1034, 880 and 668 cm−1 which represent C–O group, out of plane bending of H in aromatic ring and nano Cu-O group, respectively (Citation24,Citation25). This result clarifies that extraction in aqueous media liberates more organic residue than extraction via hydroethanol solvent.
The FT-IR pattern of another series of bio-capped NPs, GL-CuO, is represented in . For three bio-capped CuO-NPs samples, GL-CuO-1, GL-CuO-2, and GL-CuO-3, FT-IR spectra showed a broad band in range 3400 of cm−1 indicating the phenolic or alcoholic hydroxyl stretching. Additionally, a stretching vibration band appears at 1600 cm−1 representing C = O of the amide group. The existence of N-H group at 1445 cm−1 clearly appears in GL-CuO-2 and GL-CuO-3 only. Similarly, bands at 1362 cm−1 which represent the N-O group, appear in the GL-CuO-2 and GL-CuO-3 spectra (Citation3,Citation26). A sharp intensive peak appears at 1037 cm−1 which indicates the existence of ether linkage in all three bio-capped samples (Citation27). Finally, a peak indicating the formation of nano CuO appeared in the range at 676 cm−1 (Citation14). These FT-IR results demonstrate the high ability of the soaking technique to liberate more organic residue compared to the boiling method.
From all previous results, it can be observed that the prepared bio-capped CuO fabricated from pomegranate peel extracts was thoroughly mixed with different organic residues. Organic compounds such as polyphenols, carboxylic acids, alcohols, ethers, and aromatic compounds were found to cover the surace of PP-CuO-1and PP-CuO-3 to a greater extent than PP-CuO-2. This leads us to suggest that the extraction of pomegranate peel by water liberated more organic matter compared to extraction using ethanol. On the other hand, the extraction technique played a key role in the formation of bio-capped CuO-NPs using Guava leaves extracts. Finally, FT-IR investigation revealed that the preparation of CuO from both pomegranate peel extraction and guava leaves extraction resulted in CuO-NPs fully capped with different organic residues.
3.1.3 SEM, TEM and EDX analysis
The surface characteristics of the three biosynthesized PP-CuO series have been recorded in . All three images of SEM show regular spherical nanoparticles for the biosynthesized copper oxide with a uniform distribution. The SEM image also clarifies the aggregation of the biosynthesized CuO surface due to the existence of organic matter residue from pomegranate peel. Similarly, the other three SEM images of biosynthesized CuO-NPs produced from Guava leaves extraction are listed in . These three nanoparticles show a similar distribution and shape to biosynthesized copper oxide prepared by pomegranate peel, except GL-CuO-3, which shows regular spherical CuO-NPs with a more uniform distribution and less aggregation. This distribution could be due to less organic residue existing on the surface of GL-CuO-3 compared to GL-CuO-1 and GL-CuO-2. The data obtained from the TEM investigation for all bio-capped copper oxide nanoparticles was summarized in . This data also shows that the particle size of all bio-capped CuO is in the range less than 20 nm. However, a few aggregates were also observed for almost biosynthesized copper oxide, which confirms the existence of organic matter residue in capping form. The electron diffraction analysis of all the prepared specimens is shown in . It was seen that the electron diffraction of the CuO exhibited a well precise ring-like pattern with dots, confirming that the particles are well crystallized. The SAED rings are well matched with the XRD peaks, as shown earlier.
Figure 3. SEM micrographs of 6 samples at representative magnification of x10, 000. (a) PP-CuO-1, (b) PP-CuO-2, (c) PP-CuO-3, (d) GL-CuO-1, (e) GL-CuO-2, and (f) GL-CuO-3. All scale bars are 2 µm.
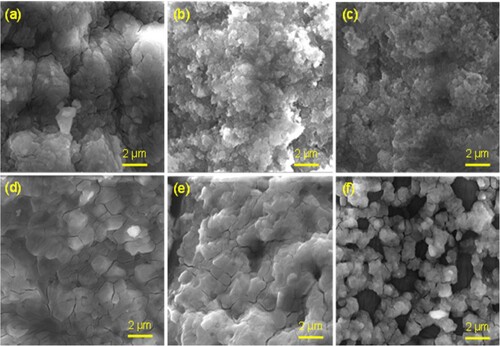
Figure 4. TEM micrographs of 6 samples at representative magnification of x10, 000. (a) PP-CuO-1, (b) PP-CuO-2, (c) PP-CuO-3, (d) GL-CuO-1, (e) GL-CuO-2, and (f) GL-CuO-3. All scale bars are 2 µm.
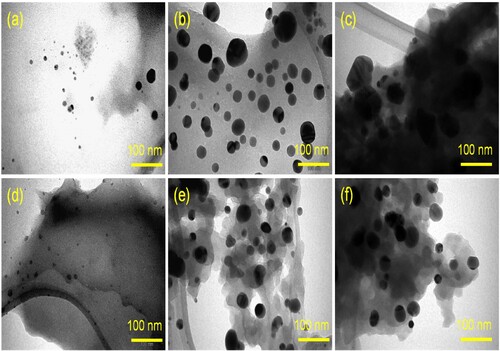
Figure 5. Selected-area electron diffraction (SAED) analysis (a) PP-CuO-1, (b) PP-CuO-2, (c) PP-CuO-3, (d) GL-CuO-1, (e) GL-CuO-2, and (f) GL-CuO-3.
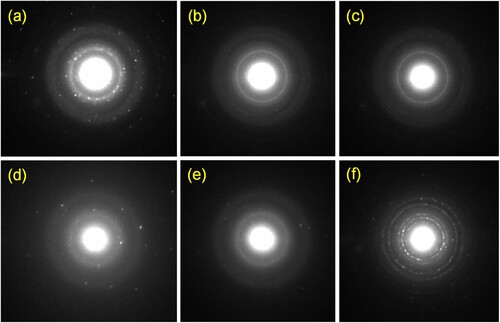
EDX analysis explores the elemental percentages of the bio-capped CuO, which varied from one sample to another (). In PP-CuO samples, the percentage of Cu fluctuated between 14.6 and 36.9%. The low percent of Cu in the sample PP-CuO-3 is compensated by an increase in the percent of carbon and oxygen, which could be assigned to the organic residue of the capping extracts. While in GL-CuO samples the percentage of Cu decreased compared to PP-CuO, it ranges from 10.1 to 19.1%, and carbon percent ranges from 12.0 to 40.0%. Results recommend that both types of plant material and the procedure of extraction played an important role in the composition percentage and quality of the nanoparticles synthesized in .
3.1.4 TGA and DTA analysis
displays the thermogravimetric curves of the six samples that were synthesized, illustrating the samples’ heating from 0°C to 600°C in the presence of oxygen. A summary of the TGA data is presented in , with the Tdm values for samples PP-CuO-1–3 and GL-CuO-1–3 recorded at 373, 386, and 388 and 373, 397, and 390, respectively. The broad endothermic peak recorded in all samples indicates dehydration. The mass loss observed in the first transition at T < 100 °C, was due to the evaporation of water content, which seems to be less than 1% in the six synthesized samples indicating low moisture content. It can be observed that for all samples, the weight residue percentage undergoes a significant decrease within the temperature range of 373–397 °C. This can be attributed to the thermal degradation of the organic residues of the capping agents (Citation28). The thermal analysis results suggest that the PP-CuO and GL-CuO samples exhibit greater thermal stability compared to previously synthesized bio-capped CuO samples (Citation29). Then, there is no significant weight loss observed above 400°C which indicates no further degradation. The high thermal stability is consistent with the percentage of CuO detected through Edx analysis.
Table 1. TGA data of six synthesized samples.
3.1.5 Zeta potential analysis
The Zeta potential measurement is a crucial characterization technique used to estimate the surface charge of synthesized bio-capped CuO nanoparticles samples (Figure 3 in the supplementary file), which in turn is indicative of their stability (Citation30). In this research, the zeta potential values recorded to GL-CuO series were −3.31,−11.3, and −12.5 mV respectively. On the other hand, for the PP-CuO series, the values recorded were −13.3, −6.4, and −15.1 mV respectively. These results demonstrate the dependence of the surface charge of the synthesized nanoparticles on the capping agent and the extraction method (Citation31, Citation32). The lowest recorded zeta potential value for the PP series was for PP-CuO-2, which was synthesized from a hydroethanol (1:1) extract. This may be attributed to the high concentration of ethanol (50% or more) in the extraction of pomegranate peel, which led to the formation of large aggregates of nanoparticles, reducing the stability and homogeneity of the resulting nanoparticle suspension. In contrast, the lowest value for the GL series was observed in GL-CuO-1, which was synthesized using the boiling method. This indicates that boiling may not be the most efficient method for extracting bioactive compounds from guava leaves, which can increase surface charge compared to other methods such as soaking. Finally, the results of physicochemical properties were summarized in (). The data shows that all the bio-capped CuO-NPs samples have similar surface shapes and particle sizes. However, there is a difference in the surface charge of the six bio-capped CuO-NPs samples. All six samples have a negative charge, but only PP-CuO-3 and GL-CuO-3 have the highest charge value. PP-CuO-3 and GL-CuO-3 also exhibit the highest thermal stability and a higher content of oxygen species, which play an important role in termination of microbial cells (Citation31).
Table 2. Physicochemical properties of six synthesized samples.
3.2 Antimicrobial activity of bio-capped CuO samples
The bio-capped CuO-NPs samples prepared by the green method were used to assess their antibacterial and anti-fungal properties against MRSA, MDR-AB, MDR-PA, and C. albicans by determining their MIC and MBC/MFC values using the broth dilution method. The MICs and MBCs/MFCs values of green synthesized NPs are shown in . It has been found that GL-CuO-3 showed maximum antimicrobial activity against gram-positive MRSA () and C. albicans () i.e. MIC was 0.625 mg/ml and MBC/MFC was 1.25 mg/ml, in compared to gram-negative bacteria MDR-PA () and MDR-AB () i.e. MIC was 1.25 mg/ml and MBC was 2.5 mg/ml, respectively. Whereas, GL-CuO-1 showed least antibacterial activity against MRSA (), MDR-PA (), and MDR-AB () i.e. (MIC/MBC 5/10 mg/ml) (). Similarly, the NPs synthesized by pomegranate peels showed that PP-CuO-3 exhibits enhanced antibacterial activity against gram-positive MRSA bacteria i.e. the MIC and MBC values were 0.625 and 1.25 mg/ml, respectively (, ). Whereas, PP-CuO-2 showed the least antimicrobial activity against MDR-AB (MIC/MBC 5/10 mg/ml) and C. albicans (MIC/MBC 5/10 mg/ml) followed by MRSA and MDR-PA (MIC/MBC 3.5/7 mg/ml), respectively (). Penicillin and fluconazole were used as positive controls whereas plant extract was used as negative control. It was observed that multidrug-resistant A. baumannii and multidrug-resistant P. aeruginosa was resistant to penicillin. For E. coli, the MIC of penicillin was 64 µg/ml, while the MIC of fluconazole for C. albicans was also 64 µg/ml. Using the well diffusion method, (Citation33) reported noteworthy antibacterial action of CuO-NPs against human pathogenic bacteria, including E. coli, S. aureus, P. aeruginosa, and Proteus mirabilis. Similar results were also observed in the present study against human pathogenic bacteria. It was observed that green CuO NPs exhibited dose-dependent antibacterial activity. Our findings are consistent with other published work that also showed that the antibacterial activity of CuO-NPs increases with increasing concentration (Citation34,Citation35). In another study, it was reported that the CuO-NPs were effective against B. subtilis, S. aureus, E. coli, and S. typhi bacterial strains at concentration of 20 mg/ml (Citation36). However, in another study, it was found that CuO NPs synthesized by Abutilon indicum leaf extract were effective against S. aureus, Klebsiella and B. subtilis at 5 mg (Citation37). It is quite clear from that the synthesized NPs were more effective against gram-positive bacteria, MRSA, and fungi, C. albicans, than against gram-negative bacteria i.e. MDR-PA and MDR-AB. MIC and MBC values determined for all samples tested in this study are consistent with the latest research in literature (Citation38) that indicated MIC and MBC values of CuO-NPs in the range less than 5000 mg/ml. The explanation of these results could emerge from the capability of these particles to oxidize the insoluble metallic copper form; thus, the inhibition of microbial contact diminishes, and more NPs are needed to kill bacteria (Citation39) ( ).
Figure 9. MHA plates showing MBC (mg/ml) value for methicillin-resistant Staphylococcus aureus mixed with CuO nanoparticles synthesized by GL and PP extract.
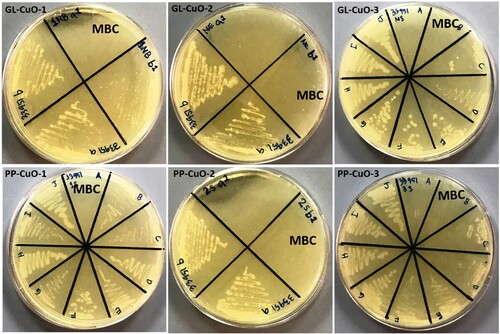
Figure 10. SDA plates showing MBC (mg/ml) value for C. albicans treated with CuO nanoparticles synthesized by GL and PP extract.
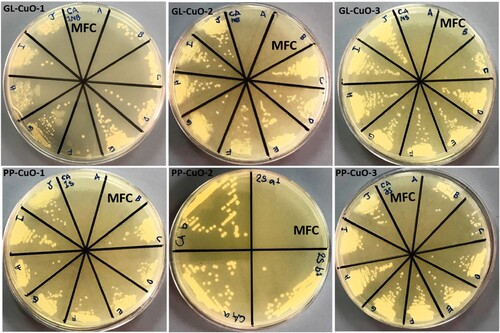
Figure 11. MHA plates showing MBC (mg/ml) value for Pseudomonas aeruginosa mixed with CuO nanoparticles synthesized by GL and PP extract.
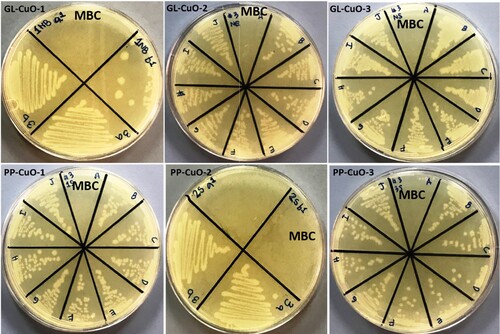
Figure 12. MHA plates showing MBC (mg/ml) value for Acinetobacter baumannii mixed with CuO nanoparticles synthesized by GL and PP extract.
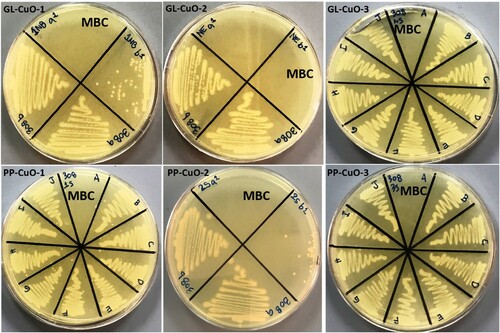
Table 3. MIC and MBC/MFC (mg/ml) values of tested nan bio-capped CuO samples.
3.3 Suggested mechanisms for antimicrobial activity of PP-CuO and GL-CuO series
The ability of bio-capped nanoparticles to penetrate bacteria’s outer membrane is usually the key factor for CuO lethality toward microbial cells. This step leads to the release of Cu+2 inside the cell, which damages the microbial cell (Citation40). Further investigations have also established that the penetration of nano-metal oxide into the cell membrane of gram-negative bacteria is more successful than in the gram-positive bacteria due to the thin outer layer of gram-negative bacteria consisting of liposaccharides. In contrast, the outer layer of gram-positive bacteria, which consists of many thick layers of peptidoglycan, can resist the penetration of nanometal oxide (Citation41). However, in this work, the result declared that bio-capped CuO-NPs show a higher lethal ability toward gram-positive bacteria compared to gram-negative bacteria, especially for those bio-capped CuO extracted by soaking in aqueous media, namely PP-CuO-3 and GL-CuO-3, which release more organic residue. That leads us to suggest a three-step mechanism to explain this ability. The first step includes the penetration of bio-capped CuO-NPs into the bacteria cell’s outer membrane. In this step, bio-capped CuO-NPs successfully penetrate the outer membrane of gram-positive bacteria more effectively than gram-negative bacteria. This is due to the possible negative repulsion generated between the negative charge of the liposaccharide layer covering gram-negative bacteria and the negative charge coverage of the bio-capped CuO-NPs from the capping agent (as judged from zeta potential and FT-IR results).
The second step assumes the release of Cu2+ ions from bio-capped CuO-NPs into the bacteria cell. The copper ions inactivate the catalytic enzyme processes or produce ROS species (Citation42). Since the ability of bio-capped CuO-NPs to penetrating the outer membrane of negative-gram bacteria was less than that of bio-capped CuO-NPs, we expected that the amount of Cu2+ ion released would be less effective in killing the gram-negative bacteria. The third step in this work is considered to be synchronized with the second step, where the capping agent, consisting of phenolic, carboxylic acids, and flavonoid compounds (as judged from FT-IR), produces ROS species when contacted with metal or metal oxide in physiological media (Citation43). The ROS species work to damage the bacteria’s cell by affecting various macromolecules inside the cell such as lipids, DNA and proteins (Citation31, Citation44).
Conclusion
This study is intended to investigate the impact of both extraction technique and extraction solvent on the effectiveness of the synthesized CuO-NPs against selected types of multi-drug resistant bacteria and fungi. The different techniques used in the synthesis of bio-capped CuO-NPs slightly affect the morphological and physicochemical properties; however, they ultimately influence their antimicrobial efficiency. Although bio-capped CuO-NPs prepared from both capping agents, GL and PP, demonstrated high antimicrobial potency against selected MDR strains, the results were influenced by the medium and technique applied for the extraction of the capping agent. Notably, aqueous extracts of pomegranate peel obtained via boiling or soaking demonstrated a notable enhancement of the antibacterial effect against gram-positive strains compared to the extract obtained by 1:1 hydroethanol extraction. However, the results confirmed that GL-CuO NPs obtained by soaking in either of pure water or 1:1 hydroethanol exhibited enhanced antimicrobial activity against gram-positive MRSA and C. albicans compared to the extract obtained by boiling in water. Furthermore, the antimicrobial mechanism of the bio-capped CuO-NPs against gram-positive and gram-negative bacteria includes three steps, as discussed. The first step involves the penetration of bio-capped CuO-NPs to the outer membrane of both types of bacteria examined, followed by two synchronized steps: the release of copper ions and the production of ROS species. The Cu + 2 and ROS work together to terminate the bacterial activity. However, more studies should be conducted in the future to investigate the detailed mechanism of the lethal effect of the bio-capped CuO-NPs toward the fungi cells. Finally, further research should be conducted using different solvents and media for extracting and synthesizing CuO–NPs and investigating its biological effect as an antimicrobial agent.
Supplemental Material
Download MS Word (2 MB)Disclosure statement
No potential conflict of interest was reported by the author(s).
The complete author details are provided below: Nesrine M.R. Mahmouda, Amal L.Al-Aotaibib,e, Sultan Akhtarc, Mohammad Azam Ansarid, Abeer Ramadana, Somia B. Ahmeda
aDepartment of Basic Sciences, Deanship of Preparatory Year and Supporting Studies, Imam Abdulrahman Bin Faisal University, P.O. Box 1982, Dammam 34212, Saudi Arabia. Email:[email protected], [email protected]
bDepartment of Physics, College of Science, Imam Abdulrahman Bin Faisal University, P.O. Box 1982, Dammam 31441, Saudi Arabia.
cDepartment of Biophysics, Institute for Research and Medical Consultations (IRMC), Imam Abdulrahman Bin Faisal University, P.O. Box 1982, Dammam 31441, Saudi Arabia.
dDepartment of Epidemic Disease Research, Institute for Research and Medical Consultations (IRMC), Imam Abdulrahman Bin Faisal University, P.O. Box 1982, Dammam 31441, Saudi Arabia. [email protected]
e Basic and Applied Scientific Research Center, Imam Abdulrahman Bin Faisal University, P.O. Box 1982, Dammam 31441, Saudi Arabia.
Correction Statement
This article has been corrected with minor changes. These changes do not impact the academic content of the article.
Additional information
Funding
References
- Ying, S.; Guan, Z.; Ofoegbu, P.C.; Clubb, P. Green Synthesis of Nanoparticles: Current Developments and Limitations. Environ. Technol. Innov. 2022, 26, 102336. doi:10.1016/j.eti.2022.102336.
- Al-fa Abu-kharma, A.M.; Awwad, M.H. Green Synthesis of Copper Oxide Nanoparticles Using Bougainvillea Leaves Aqueous Extract and Antibacterial Activity Evaluation. Chem Int 2021, 7 (3), 155–162.
- Mahmoud, N.M.R.; Elbadawy, H.A.; Refaat, H.M. An Efficient Green Protocol for Photo-Degradation of Bromophenol Blue dye. Desalin. Water. Treat. 2021, 229, 403–412. doi:10.5004/dwt.2021.27384.
- Ahmad, W.; Pandey, A.; Rajput, V.; Kumar, V.; Verma, M.; Kim, H. Plant Extract Mediated Cost-Effective tin Oxide Nanoparticles: A Review on Synthesis, Properties, and Potential Applications. Curr. Res. Green Sustain. Chem. 2021, 4, 100211. doi:10.1016/j.crgsc.2021.100211.
- SD, D.S.; Roshan, A.; Sharma Timilsina, S.; Sunita, S. A review on the medical plant Psidium guajava Linn. (Myrtaceae). J. Drug Deliv. Ther. 2013, 3, 162–168. doi:10.22270/jddt.v3i2.404.
- Qian, H.; Nihorimbere, V. Antioxidant Power of Phytochemicals from Psidium guajava. J. Zhejiang Univ. Sci. 2004, 5, 676–683. doi:10.1631/jzus.2004.0676.
- Gutiérrez, R.M.P.; Mitchell, S.; Solis, R.V. Psidium Guajava: A Review of its Traditional Uses, Phytochemistry and Pharmacology. J. Ethnopharmacol. 2008, 117 (1), 1–27. doi:10.1016/j.jep.2008.01.025.
- Seo, J.; Lee, S.; Elam, M.L.; Johnson, S.A.; Kang, J.; Arjmandi, B.H. Study to Find the Best Extraction Solvent for Use with Guava Leaves (Psidium guajava L.) for High Antioxidant Efficacy. Food Sci. Nutr. 2014, 2 (2), 174–180. doi:10.1002/fsn3.91.
- Sampath Kumar, N.S.; Sarbon, N.M., Rana, S.S., et al. Extraction of Bioactive Compounds from Psidium guajava Leaves and its Utilization in Preparation of Jellies. AMB Expr. 2021, 11 (1). doi:10.1186/s13568-021-01194-9.
- Sathiyavimal, S.; Vasantharaj, S., Veeramani, V., et al. Green Chemistry Route of Biosynthesized Copper Oxide Nanoparticles Using Psidium guajava Leaf Extract and Their Antibacterial Activity and Effective Removal of Industrial Dyes. J. Environ. Chem. Eng. 2021, 9 (2), 105033. doi:10.1016/j.jece.2021.105033.
- Eghbali, S.; Askari, S.F.; Avan, R.; Sahebkar, A. Therapeutic Effects of Punica granatum (Pomegranate): An Updated Review of Clinical Trials. J. Nutr. Metab. 2021, 5297162. doi:10.1155/2021/5297162.
- El-Hadary, A.E.; Taha, M. Pomegranate Peel Methanolic-Extract Improves the Shelf-Life of Edible-Oils Under Accelerated Oxidation Conditions. Food Sci. Nutr. 2020, 8 (4), 1798–1811. doi:10.1002/fsn3.1391.
- Siddiqui, V.U.; Ansari, A.; Chauhan, R.; Siddiqi, W.A. Green Synthesis of Copper Oxide (CuO) Nanoparticles by Punica granatum Peel Extract. Mater. Today Proc. 2020, 6, 751–755. doi:10.1016/j.matpr.2020.05.504.
- Mahmoud, N.; Mohamed, H.I.; Ahmed, S.B.; Akhtar, S. Efficient Biosynthesis of CuO Nanoparticles with Potential Cytotoxic Activity. Chem. Pap. 2020, 74, 2825–2835. doi:10.1007/s11696-020-01120-6.
- Saparbekova, A.A.; Kantureyeva, G.O.; Kudasova, D.E.; Konarbayeva, Z.K.; Latif, A.S. Potential of Phenolic Compounds from Pomegranate (Punica granatum L.) by-Product with Significant Antioxidant and Therapeutic Effects: A Narrative Review. Saudi J. Biol. Sci. 2023, 30 (2), 103553. doi:10.1016/j.sjbs.2022.103553.
- Biswas B, Rogers K, McLaughlin F, Daniels D, Yadav A. Antimicrobial Activities of Leaf Extracts of Guava (Psidium guajava L.) on Two Gram-Negative and Gram-Positive Bacteria. Int. J. Microbiol. 2013. doi:10.1155/2013/746165.
- Hamida, R.S.; Ali, M.A.; Goda, D.A.; Khalil, M.I.; Al-Zaban, M.I. Novel Biogenic Silver Nanoparticle-Induced Reactive Oxygen Species Inhibit the Biofilm Formation and Virulence Activities of Methicillin-Resistant Staphylococcus aureus (MRSA) Strain. Front. Bioeng. Biotechnol. 2020, 8, 1–14. doi:10.3389/fbioe.2020.00433.
- Koulenti Xu, M., et al. Novel Antibiotics for Multidrug-Resistant Gram-Positive Microorganisms. Microorganisms. 2019, 7 (8), 270. doi:10.3390/microorganisms7080270.
- Karaiskos, I.; Giamarellou, H. Multidrug-resistant and Extensively Drug-Resistant Gram-Negative Pathogens: Current and Emerging Therapeutic Approaches. Expert. Opin. Pharmacother. 2014, 15 (10), 1351–1370. doi:10.1517/14656566.2014.914172.
- Ansari, M.A.; Kalam, A., Al-Sehemi, A.G., et al. Counteraction of Biofilm Formation and Antimicrobial Potential of Terminalia Catappa Functionalized Silver Nanoparticles Against Candida Albicans and Multidrug-Resistant Gram-Negative and Gram-Positive Bacteria. Antibiotics 2021, 10 (6), 725. doi:10.3390/antibiotics10060725.
- Ansari, M. Sonochemical Synthesis of ZnCo2O4/Ag3PO4 Heterojunction Photocatalysts for the Degradation of Organic Pollutants and Pathogens: A Combined Experimental and Computational Study. New J. Chem. 2022, 46 (29), 14030–14042. doi:10.1039/D2NJ01352E.
- Begum, S.; Esakkiraja, A.; Asan, S. Green Synthesis, Characterization and Antibacterial Activity of Copper Oxide Nanoparticles Synthesized Using Catharanthus Roseus Leaf Extract. J. Appl. Sci. Comput. 2020, 5 (8), 21–27.
- Siddiqui, H.; Parra, M.R.; Qureshi, M.S.; Malik, M.M.; Haque, F.Z. Studies of Structural, Optical, and Electrical Properties Associated with Defects in Sodium-Doped Copper Oxide (CuO/Na) Nanostructures. J. Mater. Sci. 2018, 53 (12), 8826–8843. doi:10.1007/s10853-018-2179-6.
- Wang, R.; Ding, Y.; Liu, R.; Xiang, L.; Du, L. Pomegranate : Constituents, Bioactivities and Pharmacokinetics. Fruit, Veg Cereal Sci Biotechnol 2010, 4 (2), 77–87.
- Viuda-Martos, M.; Fernández-López, J.; Pérez-Álvarez, J. Pomegranate and its Many Functional Components as Related to Human Health: A Review. Compr. Rev. Food Sci. Food Saf. 2010, 9, 635–654. doi:10.1111/j.1541-4337.2010.00131.x.
- Bhardwaj, B.S.; Punj, D.; Raji, K.P.; Kailasnath, M.; Chandramohanakumar, N. Green Synthesis of Silver Nanoparticles Using Azadirachta. J. Environ. Sci. 2010, 325 (1), 2367.
- Ahmed, S.; Mohamed, H.; Al-Subaie, A.; Al-Ohali, A.; Mahmoud, N. Investigation of the Antimicrobial Activity and Hematological Pattern of Nano-Chitosan and its Nano-Copper Composite. Sci. Rep. 2021, 11. doi:10.1038/s41598-021-88907-z.
- Ahmed, S.B.; Mahmoud, N.M.R.; Manda, A.A.; Refaat, H.M. Study of the Optimization and Mechanism for the Remediation Process of Malachite Green Dye Via Hybrid-Based Magnetite-Date’s Stones: Study of the Optimization and Mechanism for the Remediation Process. Alexandria Eng. J. 2022, 61 (12), 9879–9889. doi:10.1016/j.aej.2022.02.065.
- Kolahalam, L.A.; Prasad, K.R.S.; Krishna, P.M.; Supraja, N.; Shanmugan, S. The Exploration of bio-Inspired Copper Oxide Nanoparticles: Synthesis, Characterization and in-Vitro Biological Investigations. Heliyon 2022, 8 (6), e09726. doi:10.1016/j.heliyon.2022.e09726.
- Alhalili, Z. Green Synthesis of Copper Oxide Nanoparticles CuO NPs from Eucalyptus Globoulus Leaf Extract: Adsorption and Design of Experiments. Arab. J. Chem. 2022, 15 (5), 103739. doi:10.1016/j.arabjc.2022.103739.
- Akintelu, S.A.; Folorunso, A.S.; Folorunso, F.A.; Oyebamiji, A.K. Green Synthesis of Copper Oxide Nanoparticles for Biomedical Application and Environmental Remediation. Heliyon 2020, 6 (7), e04508. doi:10.1016/j.heliyon.2020.e04508.
- Dashrath Bansod, S. Synthesis and Evaluation of Antimicrobial Potential of Copper Nanoparticle Against Agriculturally Important Phytopathogens Production of Biofertilizer amd Micronutrients. View Project. Int. J. Biol. Res. 2016, 1, 41–47. https://www.researchgate.net/publication/309703772.
- Altikatoglu, M.; Attar, A.; Erci, F.; Cristache, C.; Isildak, I. Green Synthesis of Copper Oxide Nanoparticles Using Ocimum Basilicum Extract and Their Antibacterial Activity. Fresenius Environ. Bull. 2017, 26, 7832–7837.
- Ssekatawa, K.; Byarugaba, D., Angwe, M., et al. Phyto-Mediated Copper Oxide Nanoparticles for Antibacterial, Antioxidant and Photocatalytic Performances. Front. Bioeng. Biotechnol. 2022, 10, 1–17. doi:10.3389/fbioe.2022.820218.
- Amin F, Fozia, Khattak B, et al. Green Synthesis of Copper Oxide Nanoparticles Using Aerva javanica Leaf Extract and Their Characterization and Investigation of In Vitro Antimicrobial Potential and Cytotoxic Activities. Evidence-Based Complement Altern. Med.. 2021:5589703. doi:10.1155/2021/5589703.
- Dulta K, Ağçeli G, Chauhan P, Jasrotia R, Chandan G, Chauhan P. Multifunctional CuO Nanoparticles with Enhanced Photocatalytic Dye Degradation and Antibacterial Activity. 2021. doi:10.21203/rs.3.rs-346220/v1.
- Ijaz, F.; Shahid, S.; Khan, S.; Ahmad, W.; Zaman, S. Green Synthesis of Copper Oxide Nanoparticles Using Abutilon indicum Leaf Extract: Antimicrobial, Antioxidant and Photocatalytic Dye Degradation Activitie. Trop. J. Pharm. Res. 2017, 16, 743–753. doi:10.4314/tjpr.v16i4.2.
- El-Sherbiny, G.M.; Kalaba, M.H., Sharaf, M.H., et al. Biogenic synthesis of CuO-NPs as nanotherapeutics approaches to overcome multidrug-resistant Staphylococcus aureus (MDRSA). Artif. Cells Nanomed. Biotechnol. 2022, 50 (1), 260–274. doi:10.1080/21691401.2022.2126492.
- Bagchi, B.; Dey, S., Bhandary, S., et al. Antimicrobial Efficacy and Biocompatibility Study of Copper Nanoparticle Adsorbed Mullite Aggregates. Mater. Sci. Eng. C 2012, 32 (7), 1897–1905. doi:10.1016/j.msec.2012.05.011.
- Singh, R.; Smitha, M.S.; Singh, S.P. The Role of Nanotechnology in Combating Multi-Drug Resistant Bacteria. J. Nanosci. Nanotechnol. 2014, 14 (7), 4745–4756. doi:10.1166/jnn.2014.9527.
- Padi, V.V.T.; Cernik, M. Green Synthesis of Copper Oxide Nanoparticles Using gum Karaya as a Biotemplate and Their Antibacterial Application. Int. J. Nanomed. 2013, 8, 889–898. doi:10.2147/IJN.S40599.
- Kessler, A.; Hedberg, J.; Blomberg, E.; Odnevall, I. Reactive Oxygen Species Formed by Metal and Metal Oxide Nanoparticles in Physiological Media—A Review of Reactions of Importance to Nanotoxicity and Proposal for Categorization. Nanomaterials 2022, 12 (11), 1922. doi:10.3390/nano12111922.
- Held, P. An Introduction to Reactive Oxygen Species Measurement of ROS in Cells. BioTek Instruments 2012, 1–14. http://www.biotek.com/resources/articles/reactive-oxygen-species.html.
- Azam, A.; Ahmed, A.S.; Oves, M.; Khan, M.S.; Habib, S.S.; Memic, A. Antimicrobial Activity of Metal Oxide Nanoparticles Against Gram-Positive and Gram-Negative Bacteria: A Comparative Study. Int. J. Nanomed. 2012, 7, 6003–6009. doi:10.2147/IJN.S35347.