ABSTRACT
As an important class of natural products, pyrazole alkaloids have a large number of applications in various aspects such as medicines and pesticides. Due to the special position of its amino group, 3-aminopyrazole is easy to form a hydrogen bond donor–acceptor-donor, and the hydrogen bond ‘zipper’ structure enhances the binding ability of such compounds to receptors. This work provides a new one-pot three-components reaction for the efficient construction of multi-substituted aminopyrazoles derivatives via iodine-mediated cyclization in the presence of ethanol. After that, the potential mechanism of the reaction was explored based on the control transformation experimental and density functional theory (DFT) calculations. In addition, all obtained multi-substituted aminopyrazole derivatives were fully investigated for their application as potential anti-cancer agents, and some of which have been proved to exhibit excellent anti-cancer activity against A875 and HepG2 cell lines, and the possible structure–activity relationships also has been discussed based on the screening results. The kinase assay and molecular docking results indicate that these compounds may be potential CDK1 inhibitors.
GRAPHICAL ABSTRACT
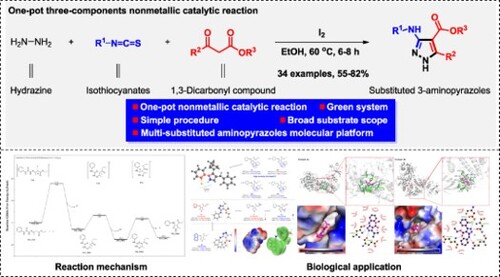
Introduction
Natural products, especially heterocyclic compounds, have been an important source of drug molecules and molecular libraries of potential drugs. By strengthening the research on natural product molecules, it is beneficial to discover new active molecules, improve the activity of known structures, and realize the chemical synthesis and industrialization of drug molecules (Citation1–8). Among many natural products-inspired molecules, pyrazoles are widely present in many drug molecules due to their excellent biological activities such as analgesic and anti-inflammatory (Citation9–15), antibacterial (Citation16–23), antitumor (Citation24–31) and Alzheimer’s disease treatment (Citation32), especially, some of them have been developed as commercially available drugs, such as Celecoxib and Razaxaban ().
Pyrazole alkaloids are also a class of natural products with excellent activity (Citation33–36), especially 3-aminopyrazole, due to the special position of its amino group, three spatially similar nitrogen are easy to form hydrogen bond donor–acceptor-donor with the acceptor. The hydrogen bond ‘zipper’ structure enhances the binding ability of this type of compound to the receptor, making it have excellent drug-making potential (Citation37–45), but the synthesis of this type of compound is relatively complicated ((A)). According to previous report, the synthesis of 3-aminopyrazole was achieved through adding a nitro or carboxyl group at the 3rd position of pyrazole, and then reducing it to an amino group (Citation46–51); or activating the third position of pyrazole with a halogen, and then useing an amine to replace it (Citation52–57); or reacting with hydrazine hydrate and a cyano group to obtain an unsubstituted amino group (Citation58–61). Some 3-aminopyrazoles can also be constructed from complex structures by ring opening and closing reactions (Citation62,Citation63). However, in addition to the general cumbersome steps, these methods also face problems such as low yields, difficult access to substrates, and harsh conditions, etc.
As we all know, isothiocyanates (R-N = C = S) are a class of important organic synthetic blocks, which can participate in a variety of organic reactions. They are used to construct various types of sulfur, nitrogen and oxygen compounds, especially heterocyclic compounds, and are widely used in the preparation of organic synthetic products such as pharmaceuticals, pesticides, and dyes, etc. As indicated in (B), phenyl isothiocyanate is widely used for the synthesis of different chain (Citation64–68) or heterocyclic structures (Citation69–85) because of its excellent reactivity. In addition, the multi-component reaction (MCR) is a popular strategy for highly efficient organic transformation to construct various heterocycles and their derivatives (Citation86–98), which has the advantages of mild reaction, high atom-efficiency and simple operation, and attract the increasing interesting in organic and medicinal chemistry.
So, as our continuing interest in the discovery of functional molecules based on novel heterocycle construction, herein we report an iodine-mediated cyclization strategy for the construction of multi-substituted 3-aminopyrazoles via a convenient three-components nonmetallic catalytic process ((C)). In this method, the easily available hydrazine hydrate, isothiocyanates and 1,3-dicarbonyl compounds were adopted as substrates in the presence of ethanol to construct diverse pyrazole derivatives. The potential biological applications of all synthesized multi-substituted pyrazole derivatives were fully investigated based on biological evaluation, kinase assay and molecular docking.
Results and discussion
Chemistry
First, the three-component reaction of hydrazine hydrate (1), phenyl isothiocyanate (2a), ethyl acetoacetate (3a) was chosen as the template reaction for the optimization of reaction conditions (). Several Lewis acids have been screened for this transformation, but limited target products could be obtained when CuBr2, CuCl2, Zn(OAc)2, Bi(OTf)3, or BF3·OEt2 was used as the catalysts (Entries 4–8), and the target product could not be obtained either without using a catalyst (Entry 27). Regardless of using weaker Lewis acids such as CuBr2, CuCl2, or using stronger Lewis acids such as Bi(OTf)3, BF3·OEt2, the desired product cannot be obtained, which indicates that the role of iodine is not only as a Lewis acid. Subsequently, the iodine or the combination of iodine and other Lewis acid can catalyze this reaction and obtain the target multi-substituted pyrazole molecules, which indicates that iodine did not act only as a Lewis acid. On the other hand, the addition of an oxidizing agent had little effects on the reaction (Entry 14), indicating that the reaction did not undergo a process of peroxidative ring formation. Further investigations revealed that the reaction was also affected by the catalyst amount and solvents. For different solvent used, there is little difference in yield between ethanol and 1,4-dioxane, and both solvents can be obtained with a yield of more than 70%, while the use of polar aprotic solvents is basically impossible (Entries 16–19). For the product, considering green chemistry and convenient reaction post-processing, ethanol was selected as the solvent to optimize the subsequent conditions. When ethanol was used as the solvent and an equivalent of iodine was added as the catalyst, the product could be obtained with a yield of 80% (Entry 3).
Table 1. Condition optimization for the three-component reaction.Table Footnotea
When discussing the effect of temperature on the reaction, the yield increases gradually with the increase of temperature, and the temperature rises to ethanol reflux has little effects on the reaction yield (). When the reaction temperature is higher than 60 degrees, the yield is basically the same. From the relationship between time and product content, it can be seen that the product gradually increases linearly with the change of time. In addition, experiments on the reaction intermediates show that the substrates are all converted into intermediates in a short time, so the catalytic cyclization of iodine is the rate-determining step. These investigations indicated that the reaction was also affected by the temperature and reaction time.
Table 2. The optimization on reaction temperature and time for three-component reaction.
For the selection of the template reaction, experiments have proved that I2 (1eq.) is the catalyst with the best catalytic effect, and the use of other Lewis acids or oxidants has not yielded better results. Although the effect of using 1,4-dioxane as a solvent is similar to that of ethanol, from the perspective of environmental friendliness and convenient post-processing, ethanol is selected as the solvent for the reaction. In terms of temperature and reaction time, 60°C was used to react for three hours. Considering that the boiling point of ethanol is 78.3°C, using 60°C can give consideration to both reaction time and yield.
With a set of optimized conditions, we have also explored the expansion of substrates and tried a variety of different substrate combinations. Generally, functional groups with different electrical properties and different structures can be transferred stably and efficiently to the desired products. As evidenced in Scheme 1, for the substituents on the benzene of phenyl isothiocyanate, electron withdrawing groups such as nitro, halogen (4i, 4j), the electrons will be pushed out which leads to increased reactivity of these isothiocyanates, and as well as the yield increased. The substituents with other groups will slightly reduce the yield. Generally, the influence of the substituents on the benzene ring is small, and the substances bearing functional groups with different positions and different electrical properties can also be transferred to the corresponding products in higher yields. In addition to the benzene ring, both pyridine isothiocyanate and naphthalene isothiocyanate (4b, 4 h) were also used to construct this class of substituted pyrazoles with rather high yields 64 and 63%, respectively.
For 1,3-dicarbonyl compounds, it is necessary to have oxygen or nitrogen at one end to achieve selective addition. Substitution of R2 with aliphatic carbon, pyridine or benzene ring (4s, 4x, 4y), significantly improve the product yield, which might indicate that electron-rich groups increase the selectivity for carbonyl addition. Products with ester groups and amides at R3 have high yields (Scheme 2), and these two active groups can be introduced stably, which further improves the derivatization potential of the products. After hydrolysis, other pharmacophore groups can be linked to improve activity, while oxygen or substituents on nitrogen did not have significant effects on yields.
In drug design, it is sometimes necessary to introduce multiple pharmacophores at the same time to improve the activity of the compounds. So, the application potential of this aspect has also been studied, by using substituted phenyl isothiocyanate containing methoxy, fluorine and trifluoromethyl groups, and 1,3-dicarbonyl compounds with 2-chlorophenyl, phenyl and 2,6-dichloro-5-fluoropyridin-3-yl units as substrates for cross-pairing reaction (Scheme 3). It showed that the yield of the product is maintained at a high level when groups at multiple positions are changed at the same time which indicated the excellent application potential of this reaction.
Finally, to illustrate the potential application of our developed method and diversify the structures of multi-substituted pyrazoles, we also performed a gram-scale reaction using the optimized conditions and observed the product 4a could be obtained with good isolated yield when 15 mmol of raw materials were input. In addition, the compound 4a can also be easily transformed to the corresponding substituted pyrazole carboxylic acid 5a, pyrazolyl acylhydrazide 5b, N-substituted pyrazoles 5c, and substituted pyrazolmethanol 5d via saponification, substitution, or reduction reaction, respectively (Scheme 4). These building blocks can be used as important units to construct diverse potential drug molecules.
Spectroscopy
All obtained pyrazole derivatives were elucidated based on chemical analyses including 1H NMR, 13C NMR, ESI-MS, and X-Ray diffraction analyses. For the 1H NMR spectra of all aminopyrazole derivatives 4a-z, the obvious signals at 2.43–2.28 ppm were attributed to the methyl group attached to the pyrazole ring as shown in the molecular structure, the 1H NMR spectrum indicated a unique signal for the flexible bond CH2CH3 unit, with a quartet signal at about 4.35–4.22 ppm and a triplet signal at about 1.40–1.30 ppm, respectively. Both the NH protons on the pyrazole ring and the NH protons next to the pyrazole ring appear in the range of 8.41–8.00 ppm. The remaining signal peaks at low fields are attributed to the proton peaks of the pyrazole ring or other aromatic rings. All the characteristic peaks observed within the 1H NMR spectra for title compounds are given in Materials and methods. In addition, the representative single crystal diffraction analysis of target compound 4a is shown in .
Reaction pathway
In order to explore the possible reaction pathway, we designed a control experiment by reacting phenyl isothiocyanate (2a), ethyl acetoacetate (3a) and hydrazine hydrate (1) directly in ethanol without adding any catalyst (Scheme 5). Compound 6 can be conveniently isolated from reaction solution. In addition, compound 6 can be transformed in ethanol after the addition of iodine to give the final product 4a, which demonstrate that compound 6 may be an important reaction intermediate. During the course of this transformation, the UPLC-MS data indicate the obvious MS (ESI) m/z 280.33 = [M + H]+ of compound 6, which is consistent with the molecular weight of intermediate 6 (M = 279.10). In addition, the 1H NMR analyses present the special peaks for this molecule, and two secondary amine active hydrogens can be observed the singlets appear at 10.56 and 9.93 ppm, the multiplets of the benzene ring appear at 7.59–7.71 ppm, and the methyl, methylene and ethyl groups from ethyl acetoacetate appear in the high field area (see Supporting Information).
Based on the aforementioned investigation, a plausible mechanism for the synthesis of multi-substituted pyrazoles was proposed and depicted in Scheme 6. The first step of this reaction is that hydrazine attacks isothiocyanate to form thiosemicarbazide 7, and then condenses with 1,3-dicarbonyl compound to form intermediate 6. The nucleophilic reagent attacks the iodine-substituted methyl active center to form a six-membered ring intermediate 9, iodine leaves in the form of ions and removes a hydrogen ion, and then intramolecular electron transfer triggers sulfur elimination to obtain the final product pyrazoles 4.
Furthermore, the density functional theory (DFT) calculations has been performed for explore the reaction mechanism. The Gibbs free energy distribution is shown in . The reaction starts from intermediate 6, of which SN2 reaction is first happened to replace by iodine, and crossover of 71.3 kcal/mol energy barrier activates the α-position of the carbonyl group. Then the nucleophilic sulfur attacks the electrophilic carbonyl α-position due to iodine’s electron-absorbing electrons, and gains 25.7 kcal/mol to form an intermediate state TS2, a part of iodine and hydrogen leaves in the form of ions, energy drops by 18.0 kcal/mol, the system tends to be stable, and finally crosses the third energy barrier with an energy of 37.7 kcal/mol, unstable sulfur leaves as elemental sulfur, and intramolecular electron transfer forms 3-aminopyrazole structure.
Biological evaluation
In vitro bioassay
In order to examine the practicability of this aminopyrazoles derivatives, all of the obtained multi-substituted pyrazole derivatives were screened for their potential in vitro anti-cancer activities against HepG2 (Human hepatocellular liver carcinoma cells), A875 (Human melanoma cells) cell lines using the standard MTT assay, meanwhile, the normal cell line HL-7702 (Human normal liver cells) is also used to verify the possible safety of compounds, and the preliminary bioassay results were described in and . Generally, as shown in , the activities of compounds 4ea, 4ec, 4 h, 4s and 4z were better than that of the positive control 5-FU. Among them, 4ea only showed better activity than the positive control on HepG-2 cells, and the other four compounds indicated better activity on both cancer cells than that of 5-FU. Among these four compounds, compound 4s is less toxic to normal liver cells, this proves that compound 4s has higher safety compared with other compounds and has higher potential for drug development.
Figure 5. Survival rates of HL-7702, A875 and HepG-2 treated with different concentration of 4ea, 4ec, 4h, 4s and 4z.
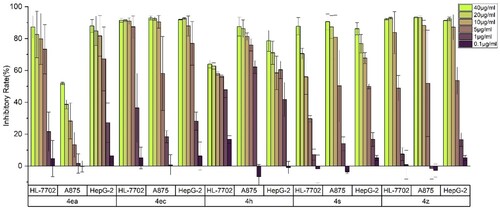
Table 3. In vitro cytotoxic activities of target compounds against tested cell lines.
Whether it can still exhibit tumor-inhibitory ability at low concentrations is an important criterion for judging a compound’s drug potential. By comparing several compounds with the best activity in previous biological assays, the relationship between concentration and inhibition rate was also analyzed (). Overall, the inhibitory rate of all five compounds on HepG-2 cells at low concentrations are higher than that of A875 cells, which indicates that this type of aminopyrazoles has better inhibitory activity on HepG-2 cells. It also can be seen that the high activity is maintained for 4 h at different concentrations, and it can still maintain a similar inhibition rate at a lower concentration of 1 μg/ml, and its effect on cancer cells is always higher than on normal cells. Those other three compounds 4ea, 4ec and 4z having similar structures, with chlorine in the same position, show similar properties in activity. While the concentration decreases to 5 μg/ml, all three compounds maintain high inhibitory activities, especially against HepG-2 cells, but the activities decrease correspondingly with further decreasing concentrations. The activity of 4s is affected by the concentration of the compound. However, the decreasing trend of toxicity of 4s at decreased concentrations on normal cells is significantly higher than that on cancer cells, and it also has certain potential for further research.
Structure–activity relationships
Generally, compounds 4ea, 4ec, 4ga and 4z exhibited higher activity on HepG-2, all of which were better than that of the positive control 5-FU. What they have in common is that there is the chlorine in the ortho-position. From the molecular point of view, chlorine, as a strong charge-absorbing group, might changes the activity of the molecule, and form a six-membered ring with the hydrogen at the first position of pyrazole through a hydrogen bond in space (). On one hand, it changes the surface electrostatic potential of the molecule, on the other hand, the strong charge-absorbing chlorine can enhance the electrophilicity of the nitrogen on the central pharmacophore, making it easier to form hydrogen bonds and bind to the target. From the perspective of drug administration, the introduction of halogen can increase the water solubility of the molecule, increase the permeability to cells, and render a higher activity. In addition to HepG-2 cells, 4ec and 4z also exhibit excellent activity against A875.
For compound 4s, compared with 4a without introduction of pharmacophore, the isopropyl group linked to the ester bond at the fourth position did not improve the activity significantly, but the isopropyl group directly linked to the fifth position instead of a methyl group increased the activity for about five times. It might be attributed to the hydrophobicity of the alkyl group. The alkyl group is prone to desolvating in cells, and this desolvation can usually enable the molecules better embedded in the hydrophobic pocket of the target, so as to realize the close combination with the target and improve the molecular activity.
Another one with better activity is compound 4 h. When the group linked to the amino group at the third position is benzene or pyridine, the phenyl or pyridyl group in the molecule is linked to the pyrazole through the nitrogen with a p orbital, which is easy to connect with the group at the fourth position. The ester group forms a delocalized Π bond, which tends to be a planar structure, but when the third position is introduced with naphthalene, it tends to form a Π-Π stacking interaction. It can be clearly seen that the surface electrostatic potential of 4 h produces a large electronegativity planes (), Π bonds also generate mutual folding, which leads the formation of Π-Π stacking interaction between 4 h and the target protein, strengthens the binding ability to the target, and then improves the activity.
Kinase inhibitory assay and molecular docking
Based on the aforementioned in vitro results and SARs analyses, in order to further investigate the potential target of highly active molecules, the kinase inhibitory profile of potential compounds 4s and 4z were screened at the concentration of 10 µM over a panel of sixteen kinases, and the kinase inhibitory activities are listed in . It was found that compound 4s exerted certain inhibitions on the tested CDK1, FAK and ALK protein kinases at 10 µM, and compound 4z also indicated some inhibitions on the tested CDK1, ALK, MET, and FAK protein kinases at 10 µM. Comprehensive analysis indicated that these two compounds had relatively good inhibitory activity against CDK1, and this result will provide some explanations or reference for their good anti-cancer activities.
Table 4. Kinase inhibitory activity.
As we know, cyclin-dependent kinases (CDK) is a set of Ser/Thr kinase systems related to cell cycle progression. By inhibiting cyclin-dependent kinases, it can target and regulate the tumor cell cycle and then trigger tumor cell apoptosis (Citation44,Citation45). Pevarello and coworkers reported that 3-aminopyrazole could act as an inhibitor of CDK, by entering the lipophilic pocket composed of residue Val18, Ala31, Val64, and Phe80, and form hydrogen bonds with the hinge region (Glu81-Leu83) of the kinase ATP pocket. This structure is completely consistent or highly conserved in the CDK protein family, and may be highly potential CDK inhibitors (Citation44,Citation45). So, in order to get a deeper explanation in structure and activity relationships for these multi-substituted pyrazole derivatives, two compounds with good potentials (4s and 4z) were docked with protein CDK1 (PDB: 4Y72) respectively ().
As shown in the , the docking results of compound 4s confirmed the previous conjecture, which can well enter the aforementioned lipophilic pocket, and the nitrogen on the pyrazole ring can be combined with residue Glu81 and Leu83 and form the corresponding hydrogen bonds, a donor–acceptor-donor ‘zipper’ structure of hydrogen bonds is formed, which enhances the binding ability between 4s and CDK1 protein. The bond lengths are 2.6, 3.0 and 3.3 Å respectively. In addition to hydrogen bonds, 4s also binds to lipophilic pocket Ile10, Val18, Ala31, Val64, Phe80, Phe82, Met85, Asp86, and Leu135 interact with each other. On the contrary, the docking results of 4z showed different results, and compound 4z interacted with multiple groups of residues, forming hydrogen bonds with Glu12, Gln132, Asn133 and Asp146 respectively, and the benzene ring at the fifth position of pyrazole can enter deeper into the lipophilic pocket, interacting with residues Ile10, Gly11, Tyr15, Val18, Asp86 and Ala145, the different docking results may explain why 4ea, 4ec, 4ga and 4z, which have similar structures, have the same performance in activity. Overall, the docking results prove that these compounds have a strong binding ability to CDK1 protein, and CDK1 may be the potential target for these compounds to produce anticancer activity.
Conclusion
A novel iodine-mediated cyclization reaction was developed by using one-pot three-components reaction to construct diverse multi-substituted aminopyrazoles molecular platform. The established reactions can also be used to construct several important synthetic blocks for drug discovery. The newly prepared pyrazole derivatives have been bio-evaluated as highly potential anticancer agents against A875, HepG2 cell lines. The kinase assay and molecular docking analysis indicate that the corresponding potential molecules 4s and 4z have a strong binding ability to CDK1 protein, which may be developed as potential CDK inhibitors for discovery of novel anti-cancer agents.
Materials and methods
Instrumentation and chemicals
Unless otherwise noted, all reagents were purchased from commercial suppliers and used without purification. 1H NMR, 13C NMR and 19F NMR spectra were recorded on a Bruker Avance III 600 MHz FT-NMR spectrometer using CDCl3, DMSO-d6 or CD3OD as the solvent and tetramethylsilane (TMS) as the internal standard. Mass spectra were performed on a WATERS ACQUITY UPLC® H-CLASS PDA (Waters®) instrument. Thin-layer chromatography (TLC) was carried out on precoated silica gel plates GF254 (Qindao Haiyang Chemical, China), and spots were visualized under ultraviolet light. All starting materials and reagents commercially available were used without further purification, unless otherwise specified.
General synthetic procedure for the target compounds 4a-z
Substituted phenyl isothiocyanate (0.5 mmol), 1,3-dicarbonyl compound (0.5 mmol), hydrazine hydrate (0.5 mmol), I2 (0.5 mmol) and ethanol (5 mL) were added in a 25 mL single-neck flask, and stirred at 60°C for several hours. After the completion of reaction, the mixtures were concentrated and purified by silica gel column chromatography (petroleum ether/ethyl acetate) to give the target compounds. Their physico-chemical properties and the spectra data are indicated in Supporting information.
Density functional theory analyses
The calculation was performed use the Gaussian 16.0W software package, and the structures were displayed by GaussView 6.0 (Citation99).
Basically, all the calculations were based on the density functional theory (DFT) [DFT: <Quantum Chemistry > Levine, Ira N. 7 edition, 2014.P 621]. In the self-consistent field theory (SCRF), the Solvation Model based on Density (SMD) was used to consider the solvation effect of the molecules in the ethanol solution. The calculation was set in the conditions with temperature at 298.15 K, and the pressure at 1 atm, and all species of chemicals in the solution at 1 M. Under the above conditions, the geometry optimization, frequency and thermochemical analysis, potential energy surface scan, intrinsic reaction coordinate analysis, and single point energy calculate were carried out.
Among them, the geometry optimization and frequency analysis (OPT FREQ), transition state scan (TS), and intrinsic reaction coordinate (IRC) analysis were all described the C, H, O, N, S elements with the 6-31G (d) basis set at the level of Minnesota Functional Module 6.0 with double the amount of nonlocal exchange with Grimme's D3 Zero correction(M06-2X-D3), and single point energy (SP) calculate described C, H, O, N, S elements with 6-311 + G (d, p) basis set. The SDD pseudopotential basis set (G. Igel Mann, H. Stoll, H. Preuss, Mol. Phys. 65, 1321 (1988).) for I element in the all calculations, and the mixed basis set is used for special description.
(M06-2X: doi:10.1007/s00214-007-0310-x)
(SMD: doi:10.1021/jp810292n)
(SCRF: doi:10.1016/0301-0104(81)85090-2)
(D3 Zero: doi:10.1063/1.3382344)
(6-31G(d): doi:10.1002/jcc.1058)
(6-311 + G(d,p): doi:10.1063/1.438955)
Frequency analysis were based on the geometry optimized structures and carried out to verify the stationary point as minima. The transition state was found by relaxed scan. The maximum energy points of the scanned system were extracted as the optimization of the transition state, and the TS method was used to search for the transition state. All the above calculations ensured that the intermediates and products have no imaginary frequencies, and the transition states have only one imaginary frequency. By the observed vibrate directions of the imaginary frequency, it was verified that the directions of the transition state vibrations connected the reactant and the product.
All the transition states are verified by IRC analysis based on local quadratic approximation. The step-size of the calculation was 5 and the max points were 40. The obtained transition states and the front and rear end systems scanned by IRC were optimized, and the single point energy calculations were carried out to analyze the change of free energy in the reaction process.
The following thermochemical parameters, such as Gibbis free energy (G), Zero Point Energy (ZPE), Molecule Total Energy (MTE), internal energy (U), enthalpy (H) and entropy (S) were calculated by Shermo 2.3.4 (Citation100).
In vitro cytotoxicity assays
The in vitro cytotoxicities of these pyrazole derivatives against normal human liver cells (HL-7702), human melanoma (A875), and Human hepatoellular carcinomas (HepG-2) cell lines were evaluated using the MTT assay. All data were analyzed with SPSS software, and the 50% inhibitory concentrations (IC50) of each compound for the different cell lines were determined. All assays were performed in triplicate in three independent experiments.
Kinase inhibitory assay
The kinase inhibitory profile of compounds 4s and 4z were screened at the concentration of 10 µM over a panel of sixteen kinases, and each assay was repeated twice. All the inhibitory assays were carried out through kinase profiling services provided by HY Biotech (Chinese), in which ADP-GLO kinase assays were used.
Molecular docking
The protein preparation process of flexible docking mainly includes fixing the exact residues, removing irrelevant water molecules, adding hydrogen atoms and adding charges, etc. The crystal structure of human CDK1/CyclinB1/CKS2 complexed with the inhibitor (PDB: 4Y72, https://www.rcsb.org/structure/4Y72) was used to bind to 4s and 4z in the docking studies.
After the solvent and ligands were removed, the macromolecules and the pyrazole derivatives were submitted to AutoDock Vina 1.2.0 to run the docking program using Scripts (Citation101). Two-dimensional molecular-protein interaction diagram was drawn using LigPlus + 2.2 software (Citation102), and the three-dimensional picture was drawn using Pymol 2.5 software (Citation103).
Multiwfn was used to calculate the surface electrostatic potential and stacking Π bond related images (Citation104,Citation105), while PMV was used to output related images (Citation106). The packing diagrams were drawn using VESTA (Citation107).
Supplemental Material
Download MS Word (5.3 MB)Acknowledgements
This work was financially supported by the Key R&D Project of Hubei Province (2023BBB063, 2021BBA081) and Natural Science Foundation of Hubei Province (2022CFB244), and the authors also gratefully acknowledge the partial support from Hubei Agricultural Science Innovation Center (2021-620-000-001-027).
Disclosure statement
No potential conflict of interest was reported by the author(s).
Additional information
Funding
References
- Yang, Y.; Liu, Q.; Shi, X.; Zheng, Q.; Chen, L.; Sun, Y. Advances in Plant-Derived Natural Products for Antitumor Immunotherapy. Arch. Pharm. Res. 2021, 44 (11), 987–1011.
- Greco, G.; Catanzaro, E.; Fimognari, C. Natural Products as Inducers of Non-Canonical Cell Death: A Weapon Against Cancer. Cancers (Basel) 2021, 13 (2), 1–64.
- Fang, Y.; Li, H.; Ji, B.; Cheng, K.; Wu, B.; Li, Z.; Zheng, C.; Hua, H.; Li, D. Renieramycin-type Alkaloids from Marine-Derived Organisms: Synthetic Chemistry, Biological Activity and Structural Modification. Eur. J. Med. Chem. 2021, 210, 113092.
- Bhutani, P.; Joshi, G.; Raja, N.; Bachhav, N.; Rajanna, P.K.; Bhutani, H.; Paul, A.T.; Kumar, R. U.S. FDA Approved Drugs from 2015-June 2020: A Perspective. J. Med. Chem. 2021, 64 (5), 2339–2381.
- Davison, E.K.; Sperry, J. Natural Products with Heteroatom-Rich Ring Systems. J. Nat. Prod. 2017, 80 (11), 3060–3079.
- Newman, D.J.; Cragg, G.M. Natural Products as Sources of New Drugs Over the 30 Years from 1981 to 2010. J. Nat. Prod. 2012, 75 (3), 311–35.
- Newman, D.J.; Cragg, G.M. Natural Products as Sources of New Drugs Over the Nearly Four Decades from 01/1981 to 09/2019. J. Nat. Prod. 2020, 83 (3), 770–803.
- Bailly, C. Anticancer Properties and Mechanism of Action of the Quassinoid Ailanthone. Phytother. Res. 2020, 34 (9), 2203–2213.
- Abd El-Karim, S.S.; Mohamed, H.S.; Abdelhameed, M.F.; El-Galil, E.A.A.; Almehizia, A.A.; Nossier, E.S. Design, Synthesis and Molecular Docking of new Pyrazole-Thiazolidinones as Potent Anti-Inflammatory and Analgesic Agents with TNF-α Inhibitory Activity. Bioorg. Chem. 2021, 111, 104827.
- Fadaly, W.A.A.; Elshaier, Y.; Hassanein, E.H.M.; Abdellatif, K.R.A. New 1,2,4-triazole/pyrazole hybrids linked to oxime moiety as nitric oxide donor celecoxib analogs: Synthesis, cyclooxygenase inhibition anti-inflammatory, ulcerogenicity, anti-proliferative activities, apoptosis, molecular modeling and nitric oxide release studies. Bioorg. Chem. 2020, 98, 103752.
- Kumar, D.; Kumar, R.R.; Pathania, S.; Singh, P.K.; Kalra, S.; Kumar, B. Investigation of Indole Functionalized Pyrazoles and Oxadiazoles as Anti-Inflammatory Agents: Synthesis, in-Vivo, in-Vitro and in-Silico Analysis. Bioorg. Chem. 2021, 114, 105068.
- Labib, M.B.; Fayez, A.M.; El-Nahass, E.S.; Awadallah, M.; Halim, P.A. Novel Tetrazole-Based Selective COX-2 Inhibitors: Design, Synthesis, Anti-Inflammatory Activity, Evaluation of PGE2, TNF-α, IL-6 and Histopathological Study. Bioorg. Chem. 2020, 104, 104308.
- Gedawy, E.M.; Kassab, A.E.; El Kerdawy, A.M. Design, Synthesis and Biological Evaluation of Novel Pyrazole Sulfonamide Derivatives as Dual COX-2/5-LOX Inhibitors. Eur. J. Med. Chem. 2020, 189, 112066.
- Sahoo, J.; Sahoo, C.R.; Nandini Sarangi, P.K.; Prusty, S.K.; Padhy, R.N.; Paidesetty, S.K. Molecules with Versatile Biological Activities Bearing Antipyrinyl Nucleus as Pharmacophore. Eur. J. Med. Chem. 2020, 186, 111911.
- Turones, L.C.; Martins, A.N.; Moreira, L.; Fajemiroye, J.O.; Costa, E.A. Development of Pyrazole Derivatives in the Management of Inflammation. Fundam. Clin. Pharmacol. 2021, 35 (2), 217–234.
- Marinescu, M. Synthesis of Antimicrobial Benzimidazole-Pyrazole Compounds and Their Biological Activities. Antibiotics (Basel) 2021, 10 (8), 1002.
- Dorababu, A. Recent Update on Antibacterial and Antifungal Activity of Quinoline Scaffolds. Arch. Pharm. (Weinheim) 2021, 354 (3), e2000232.
- Ibrahim, S.A.; Fayed, E.A.; Rizk, H.F.; Desouky, S.E.; Ragab, A. Hydrazonoyl bromide precursors as DHFR inhibitors for the synthesis of bis-thiazolyl pyrazole derivatives; antimicrobial activities, antibiofilm, and drug combination studies against MRSA. Bioorg. Chem. 2021, 116, 105339.
- Liu, H.; Chu, Z.W.; Xia, D.G.; Cao, H.Q.; Lv, X.H. Discovery of Novel Multi-Substituted Benzo-Indole Pyrazole Schiff Base Derivatives with Antibacterial Activity Targeting DNA Gyrase. Bioorg. Chem. 2020, 99, 103807.
- Mekky, A.E.M.; Sanad, S.M.H. Novel bis(Pyrazole-Benzofuran) Hybrids Possessing Piperazine Linker: Synthesis of Potent Bacterial Biofilm and MurB Inhibitors. Bioorg. Chem. 2020, 102, 104094.
- Verma, R.; Verma, S.K.; Rakesh, K.P.; Girish, Y.R.; Ashrafizadeh, M.; Sharath Kumar, K.S.; Rangappa, K.S. Pyrazole-based Analogs as Potential Antibacterial Agents Against Methicillin-Resistance Staphylococcus Aureus (MRSA) and its SAR Elucidation. Eur. J. Med. Chem. 2021, 212, 113134.
- Xu, Z.; Gao, C.; Ren, Q.C.; Song, X.F.; Feng, L.S.; Lv, Z.S. Recent Advances of Pyrazole-Containing Derivatives as Anti-Tubercular Agents. Eur. J. Med. Chem. 2017, 139, 429–440.
- Hamed, A.A.; Abdelhamid, I.A.; Saad, G.R.; Elkady, N.A.; Elsabee, M.Z. Synthesis, Characterization and Antimicrobial Activity of a Novel Chitosan Schiff Bases Based on Heterocyclic Moieties. Int. J. Biol. Macromol. 2020, 153, 492–501.
- Beheshti, A.; Bahrani-Pour, M.; Kolahi, M.; Shakerzadeh, E.; Motamedi, H.; Mayer, P. Synthesis, Structural Characterization, and Density Functional Theory Calculations of the two new Zn (II) Complexes as Antibacterial and Anticancer Agents with a Neutral Flexible Tetradentate Pyrazole-Based Ligand. Appl. Organometallic Chem. 2021, 35 (5), e6173.
- Gaber, A.A.; El-Morsy, A.M.; Sherbiny, F.F.; Bayoumi, A.H.; El-Gamal, K.M.; El-Adl, K.; Al-Karmalawy, A.A.; Ezz Eldin, R.R.; Saleh, M.A.; Abulkhair, H.S. Pharmacophore-linked pyrazolo[3,4-d]pyrimidines as EGFR-TK Inhibitors: Synthesis, Anticancer Evaluation, Pharmacokinetics, and in silico Mechanistic Studies. Arch. Pharm. (Weinheim) 2021, 31, e2100258.
- Bennani, F.E.; Doudach, L.; Cherrah, Y.; Ramli, Y.; Karrouchi, K.; Ansar, M.; Faouzi, M.E.A. Overview of Recent Developments of Pyrazole Derivatives as an Anticancer Agent in Different Cell Line. Bioorg. Chem. 2020, 97, 103470.
- Othman, I.M.M.; Alamshany, Z.M.; Tashkandi, N.Y.; Gad-Elkareem, M.A.M.; Anwar, M.M.; Nossier, E.S. New Pyrimidine and Pyrazole-Based Compounds as Potential EGFR Inhibitors: Synthesis, Anticancer, Antimicrobial Evaluation and Computational Studies. Bioorg. Chem. 2021, 114, 105078.
- Wang, G.; Liu, W.; Peng, Z.; Huang, Y.; Gong, Z.; Li, Y. Design, Synthesis, Molecular Modeling, and Biological Evaluation of Pyrazole-Naphthalene Derivatives as Potential Anticancer Agents on MCF-7 Breast Cancer Cells by Inhibiting Tubulin Polymerization. Bioorg. Chem. 2020, 103, 104141.
- Hu, C.H.; Neissel Valente, M.W.; Halpern, O.S.; Jusuf, S.; Khan, J.A.; Locke, G.A.; Duke, G.J.; Liu, X.; Duclos, F.J.; Wexler, R.R.; Kick, E.K.; Smallheer, J.M. Small Molecule and Macrocyclic Pyrazole Derived Inhibitors of Myeloperoxidase (MPO). Bioorg. Med. Chem. Lett. 2021, 42, 128010.
- Haribabu, J.; Balachandran, C.; Tamizh, M.M.; Arun, Y.; Bhuvanesh, N.S.P.; Aoki, S.; Karvembu, R. Unprecedented Formation of Palladium(II)-Pyrazole Based Thiourea from Chromone Thiosemicarbazone and [PdCl2(PPh3)2]: Interaction with Biomolecules and Apoptosis Through Mitochondrial Signaling Pathway. J. Inorg. Biochem. 2020, 205, 110988.
- Singh, N.K.; Kumbhar, A.A.; Pokharel, Y.R.; Yadav, P.N. Anticancer Potency of Copper(II) Complexes of Thiosemicarbazones. J. Inorg. Biochem. 2020, 210, 111134.
- Li, X.; Yu, Y.; Tu, Z. Pyrazole Scaffold Synthesis, Functionalization, and Applications in Alzheimer's Disease and Parkinson's Disease Treatment (2011–2020). Molecules 2021, 26, 1202.
- Blair, L.M.; Sperry, J. Natural Products Containing a Nitrogen-Nitrogen Bond. J. Nat. Prod. 2013, 76 (4), 794–812.
- Kumar, V.; Kaur, K.; Gupta, G.K.; Sharma, A.K. Pyrazole Containing Natural Products: Synthetic Preview and Biological Significance. Eur. J. Med. Chem. 2013, 69, 735–53.
- Santos, N.E.; Carreira, A.R.F.; Silva, V.L.M.; Braga, S.S. Natural and Biomimetic Antitumor Pyrazoles, a Perspective. Molecules 2020, 25 (6), 1364.
- Wang, S.; Zhang, B.; Chen, J.; Zheng, Y.; Feng, N.; Ma, A.; Xu, X.; Abdullah, M.A. Recent Advances in Ni-Catalyzed Allylic Substitution Reactions. Chin. J. Org. Chem. 2019, 39 (1), 15–27.
- Michon, V.; du Penhoat, C.H.; Tombret, F.; Gillardin, J.M.; Lepage, F.; Berthon, L. Preparation, Structural Analysis and Anticonvulsant Activity of 3- and 5-Aminopyrazole N-Benzoyl Derivatives. Eur. J. Med. Chem. 1995, 30 (2), 147–155.
- Hameed, P.S.; Manjrekar, P.; Chinnapattu, M.; Humnabadkar, V.; Shanbhag, G.; Kedari, C.; Mudugal, N.V.; Ambady, A.; de Jonge, B.L.; Sadler, C.; Paul, B.; Sriram, S.; Kaur, P.; Guptha, S.; Raichurkar, A.; Fleming, P.; Eyermann, C.J.; McKinney, D.C.; Sambandamurthy, V.K.; Panda, M.; Ravishankar, S. Pyrazolopyrimidines Establish MurC as a Vulnerable Target in Pseudomonas Aeruginosa and Escherichia Coli. ACS Chem. Biol. 2014, 9 (10), 2274–82.
- Brawn, R.A.; Cook, A.; Omoto, K.; Ke, J.; Karr, C.; Colombo, F.; Virrankoski, M.; Prajapati, S.; Reynolds, D.; Bolduc, D.M.; Nguyen, T.V.; Gee, P.; Borrelli, D.; Caleb, B.; Yao, S.; Irwin, S.; Larsen, N.A.; Selvaraj, A.; Zhao, X.; Ioannidis, S. Discovery of Aminopyrazole Derivatives as Potent Inhibitors of Wild-Type and Gatekeeper Mutant FGFR2 and 3. ACS. Med. Chem. Lett. 2021, 12 (1), 93–98.
- Fekri, A.; Keshk, E.M.; Khalil, A.M.; Taha, I. Synthesis of Novel Antioxidant and Antitumor 5-Aminopyrazole Derivatives, 2D/3D QSAR, and Molecular Docking. Mol. Divers. 2022, 26, 781–800.
- King, H.M.; Rana, S.; Kubica, S.P.; Mallareddy, J.R.; Kizhake, S.; Ezell, E.L.; Zahid, M.; Naldrett, M.J.; Alvarez, S.; Law, H.C.; Woods, N.T.; Natarajan, A. Aminopyrazole Based CDK9 PROTAC Sensitizes Pancreatic Cancer Cells to Venetoclax. Bioorg. Med. Chem. Lett. 2021, 43, 128061.
- Pilakowski, J.; Baumann, G.; Shih, Y.H.; Meckel, T.; Schmidt, B. Design, Synthesis and Biological Evaluation of Novel Aminopyrazole- and 7-Azaindole-Based Nek1 Inhibitors and Their Effects on Zebrafish Kidney Development. Bioorg. Med. Chem. Lett. 2021, 53, 128418.
- Pevarello, P.; Brasca, M.G.; Amici, R.; Orsini, P.; Traquandi, G.; Corti, L.; Piutti, C.; Sansonna, P.; Villa, M.; Pierce, B.S.; Pulici, M.; Giordano, P.; Martina, K.; Fritzen, E.L.; Nugent, R.A.; Casale, E.; Cameron, A.; Ciomei, M.; Roletto, F.; Isacchi, A.; Fogliatto, G.; Pesenti, E.; Pastori, W.; Marsiglio, A.; Leach, K.L.; Clare, P.M.; Fiorentini, F.; Varasi, M.; Vulpetti, A.; Warpehoski, M.A. 3-Aminopyrazole Inhibitors of CDK2/Cyclin A as Antitumor Agents. 1. Lead Finding. J. Med. Chem. 2004, 47 (13), 3367–80.
- Rana, S.; Sonawane, Y.A.; Taylor, M.A.; Kizhake, S.; Zahid, M.; Natarajan, A. Synthesis of Aminopyrazole Analogs and Their Evaluation as CDK Inhibitors for Cancer Therapy. Bioorg. Med. Chem. Lett. 2018, 28 (23-24), 3736–3740.
- Almehmadi, S.J.; Alsaedi, A.M.R.; Harras, M.F.; Farghaly, T.A. Synthesis of a New Series of Pyrazolo[1,5-a]Pyrimidines as CDK2 Inhibitors and Anti-Leukemia. Bioorg. Chem. 2021, 117, 105431.
- Dalinger, I.L.; Vatsadse, I.A.; Shkineva, T.K.; Popova, G.P.; Ugrak, B.I.; Shevelev, S.A. Nitropyrazoles. Russ. Chem. Bull. 2010, 59 (8), 1631–1638.
- Ravi, P.; Tewari, S.P. Facile and Environmentally Friendly Synthesis of Nitropyrazoles Using Montmorillonite K-10 Impregnated with Bismuth Nitrate. Catal. Commun. 2012, 19, 37–41.
- Ravi, P.; Gore, G.M.; Tewari, S.P.; Sikder, A.K. A Simple and Environmentally Benign Nitration of Pyrazoles by Impregnated Bismuth Nitrate. J. Heterocyclic. Chem. 2013, 50 (6), 1322–1327.
- Tairov, M.A.; Levchenko, V.; Stadniy, I.A.; Dmytriv, Y.V.; Dehtiarov, S.O.; Kibalnyi, M.O.; Melnyk, A.V.; Veselovych, S.Y.; Borodulin, Y.V.; Kolotilov, S.V.; Ryabukhin, S.V.; Volochnyuk, D.M. Practical Synthetic Method for Functionalized 1-Methyl-3/5-(Trifluoromethyl)−1H-Pyrazoles. Org. Proc. Res. Devel. 2020, 24 (11), 2619–2632.
- Mercuri, G.; Moroni, M.; Domasevitch, K.V.; Di Nicola, C.; Campitelli, P.; Pettinari, C.; Giambastiani, G.; Galli, S.; Rossin, A. Carbon Dioxide Capture and Utilization with Isomeric Forms of bis(Amino)-Tagged Zinc Bipyrazolate Metal-Organic Frameworks. Chemistry 2021, 27 (14), 4746–4754.
- Quinn, J.F.; Bryant, C.E.; Golden, K.C.; Gregg, B.T. Rapid Reduction of Heteroaromatic Nitro Groups Using Catalytic Transfer Hydrogenation with Microwave Heating. Tetrahedron Lett. 2010, 51 (5), 786–789.
- Lohou, E.; Collot, V.; Lohou, E.; Stiebing, S.; Rault, S. Direct Access to 3-Aminoindazoles by Buchwald-Hartwig C-N Coupling Reaction. Synthesis 2011, 2011 (16), 2651–2663.
- Su, M.; Hoshiya, N.; Buchwald, S.L. Palladium-catalyzed Amination of Unprotected Five-Membered Heterocyclic Bromides. Org. Lett. 2014, 16 (3), 832–5.
- Wood, S.D.; Grant, W.; Adrados, I.; Choi, J.Y.; Alburger, J.M.; Duckett, D.R.; Roush, W.R. In Silico HTS and Structure Based Optimization of Indazole-derived ULK1 Inhibitors. ACS. Med. Chem. Lett. 2017, 8 (12), 1258–1263.
- Bollenbach, M.; Lugnier, C.; Kremer, M.; Salvat, E.; Megat, S.; Bihel, F.; Bourguignon, J.J.; Barrot, M.; Schmitt, M. Design and Synthesis of 3-Aminophthalazine Derivatives and Structural Analogues as PDE5 Inhibitors: Anti-Allodynic Effect Against Neuropathic Pain in a Mouse Model. Eur. J. Med. Chem. 2019, 177, 269–290.
- Thalji, R.K.; Raha, K.; Andreotti, D.; Checchia, A.; Cui, H.; Meneghelli, G.; Profeta, R.; Tonelli, F.; Tommasi, S.; Bakshi, T.; Donovan, B.T.; Howells, A.; Jain, S.; Nixon, C.; Quinque, G.; McCloskey, L.; Bax, B.D.; Neu, M.; Chan, P.F.; Stavenger, R.A. Structure-guided Design of Antibacterials That Allosterically Inhibit DNA Gyrase. Bioorg. Med. Chem. Lett. 2019, 29 (11), 1407–1412.
- Wang, X.R.; Wang, S.; Li, W.B.; Xu, K.Y.; Qiao, X.P.; Jing, X.L.; Wang, Z.X.; Yang, C.J.; Chen, S.W. Design, Synthesis and Biological Evaluation of Novel 2-(4-(1H-Indazol-6-yl)-1H-Pyrazol-1-yl)Acetamide Derivatives as Potent VEGFR-2 Inhibitors. Eur. J. Med. Chem. 2021, 213, 113192.
- Abunada, N.; Hassaneen, H.; Kandile, N.; Miqdad, O. Synthesis and Antimicrobial Activity of Some New Pyrazole, Fused Pyrazolo[3,4-d]-pyrimidine and Pyrazolo[4,3-e][1,2,4]-triazolo[1,5-c]pyrimidine Derivatives. Molecules 2008, 13 (7), 1501–1517.
- Zhdankin, V.V.; Anwar, H.F.; Elnagdi, M.H. Recent Developments in Aminopyrazole Chemistry. Arkivoc 2009, 2009 (1), 198–250.
- Moy, F.J.; Lee, A.; Gavrin, L.K.; Xu, Z.B.; Sievers, A.; Kieras, E.; Stochaj, W.; Mosyak, L.; McKew, J.; Tsao, D.H. Novel Synthesis and Structural Characterization of a High-Affinity Paramagnetic Kinase Probe for the Identification of non-ATP Site Binders by Nuclear Magnetic Resonance. J. Med. Chem. 2010, 53 (3), 1238–49.
- Labroli, M.A.; Dwyer, M.P.; Poker, C.; Keertikar, K.M.; Rossman, R.; Guzi, T.J. A Convergent Preparation of the CHK1 Inhibitor MK-8776 (SCH 900776). Tetrahedron Lett. 2016, 57 (24), 2601–2603.
- Guillard, J.; Goujon, F.; Badol, P.; Poullain, D. New Synthetic Route to Diaminonitropyrazoles as Precursors of Energetic Materials. Tetrahedron Lett. 2003, 44 (31), 5943–5945.
- Langer, P.; Pfeiffer, W.-D.; Dilk, E.; Roßberg, H. Synthesis and Reactivity of 3,4-dimethyl-4H-1,3,4-thiadiazines. Synlett. 2003, 15, 2392–2394.
- Wangngae, S.; Pattarawarapan, M.; Phakhodee, W. Ph3P/I2-Mediated Synthesis of N,N′,N″-substituted Guanidines and 2-iminoimidazolin-4-ones from Aryl Isothiocyanates. J. Org. Chem. 2017, 82 (19), 10331–10340.
- Li, J.-S.; Xie, X.-Y.; Jiang, S.; Yang, P.-P.; Li, Z.-W.; Lu, C.-H.; Liu, W.-D. Reagent-free Aerobic Oxidative Synthesis of Amides from Aldehydes and Isothiocyanates. Org. Chem. Front. 2021, 8 (4), 697–701.
- Yang, J.; Chen, L.; Dong, Y.; Zhang, J.; Wu, Y. Di-tert-butyl Peroxide (DTBP)-Mediated Synthesis of Symmetrical N,N′-Disubstituted Urea/Thiourea Motifs from Isothiocyanates in Water. Synth. Commun. 2021, 52 (1), 63–78.
- Fantinati, A.; Morciano, G.; Turrin, G.; Pedriali, G.; Pacifico, S.; Preti, D.; Albanese, V.; Illuminati, D.; Cristofori, V.; Giorgi, C.; Tremoli, E.; Pinton, P.; Trapella, C. Identification of Small-Molecule Urea Derivatives as PTPC Modulators Targeting the c Subunit of F1/Fo-ATP Synthase. Bioorg. Med. Chem. Lett. 2022, 72, 128822.
- Yang, C.L.; Gao, X.J.; Jiang, X.Y.; Shi, Z.; Hao, E.J.; Dong, Z.B. Synthesis of Unsymmetric Thiosulfonates Starting from N-Substituted O-Thiocarbamates: Easy Access to the S-SO2 Bond. J. Org. Chem. 2022, 87 (17), 11656–11668.
- Bolli, M.H.; Abele, S.; Binkert, C.; Bravo, R.; Buchmann, S.; Bur, D.; Gatfield, J.; Hess, P.; Kohl, C.; Mangold, C.; Mathys, B.; Menyhart, K.; Muller, C.; Nayler, O.; Scherz, M.; Schmidt, G.; Sippel, V.; Steiner, B.; Strasser, D.; Treiber, A.; Weller, T. 2-Imino-thiazolidin-4-one Derivatives as Potent, Orally Active S1P1 Receptor Agonists. J. Med. Chem. 2010, 53 (10), 4198–211.
- Rostamizadeh, S.; Aryan, R.; Reza Ghaieni, H.; Mohammad Amani, A. Efficient Synthesis of 1,3,4-Thiadiazoles Using Hydrogen Bond Donor (Thio)Urea Derivatives as Organocatalysts. J. Heterocyclic. Chem. 2010, 47, 616.
- Yella, R.; Khatun, N.; Rout, S.K.; Patel, B.K. Tandem Regioselective Synthesis of Tetrazoles and Related Heterocycles Using Iodine. Org. Biomol. Chem. 2011, 9 (9), 3235–3245.
- Guin, S.; Rout, S.K.; Gogoi, A.; Nandi, S.; Ghara, K.K.; Patel, B.K. Desulfurization Strategy in the Construction of Azoles Possessing Additional Nitrogen, Oxygen or Sulfur Using a Copper(I) Catalyst. Adv. Synth. Catal. 2012, 354 (14-15), 2757–2770.
- Polucci, P.; Magnaghi, P.; Angiolini, M.; Asa, D.; Avanzi, N.; Badari, A.; Bertrand, J.; Casale, E.; Cauteruccio, S.; Cirla, A.; Cozzi, L.; Galvani, A.; Jackson, P.K.; Liu, Y.; Magnuson, S.; Malgesini, B.; Nuvoloni, S.; Orrenius, C.; Sirtori, F.R.; Riceputi, L.; Rizzi, S.; Trucchi, B.; O’Brien, T.; Isacchi, A.; Donati, D.; D’Alessio, R. Alkylsulfanyl-1,2,4-triazoles, a New Class of Allosteric Valosine Containing Protein Inhibitors. Synthesis and Structure–Activity Relationships. J. Med. Chem. 2013, 56 (2), 437–450.
- Chandrasekhar, A.; Ramkumar, V.; Sankararaman, S. Palladium Catalyzed Carbonylative Annulation of the C(sp2)-H Bond of N,1-diaryl-1H-tetrazol-5-amines and N,4-diaryl-4H-triazol-3-amines to Quinazolinones. Org. Biomol. Chem. 2018, 16 (44), 8629–8638.
- Prevost, J.R.C.; Kozlova, A.; Es Saadi, B.; Yildiz, E.; Modaffari, S.; Lambert, D.M.; Pochet, L.; Wouters, J.; Dolušić, E.; Frédérick, R. Convenient One-pot Formation of Highly Functionalized 5-Bromo-2-Aminothiazoles, Potential Endocannabinoid Hydrolase MAGL Inhibitors. Tetrahedron Lett. 2018, 59 (49), 4315–4319.
- Patil, M.; Mhaldar, P.; Mahadik, V.; Pore, D.M. Novel, Green and Sustainable Route for Synthesis of 5-aryl-4-phenyl-1,2,4-triazolidine-3-thiones. Tetrahedron Lett. 2020, 61 (25), 152015.
- Fouad, S.A.; El-Gendey, M.S.; Ahmed, E.M.; Hessein, S.A.; Ammar, Y.A.; Zaki, Y.H. Convenient Synthesis of Some New Thiophene, Pyrazole, and Thiazole Derivatives Bearing Biologically Active Sulfonyl Guanidine Moiety. Polycycl. Aromat. Comp. 2021, 42 (9), 6628–6646.
- Kadagathur, M.; Sigalapalli, D.K.; Patra, S.; Tangellamudi, N.D. Microwave-assisted Hydrogen Peroxide-Mediated Synthesis of Benzoxazoles and Related Heterocycles via Cyclodesulfurization. Synth. Commun. 2021, 51 (14), 2213–2224.
- Tantawy, A.H.; El-Behairy, M.F.; Abd-Allah, W.H.; Jiang, H.; Wang, M.Q.; Marzouk, A.A. Design, Synthesis, Biological Evaluation, and Computational Studies of Novel Fluorinated Candidates as PI3 K Inhibitors: Targeting Fluorophilic Binding Sites. J. Med. Chem. 2021, 64 (23), 17468–17485.
- Xu, Y.; Li, F.; Zhao, N.; Su, J.; Wang, C.; Wang, C.; Li, Z.; Wang, L. Environment-friendly and Efficient Synthesis of 2-Aminobenzo-Xazoles and 2-Aminobenzothiazoles Catalyzed by Vitreoscilla Hemoglobin Incorporating a Cobalt Porphyrin Cofactor. Green Chem. 2021, 23 (20), 8047–8052.
- Han, L.; Gan, L.; Hu, X.; Li, W.; Zhu, D.; Zheng, J.; Wu, Y.; Yu, Y.; Gan, Z. Cu(OAc)2 Mediated Mild Synthesis of 2-aminobenzimidazoles and 2-aminobenzoxazoles. Synth. Commun. 2022, 52 (7), 1050–1058.
- Hu, Y.; Chen, L.; Zou, C.; He, J.; Feng, L.; Wu, J.Q.; Chen, W.H.; Hu, J. Access to 3-amino-[1,2,4]-triazolo Pyridines and Related Heterocycles Via Electrochemically Induced Desulfurative Cyclization. Org. Lett. 2022, 24 (28), 5137–5142.
- Klug, T.; Cronin, A.; O'Brien, E.; Schioldager, R.; Johnson, H.; Gleason, C.; Schmid, C.; Soderberg, N.; Manjunath, A.; Liyanage, D.; Lazaro, H.; Kimball, J.J.; Eagon, S. Microwave Mediated Synthesis of 2-Aminooxazoles. Tetrahedron Lett. 2022, 88, 153555.
- Lim, J.H.; Baek, S.E.; Lad, B.S.; Kim, J. Synthesis of 2-Imino-1,3,4-Oxadiazolines from Acylhydrazides and Isothiocyanates via Aerobic Oxidation and a DMAP-Mediated Annulation Sequence. ACS Omega 2022, 7 (32), 28148–28159.
- Reda Aouad, M.; Almehmadi, M.A.; Faleh Albelwi, F.; Teleb, M.; Tageldin, G.N.; Abu-Serie, M.M.; Hagar, M.; Rezki, N. Targeting the Interplay Between MMP-2, CA II and VEGFR-2 via New Sulfonamide-Tethered Isomeric Triazole Hybrids; Microwave-Assisted Synthesis, Computational Studies and Evaluation. Bioorg. Chem. 2022, 124, 105816.
- Bhagat, S.; Chakraborti, A.K. An Extremely Efficient Three-Component Reaction of Aldehydes/Ketones, Amines, and Phosphites (Kabachnik−Fields Reaction) for the Synthesis of α-Aminophosphonates Catalyzed by Magnesium Perchlorate. J. Org. Chem. 2007, 72 (4), 1263–1270.
- Bhagat, S.; Chakraborti, A.K. Zirconium(IV) Compounds As Efficient Catalysts for Synthesis of α-Aminophosphonates. J. Org. Chem. 2008, 73 (15), 6029–6032.
- Bhagat, S.; Shah, P.; Garg, S.K.; Mishra, S.; Kamal Kaur, P.; Singh, S.; Chakraborti, A.K. α-Aminophosphonates as Novel Anti-Leishmanial Chemotypes: Synthesis, Biological Evaluation, and CoMFA Studies. Med. Chem. Commun. 2014, 5 (5), 665–670.
- Bhagat, S.; Supriya, M.; Pathak, S.; Sriram, D.; Chakraborti, A.K. α-Sulfonamidophosphonates as new Anti-Mycobacterial Chemotypes: Design, Development of Synthetic Methodology, and Biological Evaluation. Bioorg. Chem. 2019, 82, 246–252.
- Kumar, D.; Kommi, D.N.; Chopra, P.; Ansari, M.I.; Chakraborti, A.K. L-Proline-catalyzed Activation of Methyl ketones or Active Methylene Compounds and DMF-DMA for Syntheses of (2E)-3-dimethylamino-2-propen-1-ones. Eur. J. Org. Chem. 2012, 2012 (32), 6407–6413.
- Sarkar, A.; Raha Roy, S.; Kumar, D.; Madaan, C.; Rudrawar, S.; Chakraborti, A.K. Lack of Correlation Between Catalytic Efficiency and Basicity of Amines During the Reaction of Aryl Methyl Ketones with DMF-DMA: An Unprecedented Supramolecular Domino Catalysis. Org. Biomol. Chem. 2012, 10 (2), 281–286.
- Chakraborti, A.; Parikh, N.; Roy, S.; Seth, K.; Kumar, A. ‘On-Water’ Multicomponent Reaction for the Diastereoselective Synthesis of Functionalized Tetrahydropyridines and Mechanistic Insight. Synthesis 2016, 48 (04), 547–556.
- Chakraborti, A.; Roy, S.; Jadhavar, P.; Seth, K.; Sharma, K. Organocatalytic Application of Ionic Liquids: [bmim][MeSO4] as a Recyclable Organocatalyst in the Multicomponent Reaction for the Preparation of Dihydropyrimidinones and -Thiones. Synthesis 2011, 2011 (14), 2261–2267.
- Jadhavar, P.S.; Dhameliya, T.M.; Vaja, M.D.; Kumar, D.; Sridevi, J.P.; Yogeeswari, P.; Sriram, D.; Chakraborti, A.K. Synthesis, Biological Evaluation and Structure-Activity Relationship of 2-Styrylquinazolones as Anti-Tubercular Agents. Bioorg. Med. Chem. Lett. 2016, 26 (11), 2663–2669.
- Kumar, D.; Jadhavar, P.S.; Nautiyal, M.; Sharma, H.; Meena, P.K.; Adane, L.; Pancholia, S.; Chakraborti, A.K. Convenient Synthesis of 2,3-Disubstituted Quinazolin-4(3H)-Ones and 2-Styryl-3-Substituted Quinazolin-4(3H)-Ones: Applications Towards the Synthesis of Drugs. RSC Adv. 2015, 5 (39), 30819–30825.
- Kumar, D.; Kommi, D.N.; Bollineni, N.; Patel, A.R.; Chakraborti, A.K. Catalytic Procedures for Multicomponent Synthesis of Imidazoles: Selectivity Control During the Competitive Formation of tri- and Tetrasubstituted Imidazoles. Green Chem. 2012, 14 (7), 2038–2049.
- Kumar, D.; Kumar, A.; Qadri, M.M.; Ansari, M.I.; Gautam, A.; Chakraborti, A.K. In(OTf)3-catalyzed Synthesis of 2-styryl Quinolines: Scope and Limitations of Metal Lewis Acids for Tandem Friedländer Annulation–Knoevenagel Condensation. RSC Adv. 2015, 5 (4), 2920–2927.
- Kumar, D.; Sonawane, M.; Pujala, B.; Jain, V.K.; Bhagat, S.; Chakraborti, A.K. Supported Protic Acid-Catalyzed Synthesis of 2,3-Disubstituted Thiazolidin-4-Ones: Enhancement of the Catalytic Potential of Protic Acid by Adsorption on Solid Supports. Green Chem. 2013, 15 (10), 2872–2884.
- Frisch, M.J.; Trucks, G.W.; Schlegel, H.B.; Scuseria, G.E.; Robb, M.A.; Cheeseman, J.R.; Scalmani, G.; Barone, V.; Petersson, G.A.; Nakatsuji, H.; Li, X.; Caricato, M.; Marenich, A.V.; Bloino, J.; Janesko, B.G.; Gomperts, R.; Mennucci, B.; Hratchian, H.P.; Ortiz, J.V.; Izmaylov, A.F.; Sonnenberg, J.L.; Williams; Ding, F.; Lipparini, F.; Egidi, F.; Goings, J.; Peng, B.; Petrone, A.; Henderson, T.; Ranasinghe, D.; Zakrzewski, V.G.; Gao, J.; Rega, N.; Zheng, G.; Liang, W.; Hada, M.; Ehara, M.; Toyota, K.; Fukuda, R.; Hasegawa, J.; Ishida, M.; Nakajima, T.; Honda, Y.; Kitao, O.; Nakai, H.; Vreven, T.; Throssell, K.; Montgomery Jr., J.A.; Peralta, J.E.; Ogliaro, F.; Bearpark, M.J.; Heyd, J.J.; Brothers, E.N.; Kudin, K.N.; Staroverov, V.N.; Keith, T.A.; Kobayashi, R.; Normand, J.; Raghavachari, K.; Rendell, A.P.; Burant, J.C.; Iyengar, S.S.; Tomasi, J.; Cossi, M.; Millam, J.M.; Klene, M.; Adamo, C.; Cammi, R.; Ochterski, J.W.; Martin, R.L.; Morokuma, K.; Farkas, O.; Foresman, J.B.; Fox, D.J. Gaussian 16 Rev. B.01; Wallingford, CT, 2016.
- Lu, T.; Chen, Q. Shermo: A General Code for Calculating Molecular Thermochemistry Properties. Comput. Theor. Chem. 2021, 1200, 113249.
- Eberhardt, J.; Santos-Martins, D.; Tillack, A.F.; Forli, S. AutoDock Vina 1.2.0: New Docking Methods, Expanded Force Field, and Python Bindings. J. Chem. Inf. Model. 2021, 61 (8), 3891–3898.
- Laskowski, R.A.; Swindells, M.B. LigPlot+: Multiple Ligand-Protein Interaction Diagrams for Drug Discovery. J. Chem. Inf. Model. 2011, 51 (10), 2778–2786.
- Schrödinger, L.; DeLano, W. PyMOL, 2020.
- Lu, T.; Chen, F. Multiwfn: A Multifunctional Wavefunction Analyzer. J. Comput. Chem. 2012, 33 (5), 580–592.
- Lu, T.; Chen, Q. A Simple Method of Identifying π Orbitals for non-Planar Systems and a Protocol of Studying π Electronic Structure. Theor. Chem. Acc. 2020, 139 (2), 25.
- Sanner, M.F. Python: A Programming Language for Software Integration and Development. J. Mol. Graph. Model. 1999, 17 (1), 57–61.
- Momma, K.; Izumi, F. VESTA 3 for Three-dimensional Visualization of Crystal, Volumetric and Morphology Data. J. Appl. Crystallogr. 2011, 44 (6), 1272–1276.