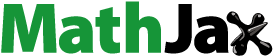
ABSTRACT
During biopharmaceutical process development, it is important to improve titer to reduce drug manufacturing costs and to deliver comparable quality attributes of therapeutic proteins, which helps to ensure patient safety and efficacy. We previously reported that relative high-iron concentrations in media increased titer, but caused unacceptable coloration of a fusion protein during early-phase process development. Ultimately, the fusion protein with acceptable color was manufactured using low-iron media, but the titer decreased significantly in the low-iron process. Here, long-term passaging in low-iron media is shown to significantly improve titer while maintaining acceptable coloration during late-phase process development. However, the long-term passaging also caused a change in the protein charge variant profile by significantly increasing basic variants. Thus, we systematically studied the effect of media components, seed culture conditions, and downstream processing on productivity and quality attributes. We found that removing β-glycerol phosphate (BGP) from basal media reduced basic variants without affecting titer. Our goals for late-phase process development, improving titer and matching quality attributes to the early-phase process, were thus achieved by prolonging seed culture age and removing BGP. This process was also successfully scaled up in 500-L bioreactors. In addition, we demonstrated that higher concentrations of reactive oxygen species were present in the high-iron Chinese hamster ovary cell cultures compared to that in the low-iron cultures, suggesting a possible mechanism for the drug substance coloration caused by high-iron media. Finally, hypotheses for the mechanisms of titer improvement by both high-iron and long-term culture are discussed.
Introduction
The cumulative sales of biologic drugs surpassed $140 billion annually in 2013, of which monoclonal antibodies (mAbs) and Fc fusion proteins reached $75.7 billion per year; in particular, etanercept (Enbrel®), the best-selling Fc fusion protein, reached $8.8 billion per year.Citation1 Fc fusion proteins comprise the Fc portion of an antibody fused to heterologous targeting or effector domains, such as cell surface receptors, cytokines, or other ligands.Citation2 Fc fusion proteins are successful alternatives to mAbs for therapeutic application, with 11 approvals in the United States or European Union by the end of 2016.Citation3 The biopharmaceutical industry has thus been successful in developing these products, but companies must remain competitive and are under pressure to reduce costs. Moreover, there is market competition among biosimilars and their reference protein drugs after patent expiration (e.g., Enbrel vs its biosimilar products Etacept, Benepali and GP2015).Citation4,Citation5 Reducing the costs of biomanufacturing to ensure that these biologic drugs can reach more patients remains a major challenge.Citation6
During process development, the cost of biomanufacturing can be reduced by increasing upstream titer and increasing downstream yield. Downstream yields now regularly approach 70–80%, suggesting limited potential for future increase.Citation7 By contrast, upstream titer has increased more than 10-fold, to an average of ∼ 3 g/L, in manufacturing over the past 30 years, and substantial potential for further improvement remains.Citation7 For example, lab-scale titers of ∼ 10 g/L have been reported in Chinese hamster ovary (CHO) fed-batch cultures,Citation8,Citation9 which demonstrates the potential for future manufacturing improvement. Therefore, increasing upstream titer will continue to play a critical role in increasing the output and reducing the costs of biomanufacturing.
In addition to titer, the consistent quality of biologics products is important to ensure patient safety and drug efficacy.Citation6 In order to reduce raw material lot-to-lot variation, there has been a trend in the industry towards use of chemically defined media to replace serum and hydrolysate media, but some of the components in these chemically defined media may have adverse effects on protein quality attributes such as drug substance color,Citation10-13 charge variants,Citation14-17 and glycosylation.Citation18-20 Since there may be more than 50 components in chemically defined media, it remains challenging to fully understand the effects and interactions of those components on cell culture performance and final protein quality.Citation13,Citation21,Citation22 Although the downstream process is critical to control the quality attributes of final formulated biologic drug substances, it is quite common that quality issues originate from characteristics of the upstream process such as cell lines,Citation21,Citation23 mediaCitation9,Citation13,Citation24 and bioreactor operation conditions.Citation25,Citation26 Since downstream processes cannot remove some of those impurities to meet the quality requirements, upstream parameters often need to be optimized, potentially sacrificing upstream titer by changing medium formulations, shifting culture temperature and even shortening culture duration.Citation13,Citation27,Citation28
Protein drug substance color is an important quality attribute for final quality control and stability studies.Citation29 Drug substance color can be influenced by components of upstream cell culture media, such as hydroxocobalamin or vitamin B12,Citation10,Citation11,Citation13,Citation29,Citation30 other B vitamins,Citation12 or iron.Citation12,Citation13,Citation29 We previously showed that the drug substance color of a Fc fusion protein was significantly affected by high levels of iron in the cell culture media.Citation13 The high concentration of iron caused tryptophan oxidation of the Fc fusion protein, which resulted in drug substance coloration. The brown color caused by high-iron, which cannot be removed by downstream purification, did not pass appearance testing, and thus the drug substance would not be acceptable for release.Citation13 In order to manufacture drug substances with acceptable color, chemically defined media with a low-iron concentration (≤ 20 µM) had to be used for clinical manufacturing, which resulted in a much lower titer.Citation13 Iron is a critical medium component for CHO cell growth, and high levels of iron (≥ 50 µM), i.e., 110 µM,Citation13 50–80 µM,Citation12 and 100–500 µM,Citation31 have been reported to improve cell culture titer significantly. Thus, reduction of iron could be expected to have significant detrimental effects on the titer of a bioprocess. An effective upstream strategy to improve titer in low-iron chemically defined media while maintaining acceptable drug substance color has not yet been reported.
In addition to color, the protein charge variant profile is another important quality attribute for patient safety, drug efficacy and process consistency.Citation32,Citation33 Several medium components at high concentrations, such as iron,Citation12 copper,Citation16 and vitamin C,Citation14 have been found to affect charge variant profiles. Due to the complexity of the process, it can be challenging to improve titer from early-phase to late-phase development while maintaining similar protein charge variant profiles.
Here, a case study on late-phase process development (Process B) is presented for production of a Fc fusion protein; the early-phase process development (Process A), using the same cell line, to improve drug substance color with the sacrifice of titer in low-iron media was previously reported.Citation13 To improve titer for the late-phase process development, we studied the effects of different iron concentrations and seed passages on cell culture performance and final quality attributes. In a prototype of Process B, we found that the titer was significantly improved after the long-term passaging of CHO cells in low-iron media. However, this long-term passaging led to a change in the charge variant profile. To match the desired charge variant profile, a full factorial design-of-experiments (DOE) study on seed passages and different medium components was performed, which identified that β-glycerol phosphate (BGP) had a significant impact on the charge variant profile. The final Process B using long-passage CHO cells in low-iron media with BGP removed was demonstrated in 500-L bioreactors to achieve a good titer with all quality attributes matching Process A. In addition, to improve understanding of the mechanism of the protein drug substance coloration caused by high-iron media, we measured reactive oxygen species (ROS) in different cell culture conditions. We demonstrated that higher ROS were present in the high-iron compared to that in the low-iron conditions and that lower ROS were present in the long-term culture than in the standard culture at manufacturing age.
Results
Impact of iron on CHO cell culture performance
In the first experiment, iron concentrations were titrated from 10 to 110 µM (). The main purpose was to determine whether we could identify a middle level iron concentration that could improve titer, but not cause drug substance coloration. We found that peak viable cell density (VCD) linearly correlated with iron concentration, increasing by ∼26% from 8.7 × 106 cells/mL to 11 × 106 cells/mL as iron concentration increased from 10 to 110 µM (). Although the final viability also increased with iron concentration at a statistically significant level, the magnitude of this viability increase was relatively small (∼ 6%; ). The final titers were almost linearly correlated with iron concentrations, increasing up to 37% in the highest iron condition compared with the lowest iron condition (). The titer increase was partially due to an increase in specific productivity (qp) by 10% with an increase of iron from 10 to 110 µM (). The specific consumption rates of nutrients such as glutamine () and glutamate () increased, while the toxic metabolite ammonium production rate qNH4 () decreased with increasing iron concentrations. Since the peak ammonium concentration reached about 8 mmol/L in the cell culture, the reduction of qNH4 by high-iron conditions should benefit cell culture performance. The lactate production rate and overall lactate concentrations were low for all conditions tested for this experiment (data not shown).
Figure 1. Impact of iron concentrations in basal production media on CHO cell cultures at passage 9 in fed-batch production 250-mL shake flasks for 12 days (n = 3): One-way analysis of (1-a) peak viable cell density (VCD) (P < 0.0001), (1-b) final viability (P < 0.01), (1-c) final titer (P < 0.0001), (1-d) specific productivity (qP) (P < 0.0001), (1-e) specific consumption rate of glutamic acid (qGlu) (P < 0.0001), (1-f) specific consumption rate of glutamine (qGln) (P< 0.001), and (1-g) specific production rate of ammonium (qNH4) (P < 0.0001).
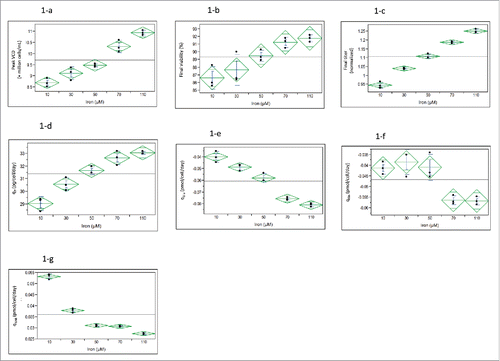
Impact of seed passages on CHO cell culture performance
During process development, we found that the longer seed passages improved titer in low-iron media, which was unexpected. Thus, the effect of different seed passages was studied in detail using chemically defined media containing 10 µM iron (). Seeds between passages 7–11 from the vial thaw of master cell bank (MCB) vials are usually sufficient to expand the seed culture for clinical good-manufacturing-practices (GMP) manufacturing. Therefore, seed passages between 5 and 13 (i.e., 2 additional passages at both the low and high ends) were used for this experiment. Peak VCD () and final titer () almost linearly increased when the seed passage increased from 5 to 13, but the final viability () and qp () had no significant difference between the different seed passages. The nutrient consumption rates of qGlu () and qGln () increased, while the toxic production rate of qNH4 () was reduced with the increase of passage numbers.
Figure 2. Impact of seed passage numbers on CHO cell cultures using low-iron media in fed-batch production 250-mL shake flasks for 12 days (n = 3): One-way analysis of (2-a) peak VCD (P < 0.0001), (2-b) final viability (P = 0.188), (2-c) final titer (P < 0.0001), (2-d) qP (P = 0.307), (2-e) qGlu (P < 0.0001), (2-f) qGln (P < 0.001), and (2-g) qNH4 (P < 0.0001).
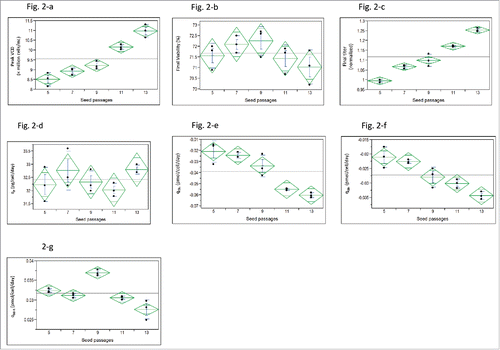
Because the protein titer continued to increase during the manufacturing seed passage range between 5 and 13 (), we hypothesized that the trend would continue and that passages longer than passage 13 (P13) would produce even higher titer. Therefore, longer term seed passages up to 33 were examined. Protein titer almost linearly increased from P8 to P18, and then maintained at a similar high level from P18 to P33 (). However, qp values decreased when the passages were beyond P23 (), which was mainly due to the fact that peak VCD increased with increasing passages from P23 to P33 (), but titer remained at the same level ().
Figure 3. One-way analysis of (3-a) Peak VCD (P < 0.0001), (3-b) Day14 titer (normalized) (P < 0.0001) and (3-c) qP (P = 0.0197) in fed-batch production 125-mL shake flasks containing low-iron media after 7–8 passages of master cell bank (MCB) and different development cell banks (DCBs) (n = 4). Passage 8: P8 seed from MCB vial thaw; Passage 12: P7 seed from the P5-DCB made from 5th passage of MCB; Passage 15: P7 seed from the P8-DCB; Passage 18: P8 seed from the P10-DCB; Passage 20: P8 seed from the P12-DCB; Passage 23: P8 seed from the P15-DCB; Passage 28: P8 seed from the P20-DCB; Passage 33: P8 seed from the P25-DCB.
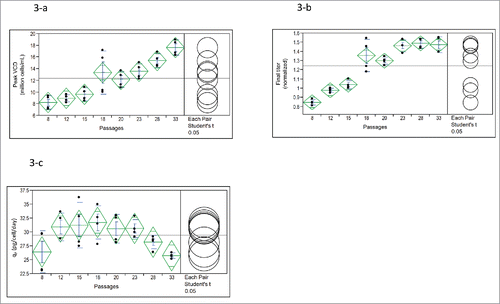
Drug substance color evaluation at 7-L bioreactor scale
Based on the aforementioned results from shake flasks, it is clear that the upstream titer can be improved after longer term seed culture in low-iron media. We then sought to evaluate whether long-term seed culture would cause drug substance coloration, as the drug substance coloration was a major concern for this particular protein.Citation13 Therefore, 7-L bioreactor cultures with cells at passage 20 and with different iron concentrations were performed for the evaluation of both productivity and drug substance color (). The peak VCD and final viability were similar for all 7-L bioreactors, while a relatively high titer was achieved for all conditions with iron concentrations ranging from 20 to 50 µM (). In addition, the drug substance color was pale yellow for the 7-L bioreactor condition at P20 with 20 µM iron, which was similar to the drug substance produced using Process A with 10 µM iron and P9 seed at the 600-L scale (). The drug substance produced with the increased iron concentration of 50 µM iron exhibited a light brown color, which was darker than the drug substance color produced by the 10 µM iron condition but lighter than the color for the 110 µM iron condition ().
Table 1. Impact of iron concentrations on CHO cell culture performance in 7-L bioreactors using long-term seed culture at P20 from MCB vial thaw (n = 2).
Charge variant profile for prototype Process B
Since the 7-L run achieved a good titer with acceptable drug substance color ( and ), the prototype Process B using long-term passage seeds in low-iron media was scaled up in both 50-L and 500-L bioreactors. Although both titer () and final drug substance color (data not shown) were good, as expected, the charge variant profile did not fully match the early-phase Process A (). The prototype Process B resulted in a significantly lower main peak and a much higher basic peak than Process A (). To identify the root cause, the charge variant profile at different steps of downstream processing was examined (). While the anion exchange chromatography step led to significant changes in the amounts of acidic and main species, basic species were relatively constant throughout all downstream processing steps (). Therefore, we concluded that the difference of basic species between Process A and prototype Process B was mainly due to upstream changes rather than downstream processing.
Figure 5. Scale-up comparison of (5-a) viable cell density and (5-b) titer for different processes: Tox process contained 110 µM iron and 0.9 g/L BGP inoculated with P9 seeds (n = 2 at 500-L scale); Process A contained 10 µM iron and 0.9 g/L BGP inoculated with P9 seeds (n = 5 at 600-L scale); prototype Process B contained 20 µM iron and 0.9 g/L BGP inoculated with P20 seeds (n = 2 with one each at 50-L and 500-L scale); Process B contained 20 µM iron and without BGP inoculated with P20 seeds (n = 2 at 500-L scale).
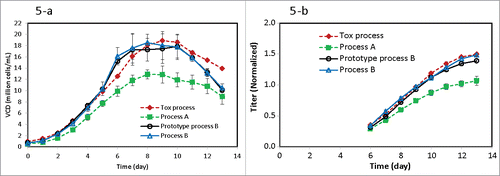
Table 2. Scale-up comparison of charge variant profiles for the final formulated drug substances made from different processes described in .
Figure 6. One-way analysis of (6-a) acidic species (P < 0.0001), (6-b) main species (P < 0.0001), and (6-c) basic species (P = 0.798) during downstream purification steps (n = 7). PAVIB: Protein A viral inactivation bulk pool; AEX pool: anion exchange chromatography pool; CEX pool: Cation exchange chromatography pool.
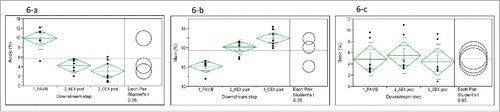
Impact of iron concentrations and cell ages on basic variants and reactive oxygen species (ROS) production
To identify the root cause for the high amounts of basic species observed in the prototype Process B, 4 different upstream parameters were investigated via full factorial DOE design (). The basic species increased with the culture duration from day 10 to 14 for most conditions (). However, shortening cell culture duration from day 14 to day 10 may not be a good strategy for late-phase development because of the significant titer reduction (). Both long-term seed passage and 0.9 g/L BGP significantly increased basic species (P < 0.0001), while both iron and galactose slightly decreased basic species (). Based on a side-by-side comparison in low-iron media, the amounts of basic species in the P23 conditions (#9-12) were about doubled in comparison to the amounts of basic species in the P8 conditions (#1-4) (). Therefore, long-term passage seeds significantly contributed to the high amounts of basic species observed in the prototype Process B. Based on a side-by-side comparison between the conditions without BGP (#1-2 and #9-10) and the conditions containing 0.9 g/L BGP (#3-4 and #11-12) in low-iron media, the amounts of basic species were reduced by about 50% by removing BGP (). In fact, the amounts of basic species were similar between the conditions at passage 8 with 0.9 g/L BGP (#3-4) and the conditions at P23 without BGP (#9-10) (). It should be mentioned that BGP had little impact on cell culture performance other than increasing basic species for this shake flask experiment (data not shown). Therefore, removing BGP in basal media reduced basic species for the prototype Process B, enabling Process B (with long-term seed passages in low-iron media) to match both drug substance color and charge variant profile in Process A and to achieve a higher titer than Process A.
Table 3. Full factorial design for the study on cell culture performance, charge variant profile and reactive oxygen species (ROS) using different seed passages, different concentrations of iron and β-glycerophosphate (BGP) in the production basal medium and different concentrations of galactose (Gal) in the feed medium in fed batch production 250-mL shake flasks (n = 3).
Figure 7. Impact of different upstream process parameters () in fed-batch production 250-mL shake flasks (n = 3): (7-a) on basic variants on day10 (D10), day12 (D12) and day14 (D14); (7-b) on the prediction profiles.
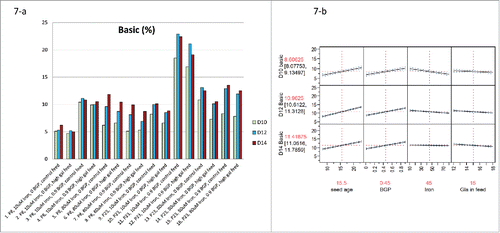
It was hypothesized that the higher level of iron would generate more ROS via Felton reaction, and thus result in higher oxidative stress, which would cause tryptophan oxidation and yellow drug substance formation during cell culture.Citation13 To test this hypothesis, ROS in cell cultures under the DOE design conditions () were measured on day 3, day 6 and day 10 (). In agreement with this hypothesis, the high-iron conditions produced significantly greater ROS on day 6 and day 10 during fed-batch cell culture, although the ROS on day 3 was slightly greater in the high-iron conditions than the low-iron conditions (). This may be due to the fact that high-iron was added in the fed-batch production media rather than seed passaging media, which took more than 3 days to have a significant effect on cell culture performance, e.g., ROS formation in the production cell culture. In addition, the production cultures inoculated from the long-term P23 seed produced significantly less ROS than those inoculated from the P8 seed on all three days with a greater ROS reduction from 60 to 45 (Arbitrary Unit) during early cell culture, e.g., day 3 ().
Figure 8. Impact of different iron concentrations and seed passages on reactive oxygen species (ROS) residuals on day 3 (n = 4 for 12 g/L galactose conditions only), day 6 (n = 8 for all DOE conditions) and day 10 (n = 8) in the fed-batch production 250-mL shake flasks from the DOE design shown in .
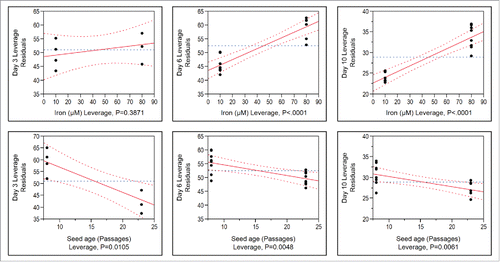
Scale-up manufacturing
In order to confirm our lab findings during late-phase process development, scale-up bioreactor runs were performed for Process B with long-term seeds and low-iron media without BGP (). Compared with Process A with low-iron and seeds at P9, both VCD () and titer () were significantly improved by either higher iron (Tox process) or longer term culture seed at P20 (prototype Process B or Process B) at similar manufacturing scales. In addition, the final formulated drug substance color was pale yellow for Process B at the 500-L scale (data not shown), which was the same as Process A and much lighter than the Tox process, which exhibited an undesirable brown color (). In addition, the charge variant profile including main, acidic and basic variants for Process B matched those profiles exhibited in Process A (). Therefore, the scale-up production for Process B demonstrated that the long-term seed culture in low-iron media without BGP produced good titer while maintaining acceptable final formulated drug substance color and charge variant profile. In addition, all other quality attributes such as N-glycan profiles, results of size-exclusion chromatography and capillary electrophoresis sodium dodecyl sulfate analysis, and amounts of host cell proteins, residual Protein A and DNA were comparable between Process A and Process B (data not shown).
Discussion
To the best of our knowledge, an effective cell culture strategy has not been reported for titer improvement while maintaining acceptable drug substance color for cell culture processes negatively impacted by high-iron concentrations. In our previous report, a low-iron medium was used for clinical GMP manufacturing of a Fc fusion protein in order to ensure acceptable color for the final drug substance, even though the titer was significantly reduced.Citation13 Here, we demonstrated for the first time that a CHO cell culture after long-term passaging can effectively achieve a high titer in low-iron media, and titer was comparable to the high-iron process. The higher titer by long-term culture in low-iron media is likely due to higher VCD with the same qP, which would have resulted from lower ROS formation (). Furthermore, higher consumption rates of nutrients (e.g., qGln and qGlu), and lower production rate of the toxic chemical ammonium were observed with an increase of basic variants in long-term culture (). Although the basic variant profile may not be a critical quality attribute for the drug substance comparability, increasing basic variants, resulting in lower main species, is generally not desirable in late-phase process development. Therefore, long-term passaging combined with subsequent medium optimization (i.e., removing BGP, which decreased basic variants), enabled titer improvement to the high-iron levels in low-iron media while matching all quality attributes of the high-iron process.
Table 4. Impact of high-iron and long-term culture adapted in low-iron media on CHO cell performance summarized from this study and literature.
Iron, a vital element for all live organisms, can be transported into mammalian cells in in vivo and in vitro cultures using serum media through a high-affinity transferrin-bound iron uptake system.Citation34,Citation35 In addition, non-transferrin-bound iron (NTBI) uptakes have been intensively studied in many different mammalian cell cultures (but not in CHO cells) using serum-free media without transferrin.Citation36-39 In general, two NTBI uptake mechanisms, which may require an allosteric regulatory protein,Citation36 have been found: 1) a high-affinity transport mechanism with Michaelis constants Km < 1 µM iron; and 2) a low-affinity transport mechanism with Km > 5 µM iron.Citation38 The NTBI transport mechanisms in cell cultures depend on cell lines, culture pH and temperature, medium components such as calcium, other metals, and/or the small molecule iron carrier tropolone.Citation36,Citation37,Citation40 Chemically defined media without transferrin have been intensively used in CHO cell culture for production of therapeutic proteins in the biopharmaceutical industry, indicating that a NTBI transport system is present and playing a major role on iron uptake by CHO cells. Although controlling the iron form, level and delivery is critical for process optimization in chemically defined media,Citation31 the literature of NTBI transport mechanisms on CHO cell culture is sparse. Small molecule iron carriers, e.g., tropolone and selenium, have been used as alternatives to transferrin for effective iron update in low-iron chemically defined media for CHO cells.Citation41 Nonetheless, tropolone is patented and the ferric selenite preparation protocol is complex, with 14 days or longer required for maturation.Citation41 Therefore, Bai et al. considered that high-iron concentrations with sodium citrate in chemically defined media could be a better option for CHO cell culture manufacturing.Citation31 In addition, the cell growth and protein titer in some CHO cell lines depend on iron concentrations in chemically defined media and both titer and growth are linearly increased between 10 to 110 µM ().Citation12,Citation13,Citation31 As summarized in , the higher titer achieved in high-iron CHO cultures are mainly due to contributions by higher VCD, viability, specific productivity (qP), and higher consumption rates for nutrients such as glutamate and glutamine with a lower toxic ammonium production rate. However, the higher ROS formation observed in high-iron cultures may also negatively impact some critical product quality attributes (e.g., coloration, oxidation and acidic variants; ).
We hypothesize that CHO cell adaptation after long-term passages with more efficient NTBI uptake and lower ROS formation in low-iron chemically defined media would be the mechanism to achieve high peak VCD and titer in this study. The cell culture performance (e.g., peak VCD, viability, titer) for the long-term culture at P20 was independent of iron concentrations (), while the cell culture performance at P9 depended on iron concentrations (). Thus, we hypothesized that the iron uptake would be improved from the low affinity transport for manufacturing age culture (e.g., P9) to the high affinity transport for long-term culture (e.g., P20). Indeed, it has been reported that other mammalian cells can be adapted in serum-free media from 500 µM to 5–10 µM inorganic iron during a long-term culture.Citation42 After long-term culture adaptation in low-iron conditions, the cell line would have more efficient iron usage for cell growth and protein synthesis via an unknown NTBI uptake system.Citation42 Here, the older cells at P23 produced significantly less ROS than younger cells at P8 (), suggesting longer-term culture may have cells well adapted to the low-iron media, and thus reduced overall cellular stress. Catechin, an antioxidant capable of scavenging ROS, significantly improved the titer and peak VCD by about 30% for the same DG44 clone at manufacturing age during the fed-batch production of the Fc fusion protein (unpublished data). This further strengthens our hypothesis that reducing ROS would be involved in the mechanism for the titer and VCD improvement observed in the long-term culture, which differs from the mechanism for the high-iron process with increased ROS ().
Although iron is an essential element for CHO cells, it has not been reported consistently that high-iron concentrations will definitely improve titer. For example, Gawlizek et al.Citation43 found that there was no effect on cell growth and titer when increasing iron concentrations from ∼10 to ∼110 µM, while others reported ∼100 µM iron significantly increased cell growth and titer.Citation13,Citation31 Nonetheless, the cell ages and/or seed passages were not reported in all of those reports. Although different CHO cell lines and clones would have different sensitivities to low-iron media, it is possible that different seed passages in low-iron media might contribute to the conflicting results in literature.Citation31,Citation43 In fact, in our previous studyCitation13 only manufacturing age seeds with less than 13 passages from the vial thaw of MCB or MCB equivalent development cell bank (DCB) vials were used; thus, iron concentrations had a significant effect on peak VCD and titer in fed-batch culture. In this study, using the same clone for the production of the same therapeutic protein, it was found that, after long-term passaging, the peak VCD and titer were independent of iron concentrations (). However, the final drug substance color, due to tryptophan oxidation by high ROS,Citation13,Citation29 still depended on the iron concentrations regardless of cell culture passages ().
It should be noted that the cell line used for this study was adapted in low-iron chemically defined media for many passages before the MCB was manufactured (). MCB is the first GMP cell bank made for an industrial cell line. To ensure MCB vials are not depleted, a working cell bank (WCB) is usually made after 3–5 passage expansions from a MCB vial thaw. The WCB is the main GMP cell bank used for vial thaw and seed expansion in clinical and commercial manufacturing, while the MCB can be used for early clinical manufacturing before the WCB is made. The CHO DG44 cell line was created with a typical protocol, including sub cloning and gene amplification in hydrolysate media (Fig. 9). Then, a long-term adaptation in the low-iron chemically defined media for 11 passages was conducted before making the DCB (). MCB vials were made after 5 passages in the low-iron media from the DCB. Although the cell line was well-adapted for cell growth with a doubling time of 30–35 hours and 99% cell viability before the freezing of MCB, the peak VCD and titer during fed-batch cultures in the low-iron media were significantly lower than those in the high-iron media inoculated with the standard seeds at P9 from MCB (). However, after the long-term adaptation for 20 passages from MCB in the low-iron media, the titer was consistent at high levels regardless of iron concentrations (). Therefore, a much longer adaptation (i.e., passage 20) would be required in order to achieve similar high peak VCD and titer during fed-batch cultures in low-iron media compared to those cell cultures at manufacturing age seeds such as P9 in the high-iron media ().
Although there is concern about the effect of long-term stability on productivity for many CHO cell lines, we found that some CHO cell lines, such as the DG44 cell line in this study, require long-term adaptation (i.e., P20-P33 or 60–99 days from MCB vial thaw) for stable high productivity (). It was reported that the titer for production of a mAb was doubled after the CHO DG44 seed age prolonged from 21 to 95 days, although the seed was adapted to the chemically defined media for growth from day 0 at the beginning of passaging.Citation44 It is not unusual that unstable cell lines have been observed during different clinical stages because the lead clone may be selected based on short-term stability study data under a tight clinical and process development timeline. Therefore, to avoid cell line stability concerns, it is common to make the MCB and WCB with as few passages as possible. In this study, we found that the lead clone was quite stable with higher viability than other clones during the clone selection stage (data not shown). However, it was found that the cell line was adapted for high VCD and titer during fed-batch cultures after much longer term passaging at P20 from MCB vial thaw and then maintained at a similar high titer up to P33 (). It would not be practical to maintain seed cultures for more than 20 passages in manufacturing. Thus, it would be a good strategy to make the WCB after 12 passages from MCB vial thaw in the low-iron media for this particular case, rather than the usual protocol of 3–5 passages. In this way, the WCB made after long-term passaging can achieve a high titer in the low-ion production media with a standard 7–11 seed passages from WCB vial thaw for cell culture manufacturing. This strategy was effectively demonstrated in our shake flask run with vial thaw and seed expansion of 8 passages from different long-term culture DCBs ().
Here, we also reported that BGP and CHO cell ages have a significant effect on protein charge variant profiles (). Drug substances must be manufactured with comparable quality attributes at different clinical stages and throughout the entire commercial manufacturing life cycle.Citation24,Citation45,Citation46 The charge variants are usually critical quality attributes, which may affect recombinant protein drug pharmacology, efficacy and patient safety.Citation32,Citation33 Therefore, charge variant profiles of therapeutic proteins should be carefully monitored to ensure quality and comparability during different clinical and process development phases. BGP is a chemically defined medium component used as a phosphorus source. Phosphorus is an essential component for CHO cell culture that is usually added as sodium phosphate. However, high concentrations of phosphate may cause precipitation with other metals such as calcium, while higher concentrations of soluble phosphorus can be achieved using BGP rather than phosphate.Citation47 Therefore, BGP is a good phosphorus source for cell culture media if higher concentrations of phosphorus are needed. Although it is good for the long-term culture to achieve a high titer, we found that the long-term culture changed the charge variant profile for the prototype Process B during late-phase process development (). Here, it was found that both BGP and long-term passages significantly increased basic variants (), while downstream purification could not remove basic variants for this particular Fc fusion protein (). However, BGP did not affect peak VCD and titer in this particular case (data not shown). In early-phase process development, both phosphate and BGP were included in the media for Process A. Therefore, we successfully developed Process B to produce a high titer with acceptable drug substance color and charge variant profile by prolonging cell culture passages in the low-iron media and removing BGP in the production basal medium during the late-phase process development ( and ).
Medium optimization, one of the most important approaches for CHO cell culture process development, is very complicated. The functions and interactions of each component on upstream titer and quality attributes for different CHO cell lines and different therapeutic proteins are not fully understood. Although it is quite common to add back some components, such as amino acids, from depletion studies for titer improvement,Citation8,Citation48 many other components, such as BGP, are typically left at their baseline concentrations in platform media. In this study, we found that BGP in the basal media could be removed entirely without affecting titer, and iron could be reduced significantly from 110 to 10–20 µM for the long passage CHO cultures. In our previous study,Citation13 we found that copper and vitamin B12 concentrations could be reduced significantly without an impact on titer for the same cell line and protein. Therefore, increasing or reducing concentrations of other, less commonly considered medium components, could be good options for CHO cell process development, depending on cell lines, seed ages, proteins to be produced and other upstream conditions.
In conclusion, we identified two key process parameters (i.e., long-term seed culture and BGP) for late-phase process improvement. Although long-term culture generally causes cell line stability concerns, we found that the CHO DG44 cell line used in this study exhibited a significant titer improvement with increasing seed passage numbers even beyond typical manufacturing age. In addition, we found that BGP in the basal media affected basic variants of the Fc fusion protein. After these two changes were implemented (i.e., longer term adaptation of CHO cells in low-iron media and removing BGP from the basal media), the titer of the process was significantly improved while quality attributes of final formulated drug substance were maintained during late-phase process development for the production of the Fc fusion protein. In future studies, we will continue to probe the NTBI iron uptake mechanism at different CHO cell culture ages under different iron concentrations, and the mechanism by which BGP affects basic charge variants. In addition, we observed greater ROS production in high-iron media in this study, further advancing our knowledge of drug substance coloration and supporting our previous hypothesis on the increase of ROS production by high-iron conditions during cell culture.Citation13
Materials and methods
Cell line, media and cell culture processes
A CHO DG44 cell line was used for expression of a proprietary Fc fusion protein using a vector with a DHFR selectable marker ().
Figure 9. Cell line creation and Master Cell Bank (MCB) Genealogy: RCB: Research Cell Bank; DCB: Development Cell Bank; all seed cultures were split every three days per passage.
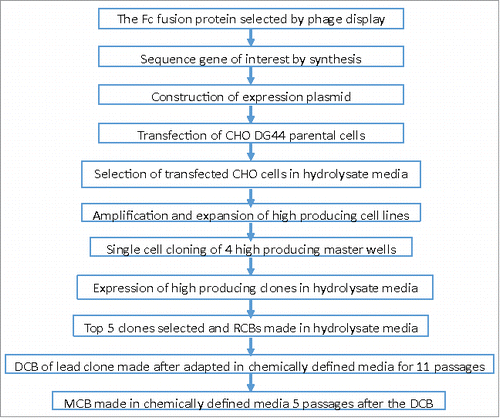
A proprietary chemically defined basal and feed media were used in this study unless otherwise specified. The low-iron media are defined as the media containing ≤ 20 µM iron, which did not cause coloration of the final formulated drug substance of the Fc fusion protein. The high-iron media are defined as the media containing ≥ 50 µM iron, which caused coloration of the Fc fusion protein. Seed media were prepared with the basal media plus 1 µM methotrexate.
Unless otherwise specified, all CHO seed cultures were started from the vial thaw of a frozen vial of MCB or MCB-equivalent DCBs made from the research cell bank using the same MCB banking protocol. All seed cultures were split every three days per passage (P) with an average doubling time of 30–35 hours.
The upstream process was run in lab, pilot and GMP manufacturing facilities, which were operated in fed-batch mode using the media and cell line described above. The inoculation density was 0.6 × 106 cells/mL. The fed-batch process was initially operated at 37°C and shifted to a lower temperature when VCD reached a proprietary level. Feeding was started when appropriate VCD was achieved and fed daily thereafter. Additional glucose was applied to maintain its concentration at a proprietary level. The bioreactor pH was set at pH 7.1 and controlled by addition of CO2 gas to decrease pH or addition of 1 M Na2CO3 base to increase pH as needed. The DO was maintained at 30% of air saturation by oxygen. For shake flask experiments, the cultures were maintained on a 25 mm shaker at 150 rpm in an incubator with 6% CO2.
In-process assays
VCD and cell viability were measured off-line using a Cedex automated cell counter (Innovatis AG). Culture samples were also analyzed off-line using a BioProfile 400 Analyzer to monitor pH, pCO2, pO2, glucose, glutamine, glutamate, lactate, and ammonium (Nova Biomedical Corporation). A Protein A HPLC method was used to measure protein titer. Titers were reported as normalized values, which are the actual values divided by the average of GMP 600-L run titers for Process A. Specific production or consumption rates of compound X (qX), e.g., specific production rate of the Fc fusion protein (qP), specific consumption rate of glutamic acid (qGlu), specific consumption rate of glutamine (qGln), specific production rate of ammonium (qNH4), from time1 (t1) to time2 (t2) were calculated using the following formula:where [X]t1 (or [X]t2) is the concentration of X at t1 (or t2), [IVCD]t1 (or [IVCD]t2) is the integral of viable cell density from t = 0 to t1 (or t2), and [X]feed is the concentration of X added to the flask in the feed from t1 to t2. Positive values of qX indicate net production, while negative values of qX indicate net consumption. The specific productivity qP was calculated from day0 (t1) to final harvest date (day12 or day14) (t2). Nutrient and metabolite consumption rates were evaluated from day 4 (the first measurement day) (t1) to final harvest date (day 12) (t2).
Harvest and purification process
Cell culture fluid was clarified using centrifugation, depth filtration followed by sterile filtration through 0.2 µm filters. Clarified harvest was processed through a three column process, consisting of MabSelect Protein A capture, viral inactivation, cation exchange chromatography, anion exchange chromatography, and ultrafiltration/diafiltration (UF/DF) steps to generate formulated bulk drug substance at a target concentration of 50 g/L. During the lab scale downstream processing for color evaluation, the depth filtration was not used for the harvest step and UF/DF was performed using 30 kDa spin columns (Cat# UFC 903008, EMD Millipore).
Color assay
For the visual assessment of color intensity and hues, 5 mL of drug substance was put in a 5-mL glass vial (Cat# WHEA223685, Wheaton). All pictures were taken under the same lighting condition.
Charge variant profile
Charge variants were assayed by imaged capillary isoelectric focusing, which was performed on a Protein Simple iCE3 instrument with an Alcott 720NV autosampler (San Jose, CA). Samples were mixed with appropriate pI markers, ampholytes, and urea and injected into a fluorocarbon-coated capillary cartridge. A high voltage was applied and the charged variants migrated to their respective pI. A UV camera captured the image at 280nM. The main peak was identified and the peaks that migrated into the acidic range and basic range were summed, quantitated, and reported as relative percent area.
Intracellular ROS assay
Intracellular ROS was measured with CellROX® Green Reagent (Thermo Fisher Scientific; C10444) following the manufacturer's instructions. In brief, cell culture supernatant samples were collected prior to the assay. Cell samples were then diluted in the supernatant samples resulting in 0.5 × 106 cells / ml. 1-mL of the sample was transferred to a non-adherent 6-well plate. After addition of the CellROX to a final concentration of 5 µM, the samples were incubated at 37°C with orbital shaking at 110 rpm for ∼5-10 min. The samples were then stored on ice and analyzed with Guava® easyCyte 8HT.
Abbreviations
BGP | = | β-glycerol phosphate |
CHO | = | Chinese hamster ovary |
DCB | = | development cell bank |
DOE | = | design-of-experiments |
GMP | = | good-manufacturing-practices |
IVCD | = | integral of viable cell density |
mAbs | = | monoclonal antibodies |
MCB | = | master cell bank |
NTBI | = | non-transferrin-bound iron |
P | = | passage |
qNH4 | = | specific production rates of ammonium |
qGln | = | specific consumption rates of glutamine |
qGlu | = | specific consumption rates of glutamic acid |
qP | = | specific productivity or specific production rates of the Fc fusion protein |
qX | = | specific production or consumption rates of compound X |
RCB | = | research cell bank |
ROS | = | reactive oxygen species |
UF/DF | = | ultrafiltration/diafiltration |
VCD | = | viable cell density |
WCB | = | working cell bank |
Disclosure of potential conflicts of interest
No potential conflicts of interest were disclosed.
Acknowledgment
The authors thank Henrik Andersen and Nripen Singh for their review and comments, Yuanzhen Wang, Bruce Eagan, You Li, Amanda Perlman, and Alison Bergeron for upstream lab work, Weixin Jin for downstream lab work, Chris House, Ves Lesins and pilot plant team for 50-L and 500-L runs, Amit Parikh, Lance Marquardt, Julie Erwin, Jess Nauman and GMP facility team for 600-L runs, and analytical teams in different sites for assay support.
References
- Walsh G. Biopharmaceutical benchmarks 2014. Nat Biotechnol. 2014;32(10):992–1000. doi:10.1038/nbt.3040. PMID:25299917.
- Rohrbach P, Broders O, Toleikis L, Dubel S. Therapeutic antibodies and antibody fusion proteins. Biotechnol Genet Eng Rev. 2003;20:137–63. PubMed. doi:10.1080/02648725.2003.10648041. PMID:14997850.
- Watier H, Reichert JM. Evolution of antibody therapeutics. In: Vaughn T, Osbourn J, Jallal B, editors. Protein Therapeutics. Weinheim, Germany: Wiley-VCH; 2017. p. 25–49.
- Scavone C, Sportiello L, Berrino L, Rossi F, Capuano A. Biosimilars in the European Union from comparability exercise to real world experience: what we achieved and what we still need to achieve. Pharmacol Res. 2017;119:265–71. doi:10.1016/j.phrs.2017.02.006. PMID:28214611.
- von Richter O, Skerjanec A, Afonso M, Sanguino Heinrich S, Poetzl J, Woehling H, Velinova M, Koch A, Kollins D, Macke L, et al. GP2015, a proposed etanercept biosimilar: Pharmacokinetic similarity to its reference product and comparison of its autoinjector device with prefilled syringes. Br J Clin Pharmacol. 2017;83(4):732–41. doi:10.1111/bcp.13170. PMID:27790726.
- Li F, Vijayasankaran N, Shen AY, Kiss R, Amanullah A. Cell culture processes for monoclonal antibody production. MAbs 2010;2(5):466–79. doi:10.4161/mabs.2.5.12720. PMID:20622510.
- Rader RA, Langer ES. 30 years of upstream productivity improvements. BioProcess Int. 2015;13(2):10–14.
- Huang YM, Hu W, Rustandi E, Chang K, Yusuf-Makagiansar H, Ryll T. Maximizing productivity of CHO cell-based fed-batch culture using chemically defined media conditions and typical manufacturing equipment. Biotechnol Prog. 2010 Sep-Oct;26(5):1400–10. doi:10.1002/btpr.436. PMID:20945494.
- Takagi Y, Kikuchi T, Wada R, Omasa T. The enhancement of antibody concentration and achievement of high cell density CHO cell cultivation by adding nucleoside. Cytotechnology. 2017;69(3):511–21. doi:10.1007/s10616-017-0066-7. PMID:28251404.
- Derfus GE, Dizon-Maspat J, Broddrick JT, Velayo AC, Toschi JD, Santuray RT, Hsu SK, Winter CM, Krishnan R, Amanullah A. Red colored IgG4 caused by vitamin B12 from cell culture media combined with disulfide reduction at harvest. MAbs. 2014;6(3):679–88. doi:10.4161/mabs.28257. PMID:24552690.
- Prentice KM, Gillespie R, Lewis N, Fujimori K, McCoy R, Bach J, Connell-Crowley L, Eakin CM. Hydroxocobalamin association during cell culture results in pink therapeutic proteins. MAbs. 2013;5(6):974–81. doi:10.4161/mabs.25921. PMID:23924851.
- Vijayasankaran N, Varma S, Yang Y, Mun M, Arevalo S, Gawlitzek M, Swartz T, Lim A, Li F, Zhang B, et al. Effect of cell culture medium components on color of formulated monoclonal antibody drug substance. Biotechnol Prog. 2013;29(5):1270–7. doi:10.1002/btpr.1772. PMID:23804462.
- Xu J, Jin M, Song H, Huang C, Xu X, Tian J, et al. Brown drug substance color investigation in cell culture manufacturing using chemically defined media: A case study. Process Biochem. 2014;49(1):130–9. doi:10.1016/j.procbio.2013.10.015.
- Chumsae C, Hossler P, Raharimampionona H, Zhou Y, McDermott S, Racicot C, Radziejewski C, Zhou ZS. When Good Intentions Go Awry: Modification of a Recombinant Monoclonal Antibody in Chemically Defined Cell Culture by Xylosone, an Oxidative Product of Ascorbic Acid. Anal Chem. 2015;87(15):7529–34. doi:10.1021/acs.analchem.5b00801. PMID:26151084.
- Hossler P, Wang M, McDermott S, Racicot C, Chemfe K, Zhang Y, Chumsae C, Manuilov A. Cell culture media supplementation of bioflavonoids for the targeted reduction of acidic species charge variants on recombinant therapeutic proteins. Biotechnol Prog. 2015 Jul-Aug;31(4):1039–52. doi:10.1002/btpr.2095. PMID:25920009.
- Kaschak T, Boyd D, Lu F, Derfus G, Kluck B, Nogal B, Emery C, Summers C, Zheng K, Bayer R, et al. Characterization of the basic charge variants of a human IgG1: Effect of copper concentration in cell culture media. MAbs. 2011;3(6):577–83. doi:10.4161/mabs.3.6.17959. PMID:22123059
- Luo J, Vijayasankaran N, Autsen J, Santuray R, Hudson T, Amanullah A, Li F. Comparative metabolite analysis to understand lactate metabolism shift in Chinese hamster ovary cell culture process. Biotechnol Bioeng. 2012;109(1):146–56. doi:10.1002/bit.23291. PMID:21964570.
- Pacis E, Yu M, Autsen J, Bayer R, Li F. Effects of cell culture conditions on antibody N-linked glycosylation–what affects high mannose 5 glycoform. Biotechnol Bioeng. 2011;108(10):2348–58. doi:10.1002/bit.23200. PMID:21557201.
- Sha S, Agarabi C, Brorson K, Lee DY, Yoon S. N-Glycosylation Design and Control of Therapeutic Monoclonal Antibodies. Trends Biotechnol. 2016;34(10):835–46. doi:10.1016/j.tibtech.2016.02.013. PMID:27016033.
- Huang CJ, Lin H, Yang JX. A robust method for increasing Fc glycan high mannose level of recombinant antibodies. Biotechnol Bioeng. 2015;112(6):1200–9. doi:10.1002/bit.25534. PMID:25565276.
- Louie S, Haley B, Marshall B, Heidersbach A, Yim M, Brozynski M, Tang D, Lam C, Petryniak B, Shaw D, et al. FX knockout CHO hosts can express desired ratios of fucosylated or afucosylated antibodies with high titers and comparable product quality. Biotechnol Bioeng. 2017;114(3):632–44. doi:10.1002/bit.26188. PMID:27666939.
- Torkashvand F, Vaziri B. Main Quality Attributes of Monoclonal Antibodies and Effect of Cell Culture Components. Iran Biomed J. 2017;21(3):131–41. PubMed doi:10.18869/acadpub.ibj.21.3.131. PMID:28176518.
- Wurm FM. CHO Quasispecies—Implications for Manufacturing Processes. Processes. 2013;1:296–311. doi:10.3390/pr1030296.
- Xu S, Hoshan L, Chen H. Improving lactate metabolism in an intensified CHO culture process: productivity and product quality considerations. Bioprocess and Biosyst Eng. 2016;39:1689–702. doi:10.1007/s00449-016-1644-3.
- Borys MC, Dalal NG, Abu-Absi NR, Khattak SF, Jing Y, Xing Z, Li ZJ. Effects of culture conditions on N-glycolylneuraminic acid (Neu5Gc) content of a recombinant fusion protein produced in CHO cells. Biotechnol Bioeng. 2010 Apr 15;105(6):1048–57. PMID:20039310.
- Zhuang C, Zheng C, Chen Y, Huang Z, Wang Y, Fu Q, Zeng C, Wu T, Yang L, Qi N. Different fermentation processes produced variants of an anti-CD52 monoclonal antibody that have divergent in vitro and in vivo characteristics. Appl Microbiol Biotechnol. 2017 Aug;101(15):5997–6006. doi:10.1007/s00253-017-8312-7. PMID:28512676.
- Aghamohseni H, Spearman M, Ohadi K, Braasch K, Moo-Young M, Butler M, Budman HM. A semi-empirical glycosylation model of a camelid monoclonal antibody under hypothermia cell culture conditions. J Ind Microbiol Biotechnol. 2017 Jul;44(7):1005–20. doi:10.1007/s10295-017-1926-z. PMID:28285402.
- Kishishita S, Nishikawa T, Shinoda Y, Nagashima H, Okamoto H, Takuma S, Aoyagi H. Effect of temperature shift on levels of acidic charge variants in IgG monoclonal antibodies in Chinese hamster ovary cell culture. J Biosci Bioeng. 2015 Jun;119(6):700–5. doi:10.1016/j.jbiosc.2014.10.028. PMID:25466646.
- Song H, Xu J, Jin M, Huang C, Bongers J, Bai H, Wu W, Ludwig R, Li Z, Tao L, et al. Investigation of Color in a Fusion Protein Using Advanced Analytical Techniques: Delineating Contributions from Oxidation Products and Process Related Impurities. Pharm Res. 2016;33:932–41. doi:10.1007/s11095-015-1839-3. PMID:26658915.
- Du C, Martin R, Huang Y, Borwankar A, Tan Z, West J, Singh N, Borys M, Ghose S, Ludwig R, et al. Vitamin B12 association with mAbs: Mechanism and potential mitigation strategies. Biotechnol Bioeng. 2018; In press:1–10. doi:10.1002/bit.26511.
- Bai Y, Wu C, Zhao J, Liu YH, Ding W, Ling WLW. Role of iron and sodium citrate in animal protein-free CHO cell culture medium on cell growth and monoclonal antibody production. Biotechnol Prog. 2011;27(1):209–19. doi:10.1002/btpr.513. PMID:21312368.
- Du Y, Walsh A, Ehrick R, Xu W, May K, Liu H. Chromatographic analysis of the acidic and basic species of recombinant monoclonal antibodies. MAbs. 2012;4(5):578–85. doi:10.4161/mabs.21328. PMID:22820257.
- Li B, Tesar D, Boswell CA, Cahaya HS, Wong A, Zhang J, Meng YG, Eigenbrot C, Pantua H, Diao J, et al. Framework selection can influence pharmacokinetics of a humanized therapeutic antibody through differences in molecule charge. MAbs. 2014;6(5):1255–64. doi:10.4161/mabs.29809. PMID:25517310.
- Aisen P, Enns C, Wessling-Resnick M. Chemistry and biology of eukaryotic iron metabolism. Int J Biochem Cell Biol. 2001;33(10):940–59. doi:10.1016/S1357-2725(01)00063-2. PMID:11470229.
- Hentze MW, Muckenthaler MU, Andrews NC. Balancing acts: Molecular control of mammalian iron metabolism. Cell. 2004;117(3):285–97. doi:10.1016/S0092-8674(04)00343-5. PMID:15109490.
- Basset P, Zwiller J. Iron-induced L1210 Cell Growth: Evidence of a Transferrin-independent Iron Transport. Cancer Res 1986;46:1644–7. PMID:3948154.
- Metcalfe H, Field, R. P., Froud, S. J. The use of 2-hydroxy-2, 4, 6- cycloheptarin-1-one (tropolone) as a replacement for transferrin. In: Spier RE GJ, Berthold W, editors. Animal Cell Technology: Products of Today, Prospects of Tomorrow. Oxford, UK: Butterworth- Heinemann; 1994. p. 88–90.
- Musílková J, Kovář J. Additive stimulatory effect of extracellular calcium and potassium on non-transferrin ferric iron uptake by HeLa and K562 cells. Biochim Biophys Acta. 2001;1514(1):117–26. doi:10.1016/S0005-2736(01)00367-4. PMID:11513809.
- Sturrock A, Alexander J, Lamb J, Craven CM, Kaplan J. Characterization of a transferrin-independent uptake system for iron in HeLa cells. J Biol Chem. 1990;265(6):3139–45. PMID:2105943.
- Inman RS, Wessling-Resnick M. Characterization of transferrin-independent iron transport in K562 cells. Unique properties provide evidence for multiple pathways of iron uptake. J Biol Chem. 1993;268(12):8521–8. PMID:8473296.
- Zhang J, Robinson D, Salmon P. A novel function for selenium in biological system: selenite as a highly effective iron carrier for Chinese hamster ovary cell growth and monoclonal antibody production. Biotechnol Bioeng. 2006;95(6):1188–97. doi:10.1002/bit.21081. PMID:16937407.
- Neumannova V, Richardson DR, Kriegerbeckova K, Kovar J. Growth of human tumor cell lines in transferrin-free, low-iron medium. In Vitro Cell Dev Biol Anim. 1995;31(8):625–32. doi:10.1007/BF02634316. PMID:8528518.
- Gawlitzek M, Estacio M, Fürch T, Kiss R. Identification of cell culture conditions to control N-glycosylation site-occupancy of recombinant glycoproteins expressed in CHO cells. Biotech Bioeng. 2009;103(6):1164–75. doi:10.1002/bit.22348.
- Beckmann TF, Kramer O, Klausing S, Heinrich C, Thute T, Buntemeyer H, Hoffrogge R, Noll T. Effects of high passage cultivation on CHO cells: a global analysis. Appl Microbiol Biotechnol. 2012 May;94(3):659–71. doi:10.1007/s00253-011-3806-1. PMID:22331235.
- Hassett B, Singh, E, Mahgoub, E, O'Brien, J, Vicik, SM, Fitzpatrick, B. Manufacturing history of etanercept (Enbrel): Consistency of product quality through major process revisions. MAbs. 2018;10(1):159–65. doi:10.1080/19420862.2017.1388483.
- Lee SY, Kwon YB, Cho JM, Park KH, Chang SJ, Kim DI. Effect of process change from perfusion to fed-batch on product comparability for biosimilar monoclonal antibody. Process Biochem. 2012;47(9):1411–8. doi:10.1016/j.procbio.2012.05.017.
- Hanning RM, Michell, MK, Atkinson, SA. In Vitro Solubility of Calcium Glycerophosphate Versus Conventional Mineral Salts in Pediatric Parenteral Nutrition Solutions. J Pediatr Gastroenterol and Nutr. 1989;9:67–72. doi:10.1097/00005176-198909010-00013.. PMID:2506324.
- Xing Z, Kenty B, Koyrakh I, Borys M, Pan SH, Li ZJ. Optimizing amino acid composition of CHO cell culture media for a fusion protein production. Process Biochem. 2011;46(7):1423–9. doi:10.1016/j.procbio.2011.03.014.