ABSTRACT
A mixture comprising horseradish peroxidase (HRP), glucose oxidase, and glucose was used to cross-link bovine gelatin via dityrosine formation. Suitable HRP, glucose oxidase, glucose addition levels, and reaction time were studied with single-factor trials, and selected as 100 U, 1 U, 0.025 mmol/g protein, and 3 h, respectively. Electrophoretic and circular dichroism analyses showed that the cross-linked gelatin contained high levels of polymerized proteins, had a typical triple helix structure at 5°C, but a more ordered secondary structure at 40°C. In comparison with bovine gelatin, the cross-linked gelatin had better colloidal stabilization in dispersion through the formation of aggregates of smaller hydrodynamic radius (73.3 versus 97.2 nm) and more negative zeta-potential (–8.15 versus –5.88 mV), showed enhanced thermal stability with a higher decomposition temperature (323.7°C versus 320.8°C), but less mass loss (50.10% versus 50.90%) and formed gels with shorter gelation time (14.1 versus 34.1 min) and stronger gel strength (increased by 12–14%).
RESUMEN
Se utilizó una mezcla compuesta de peroxidasa de rábano picante (HRP), glucosa oxidasa y glucosa para entrecruzar gelatina bovina mediante la formación de tirosina. Se estudiaron los HRP, la glucosa oxidasa, los niveles de adición de glucosa y el tiempo de reacción más adecuados con ensayos de un solo factor y seleccionados como 100 U, 1 U, 0,025 mmol/g de proteína y 3 h, respectivamente. Los análisis electroforéticos y de dicroísmo circular mostraron que la gelatina entrecruzada contenía muchas proteínas polimerizadas, tenía una estructura típica de triple hélice a 5°C aunque una estructura secundaria más ordenada a 40°C. En comparación con la gelatina bovina, la gelatina entrecruzada tenía una mayor estabilización coloidal en dispersión mediante la formación de agregados con radios hidrodinámicos más pequeños (73,3 frente a 97,2 nm) y más potencial zeta negativo (–8,15 frente a –5,88 mV), mostró una estabilidad termal mejorada con una mayor temperatura de descomposición (323,7°C frente a 320,8°C) aunque menos pérdida de masa (50,10% frente a 50,90%), podía formar geles con un menor tiempo de gelificación (14,1 frente a 34,1 min) y una mayor resistencia del gel (mejorada de 12–14%).
Introduction
Gelatin is derived from native collagen from the skin, bone, and connective tissue of animals (e.g. bovine and porcine) and consists of a heterogeneous mixture of water-soluble proteins of varying molecular weight (Derkatch, Petrova, Izmailova, & Tarasevitch, Citation1999). As one of the most commonly used biopolymers, gelatin has some important and special properties and is widely used in many fields in the form of additives, packaging materials, and other ingredients. Gelatin is capable of increasing the viscosity of its surrounding aqueous system and forming aqueous gels, and is thus widely used as an additive in processed foods such as confectionery, jellied meats, and chilled dairy products (Gilsenan & Ross-Murphy, Citation2000). At temperatures above 40°C, gelatin solution is in the so-called sol state, and is transformed to gel when cooled to room temperature at sufficiently high concentration (Gómez-Guillén et al., Citation2002). The thermo-reversibility of this process gives gelatin gels a unique attribute known as ‘melt-in-the-mouth’. The sol–gel transformation of gelatin arises from a conformational disorder involving the transition of gelatin chains, which form thermo-reversible three-dimensional networks by assembling triple helices in the junction zones stabilized by hydrogen bonds (Bigi, Cojazzi, Panzavolta, Roveria, & Rubini, Citation2002). Gelatin has good colloidal stabilization and can provide protection to dispersions such as gold colloid (Zhang, Gu, Zheng, & Zhu, Citation2009) and emulsions (Olijve, Mori, & Toda, Citation2001). As a biomaterial, the thermal stability of gelatin is also a key factor in practical applications, such as the preparation of edible films (Bigi et al., Citation2002; Sobral, Menegalli, Hubinger, & Roques, Citation2001). The properties of gelatin are markedly influenced by both amino acid composition and – in particular – the distribution of molecular weight (Muyonga, Cole, & Duodu, Citation2004). Extraction of collagen at high temperatures reduces the mechanical properties of gelatin due to excessive hydrolysis (Schrieber & Gareis, Citation2007), which is considered to limit its applications. For example, gelatin films are usually recommended as having good mechanical properties (Cao, Fu, & He, Citation2007).
In recent decades, both chemical and physical treatments have been applied to gelatin to modify its polymer network and properties via cross-linking. Chemical agents used for gelatin cross-linking include glutaraldehyde (Matsuda, Iwata, Se, & Ikada, Citation1999) and ferulic and tannin acids (Cao et al., Citation2007), resulting in gelatin films with increased mechanical strength. Physical treatments include γ-ray (Bessho, Furuta, Kojima, Okuda, & Hara, Citation2005) and ultraviolet irradiation (Bhat & Karim, Citation2009). Higher-dose γ-ray irradiation results in stiffer and more compact gelatin gels with lower specific water content, while ultraviolet irradiation confers enhanced strength on fish gelatin (Bessho et al., Citation2005; Bhat & Karim, Citation2009). In addition, a natural cross-linker (genipin) and an enzyme transglutaminase (TGase) have also been investigated for their abilities to cross-link gelatin (Bigi et al., Citation2002; De Carvalho & Grosso, Citation2004; Jongjareonrak, Benjakul, Visessanguan, & Tanaka, Citation2006). Treatment of gelatin with genipin decreases deformability, resulting in gelatin film with better thermal stability (Bigi et al., Citation2002). TGase treatment of two gelatin products resulted in an enhanced melting point for gelatin films and enhanced the strength of gelatin gels (De Carvalho & Grosso, Citation2004; Jongjareonrak et al., Citation2006).
Recently, a mixture comprising two oxidases (horseradish peroxidase and glucose oxidase) and glucose was demonstrated to enhance the cross-linking of caseinate (Chang & Zhao, Citation2012) and soy protein isolate (SPI) (Jiang & Zhao, Citation2014). In this reaction, glucose oxidase catalyses glucose oxidation to produce H2O2 (Vemulapalli & Hoseney, Citation1998), which is subsequently utilized by horseradish peroxidase (HRP) to cross-link protein substrates by dityrosine formation (Stahmann, Spencer, & Honold, Citation1977). The modified caseinate has both higher apparent viscosity and enhanced elastic and viscous modulus (Chang & Zhao, Citation2012), while the modified SPI shows shorter gelation time and lower gelation point temperature (Jiang & Zhao, Citation2014). Cross-linking gelatin by this mixture has not been studied to date, and potential property changes in cross-linked gelatin should be clarified to demonstrate its practical application in the food industry.
In the present study, a mixture of HRP, glucose oxidase, and glucose was applied to cross-link commercial bovine gelatin. Cross-linking conditions suitable for the gelatin were briefly investigated via single-factor trials. The colloidal, thermal, and gelling properties of the cross-linked gelatin were evaluated and compared to those of bovine gelatin. The objective of this study is to assess whether cross-linking is helpful in regard to improving the properties and enlarging the potential application of bovine gelatin.
Materials and methods
Material and chemicals
Commercial type B bovine gelatin with a protein content of 958 g/kg (dry basis) was purchased from Shandong Yixin Biological Technology Co., Ltd. (Binzhou, China). Glucose oxidase (E.C. 1.1.3.4, Type X-S, optimal pH 5.2, 130 kU/g) from Aspergillus niger and horseradish peroxidase (E.C. 1.11.1.7, optimal pH 7.0, 20 kU/g) were obtained from Sigma-Aldrich Co. (St. Louis, MO, USA) and Shanghai Guoyuan Biotech, Inc. (Shanghai, China). Protein markers with molecular weight 43–200 kDa were obtained from Beijing Solarbio & Technology Co., Ltd. (Beijing, China). Other chemicals used were of analytical grade. Bi-distilled water was used to prepare the buffers and solutions.
Modification of bovine gelatin by HRP, glucose oxidase, and glucose
Aqueous gelatin solution (protein concentration 55 g/kg) was prepared by soaking the gelatin in water for 15 min in a triangular flask covered with plastic film and then by heating at 60°C for 30 min. Gelatin solution (pH 6.1) was cooled to room temperature (~20°C), and the pH adjusted to 7.0 with 0.2 mol/L NaOH solution. The HRP, glucose oxidase, and glucose solutions were prepared by dissolving in water immediately prior to use and were then added to the gelatin solution, resulting in a final protein concentration of 50 g/kg for the reaction system. Gelatin cross-linking was carried out at 37°C with continuous agitation of the reaction system. In single-factor trials, the relative dityrosine content of cross-linked gelatin was analysed and used as an indicator of gelatin cross-linking to select suitable cross-linking conditions. The levels of HRP, glucose oxidase, and glucose in the reaction system were 50–200 U, 0.5–6 U, and 0.005–0.075 mmol/g protein, respectively, while the reaction time varied from 1 to 4 h. Following the reaction, the system was heated at 85°C for 10 min to inactivate the enzymes, according to a previous study (Chang & Zhao, Citation2012), cooled to room temperature, and then protein concentration reduced to 0.5 g/kg with a phosphate buffer (0.2 mol/L, pH 7.4). The diluted solutions were investigated for relative fluorescence intensity (i.e. relative dityrosine content) according to Davies, Delsignore, & Lin (Citation1987), using a Hitachi F-4500 fluorescence spectrometer (Tokyo, Japan). A slit width of 0.5 nm and wavelengths of 320 nm (excitation) and 410 nm (emission) were used.
Based on these results from the single-factor trials, suitable cross-linking conditions were selected to ensure higher relative dityrosine content of the cross-linked gelatin. The cross-linked gelatin solution was then lyophilized at an initial temperature of –24°C and pressure of 10 Pa. The powder product (cross-linked gelatin) thus obtained was dried at 60°C for 12 h to ensure complete drying but not excessive protein decomposition, and then ground to fine particles (diameter <150 μm) capable of passing through a standard sieve (Shangyu Huafeng Hardware Instrument Co., Ltd., Shaoxing, China). About 250 g of cross-linked gelatin was obtained to evaluate its properties. The original bovine gelatin was used as a control during the evaluation.
Protein concentrations of the samples were analysed using the Kjeldahl method (AOAC, Citation2005). A conversion factor of 5.55 was used in calculations (Mohtar, Perera, Quek, & Hemar, Citation2013).
Electrophoretic analysis
Sodium dodecyl sulphate-polyacrylamide gel electrophoresis (SDS-PAGE) analysis was performed according to the method of Laemmli (Citation1970). Sample solutions (5 g/L) were equally mixed with sample buffer (pH 6.8) containing 0.1 mol/L Tris-HCl, 0.2 mol/L β-mercaptoethanol, 2 g/L bromophenol blue, 40 g/L SDS, and 200 g/L glycerol. The mixtures were boiled for 5 min. Protein samples (~25 μg) were loaded onto polyacrylamide gels, comprising stacking (40 g/L) and resolving (70 g/L) gels, with a DYY-8 C electrophoresis apparatus (Beijing Liuyi Instrument Factory, Beijing, China). Electrophoresis was performed at 80 V for 40 min in the stacking gels and 100 V for 90 min in the resolving gels. After electrophoresis, the gels were stained with Coomassie brilliant blue R250 and de-stained with 200 mL/L methanol and 100 mL/L acetic acid. Standard protein markers (43–200 kDa) were also used in the analysis.
Circular dichroism measurement
Sample solutions (0.1 g/L) were prepared with a phosphate buffer (0.01 mol/L, pH 7.0). Far-UV circular dichroism (CD) spectra of the sample solutions were assessed by a spectropolarimeter (Type J-815, JASCO International Co., Ltd., Tokyo, Japan) using a 1 mm path length quartz cuvette at 5 and 40°C, according to Gómez-Guillén et al. (Citation2002). The spectra were recorded from 190 to 240 nm with a scanning rate of 100 nm/min, a data interval of 0.2 nm, a band width of 1.0 nm, and a response time of 0.125 s, to determine the molar ellipticity (θ) (deg cm2/dmol) of the samples.
Measurement of hydrodynamic radii and zeta-potential
The samples were dispersed in a phosphate buffer (0.01 mol/L, pH 7.0) at a protein concentration of 1 g/L. Hydrodynamic radii and zeta-potential were measured using a Zetasizer Nano-ZS90 (Malvern Instruments, Westborough, MA, USA) at room temperature. The dynamic light scattering (DLS) technique was used for the measurement of hydrodynamic radii, while a voltage of 80 V was applied for the measurement of zeta-potential. The hydrodynamic radii and zeta-potential were estimated, respectively, by the Stokes–Einstein and Henry equations (Lam & Nickerson, Citation2014).
Thermogravimetric analysis
Thermogravimetric (TG) analysis of the samples was carried out using a TG analyser (SDT Q600, TA Instruments Inc., New Castle, DE, USA) as previously reported (Luo & Zhao, Citation2014), with slight modification. Dried samples were maintained in a desiccator at room temperature with saturated NaCl solution (relative humidity ~75.5%) for 72 h, to ensure that the samples adsorbed water completely. Samples (2 mg) were then added to the pans and heated from 20 to 450°C at a rate of 10°C/min under air atmosphere (100 mL/min). A blank pan was previously analysed as the baseline for calibration. Two temperature regions (region I, 20–105ºC; and region II, 105–450ºC), responsible for the maximum water loss and degradation rate (Tmax) of the samples, respectively, as well as mass losses at the two temperature regions, were determined from the TG and derivative TG (DTG) curves.
Assay of gelling properties
Gel strength was assessed according to Gómez-Guillén et al. (Citation2002), with slight modifications. The bovine and cross-linked gelatin were dissolved in water at 60°C at three concentrations (25.0, 50.0, and 66.7 g/L; pH 7.0), according to the above method. The solutions were stirred to complete solubilization, poured into containers (diameter 3.2 cm, height 5.0 cm), and refrigerated at 4°C for 17 h prior to measurement. Gel strength was determined at 8–10°C using a Stable Micro Systems Texturometer (Model TA-XT2i, Stable Micro Systems Ltd., Surrey, UK) with a load cell of 5 kg, a cross-head speed of 1 mm/s, and a diameter flat-faced cylindrical plunger of 1.27 cm. The maximum force (N) of the prepared gels (diameter 3.2 cm, height 1.7 cm) was recorded when the plunger penetrated 4 mm into the gel, and is reported as gel strength.
Time sweep was used to detect gelation time, using a gelatin concentration of 66.7 g/L as described above. The analysis was carried out using a Bohlin Gemini II Rheometer (Malvern Instruments, Malvern, UK) fitted with a cup (inner diameter 37 mm) and bob geometry (diameter 34 mm; length 41 mm) as reported by Jiang & Zhao (Citation2014), with minor modifications. The gap between the cup and bob was 1.0 mm. Sample solutions were placed in the cup and initially maintained at 60°C for equilibration. Time sweep was performed with a frequency of 1 Hz at 25ºC, and measurements were taken every 30 s for 1 h. Elastic modulus (G′) values of the sample solutions were recorded during gel formation. Gelation time was defined by a G′ value higher than 10 Pa (Jiang & Zhao, Citation2014).
Statistical analysis
All experiments and analyses were performed in triplicate, and the obtained data were expressed as means ± standard deviation. One-way ANOVA was used to evaluate the statistical significance of the differences among the mean values of multiple groups at the significance level of p < 0.05, using SPSS 16.0 software (SPSS Inc., Chicago, IL, USA).
Results and discussion
Modification conditions for bovine gelatin
Glucose, glucose oxidase, and HRP addition levels, and reaction time for gelatin cross-linking were the parameters investigated in regard to the preparation of cross-linked gelatin. Dityrosine formed during protein cross-linking showed characteristic fluorescence absorption, and therefore change in fluorescence intensity was used to reflect the relative dityrosine content of the cross-linked gelatin in this study. All four parameters were found to have impacts on gelatin cross-linking, as the relative fluorescence intensity (RFI) (i.e. relative dityrosine content) of the cross-linked gelatin was clearly impacted by all four parameters (). The results in – demonstrate that additional levels of glucose, glucose oxidase, and HRP as well as prolonged reaction time did not always yield cross-linked gelatin with the highest relative dityrosine content. To achieve enhanced gelatin cross-linking, the appropriate glucose and glucose oxidase levels were found to be 0.025 mmol and 1 U/g protein, respectively, with a reaction time of 3 h. At HRP levels of 100 and 150 U/g protein, the cross-linked gelatin had respective RFI values of (3475 ± 12) and (3594 ± 20) (). That is, increasing the HRP level by 50% yielded cross-linked gelatin with an increased relative dityrosine content of only 3.4%. However, a HRP level of 50 U/g protein resulted in an decrease of about 7% in relative dityrosine content (p < 0.05). A HRP level of 100 U/g protein was therefore selected. Under these conditions, cross-linked gelatin with RFI value (3463 ± 17) was thus prepared in bulk in this study, and used in subsequent evaluation.
Figure 1. Impacts of additive levels of glucose (a), glucose oxidase (b), and HRP (c) and reaction time (d) on relative fluorescence intensity (RFI) of cross-linked gelatin. Different lower case letters above columns indicate significant differences between mean values (p < 0.05).
Figura 1. Impactos de la glucosa (a), la glucosa oxidasa (b), HRP (c) los niveles de adición y el tiempo de reacción (d) en la intensidad fluorescente relativa (RFI) de la gelatina entrecruzada. Las diferentes letras minúsculas encima de las columnas indican diferencias significativas entre los valores promedio (p < 0,05).
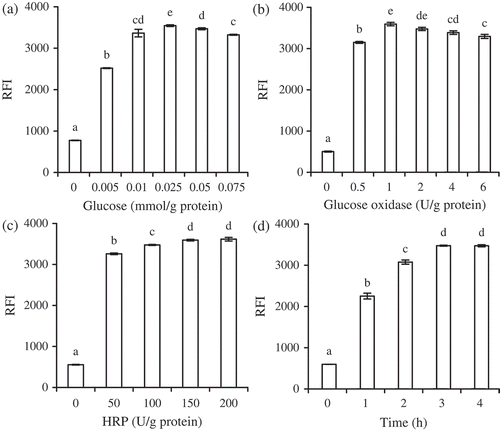
In two previous studies, appropriate HRP, glucose oxidase, and glucose levels for caseinate cross-linking were found to be 200 U, 6 U, and 0.05 mmol/g protein (Chang & Zhao, Citation2012), respectively, while levels for SPI cross-linking were 200 U, 4 U, and 0.1 mmol/g protein (Jiang & Zhao, Citation2014), respectively. These two studies also used a reaction time of 3 h. Compared with values used in these two studies, in the present study lower HRP, glucose oxidase, and glucose levels but the same reaction time were used for gelatin cross-linking. Gelatin usually has a tyrosine content of about 5.0 g/kg protein (De Carvalho & Grosso, Citation2004), while for caseinate and SPI reported levels are about 63.0 and 33.4 g/kg protein (Belitz, Grosch, & Schieberle, Citation2009), respectively. It is to be expected that gelatin has inferior ability to both caseinate and SPI in carrying out this cross-linking. That is, less HRP, glucose oxidase, and glucose would be needed in gelatin cross-linking.
Compositional and structural changes of cross-linked gelatin
The electrophoretograms obtained for bovine gelatin and cross-linked gelatin are shown in ; the electrophoretic results reveal a difference in protein composition between the two gelatins. Cross-linked gelatin had a higher protein fraction with greater molecular weight than the bovine gelatin (Lane 1 versus Lane 2), because the band at the top of Lane 1 was observed to be deeper than its counterpart at the top of Lane 2 (indicated by arrow). This result shows that the cross-linked gelatin was a cross-linked gelatin product, as a result of dityrosine formation in gelatin molecules. Electrophoretic analysis was used (Chang & Zhao, Citation2012; Jiang & Zhao, Citation2014) to prove that this mixture induces cross-linking of caseinate and SPI. The present SDS-PAGE result demonstrates that this mixture can also induce gelatin cross-linking.
Figure 2. Electrophoretic profiles of cross-linked gelatin and bovine gelatin. Lane M, standard protein markers; lane 1, cross-linked gelatin; lane 2, bovine gelatin.
Figura 2. Perfiles electroforéticos de gelatina entrecruzada y gelatina bovina. Línea M, marcadores de proteína estándar; Línea 1, gelatina entrecruzada; Línea 2, gelatina bovina.
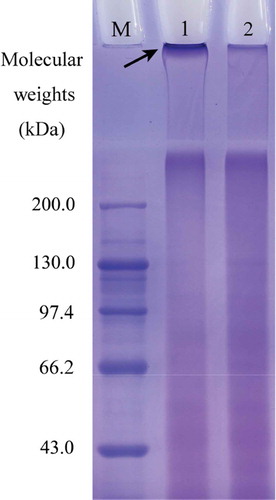
CD analysis is widely used to reflect the secondary structure of proteins. CD spectra of both cross-linked and bovine gelatin were assessed at two temperatures, and are presented in . Both gelatins showed the typical triple-helix structure because they had positive peaks around 225 nm at 5°C – a typical feature of the triple helix of collagen and collagen-like products (Gómez-Guillén et al., Citation2002). However, based on CD spectra at 40ºC, differences are noted in the secondary structure of the two gelatins. At this high temperature, rather than the presence of triple-helix structures, the gelatins remained in random coil conformation as the positive peaks around 225 nm disappeared (Wu et al., Citation2007). The cross-linked gelatin at 40ºC exhibited weaker negative absorption around 200 nm than the bovine gelatin, which is a typical indication of disordered secondary structure (Silverstein, Webster, & Kiemle, Citation2005). This indicates that the cross-linked gelatin was less disordered than the bovine gelatin: that is, the former was more ordered in regard to secondary structure than latter. This is not an unexpected result. It is speculated that dityrosine formation in the cross-linked gelatin yielded more covalent bonds in the molecules, thus conferring an ordered secondary structure.
Colloidal properties of cross-linked gelatin
The hydrodynamic radii of the gelatins in the dispersions were measured by DLS, and the results are presented in . In total, the cross-linked gelatin was observed to form smaller aggregates than the bovine gelatin (p < 0.05). The average hydrodynamic radius of the cross-linked gelatin was calculated at 73.3 ± 7.6 nm, while that of the bovine gelatin was 97.2 ± 5.3 nm. This finding reveals that the cross-linked gelatin could form smaller aggregates with good colloidal stabilization, as protein aggregates of smaller size should be more resistant to aggregation or coagulation. At the same time, the zeta-potential value of the cross-linked gelatin ranged from –7.85 to –8.39 mV (–8.15 ± 0.22 mV), while that of the bovine gelatin ranged from –5.70 to –6.00 mV (–5.88 ± 0.13 mV). These results demonstrate again that cross-linked gelatin provides better colloid protection than bovine gelatin against sedimentation on account of its markedly negative zeta-potential value (p < 0.05).
Figure 4. Distribution of hydrodynamic radii of cross-linked gelatin and bovine gelatin in solution (1 g/L) measured by dynamic light scattering.
Figura 4. Distribución de radios hidrodinámicos de gelatina entrecruzada y gelatina bovina en soluciones (1 g/L) mesuradas mediante dispersión dinámica de luz.
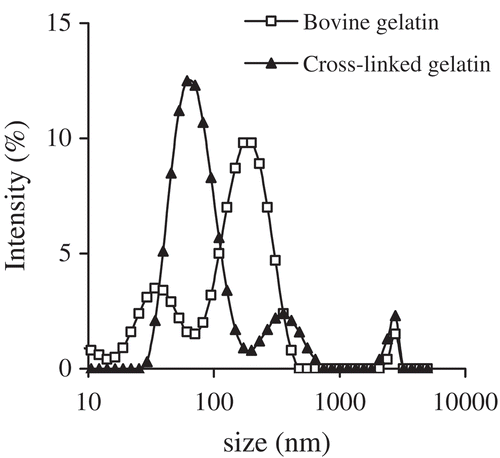
The stability of the colloidal dispersion appeared to increase with decreased particle size (Matthews & Rhodes, Citation1968). Zeta-potential is also an important index reflecting the stability of a colloidal dispersion, especially when its absolute value is higher than 30 mV (Kadar, Batalha, Fisher, & Roque, Citation2014). Higher values of zeta-potential in colloidal substances suggest that the respective colloidal dispersions are more resistant to aggregation (Hunter, Citation1981). Protein cross-linking has been shown to improve colloidal properties: for example, human serum albumin cross-linked by glutaraldehyde showed a decreased negative zeta-potential (Weber, Coester, Kreuter, & Langer, Citation2000). These reported results support the fact that cross-linked gelatin forms smaller aggregates in dispersion and provides good colloidal stabilization to the system applied.
Thermal stability of cross-linked gelatin
TG analysis is usually used to investigate the thermal stability and decomposition behaviour of polymers and composites. TG and DTG curves of the cross-linked gelatin and bovine gelatin are shown in . Mass loss of both gelatins in region I (20−105°C) reflects their ability to adsorb water. The cross-linked gelatin had higher water adsorption () and lost adsorbed water at higher temperatures (Tmax1, 45.8 ± 0.8°C versus 41.7 ± 0.6°C) () in somewhat larger amounts (16.42% ± 0.25% versus 14.92% ± 0.31%) (). In region II (105−450°C) thermal decomposition occurred. Respective maximum decomposition rates of the cross-linked gelatin and bovine gelatin were at temperatures (Tmax2) around 323.7 ± 0.8°C and 320.8 ± 1.0°C, respectively (); at the same time, the respective mass losses were 50.10% ± 0.28% and 50.90% ± 0.77% (). These results demonstrate that cross-linked gelatin has higher thermal stability, because it has a higher thermal decomposition temperature and reduced mass loss tendency following thermal treatment.
Figure 5. TG (a) and DTG (b) curves for cross-linked gelatin and bovine gelatin measured at a heating rate of 10°C/min under air atmosphere.
Figura 5. Curvas TG (a) y DTG (b) de gelatina entrecruzada y gelatina bovina medidas a una temperatura de 10°C/min bajo aire atmosférico.
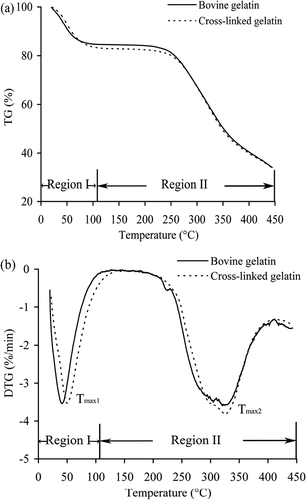
Gelatin films cross-linked by glutaraldehyde (Bigi, Cojazzi, Panzavolta, Rubini, & Roveri, Citation2001) and dialdehyde alginate (Boanini, Rubini, Panzavolta, & Bigi, Citation2010) were reported to have increased thermal stability at higher denaturation temperatures (up to 30°C for glutaraldehyde, and 3°C for dialdehyde alginate). TGase-induced cross-linking was reported to enhance the thermal stability of caseinate (Luo & Zhao, Citation2014) and β-lactoglobulin in heat-shocked whey proteins (Damodaran & Agyare, Citation2013). The present study shares the same conclusions as these studies: cross-linked gelatin showed higher resistance to thermal treatment due to dityrosine formation in its molecules.
Gelation of cross-linked gelatin
The gelling properties of cross-linked gelatin and bovine gelatin were also assessed. G′ changes in dispersion during time sweeps were measured, and the results are shown in . The gelation time of the cross-linked gelatin was about 14 min (14.1 ± 0.9 min), much shorter than that of the bovine gelatin (34.1 ± 0.5 min). After gel formation, G′ values of the cross-linked gelatin were far higher than those of the bovine gelatin, suggesting that the gels generated from the cross-linked gelatin had a more stable and firmer structure (Comfort & Howell, Citation2002). At the same time, gel strength of cross-linked gelatin and bovine gelatin was also assessed. The results, listed in , clearly reveal that the cross-linked gelatin was better able to form stronger gels than the bovine gelatin, with an increase of 12–14% in gel strength detected.
Table 1. Strengths of gels generated from cross-linked gelatin and bovine gelatin at three suggested protein concentrations.
Tabla 1. Resistencia de los geles generados a partir de gelatina entrecruzada y gelatina bovina con tres concentraciones proteínicas sugeridas.
Figure 6. Time sweep measurements of cross-linked gelatin and bovine gelatin solutions at protein concentration of 66.7 g/L.
Figura 6. Mediciones del tiempo de barrido de las soluciones de gelatina entrecruzada y gelatina bovina con una concentración proteínica de 66,7 g/L.
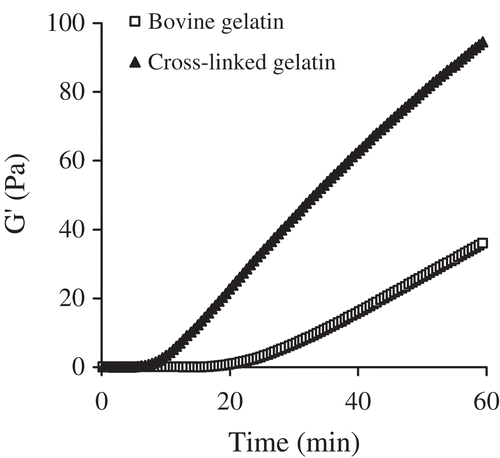
Protein cross-linking yields protein polymers, thereby impacting on protein interaction and gelation (Kuraishi, Yamazaki, & Susa, Citation2001). Gelatin gelation is a process during which transition from random coils to triple helices occurs (Kuijpers et al., Citation1999). Dityrosine formation in cross-linked gelatin resulted in additional covalent bonds within and between the gelatin molecules. These bonds result in stronger interaction between molecules than hydrogen bonds, and thus conferred the cross-linked gelatin with enhanced gelling properties, such as better gel formation. SPI cross-linked by the mixture of HRP, glucose oxidase, and glucose had a shorter gelation time during formation of the acidified gels (Jiang & Zhao, Citation2014), providing support to the present results. It is also known that gel strength is governed by molecular weight distribution and the interactions between molecules (Kaewruang, Benjakul, & Prodpran, Citation2013). Cross-linking of gelatin products by TGase can increase gel strength (Gómez-Guillén, Sarabia, Solas, & Montero, Citation2001; Mohtar et al., Citation2013), due to the formation of covalent bonds in the molecules. It is reasonable to assume that cross-linked gelatin will form gels with greater strength. Gelation time and gel strength are two important indices of gelatin quality (Karim & Bhat, Citation2009). The present findings imply that the cross-linking described can be applied to gelatin production to yield a gelatin product with enhanced properties.
Conclusion
The present results show that bovine gelatin can be cross-linked by a mixture comprising two oxidases (horseradish peroxidase and glucose oxidase) and glucose. The cross-linked gelatin contained more polymerized molecules and had a more ordered secondary structure, due to dityrosine formation. It showed good colloidal stabilization, as it formed aggregates with smaller hydrodynamic radius and enlarged negative zeta-potential in dispersion than bovine gelatin. It exhibited higher thermal stability upon heating to high temperature, resulting in higher decomposition temperature but slight lower mass loss. More importantly, it also formed gels in less time and with greater strength. Cross-linked gelatin therefore has potential applications as an additive, with better colloidal, thermal, and gelling properties than bovine gelatin.
Acknowledgement
The authors thank the anonymous reviewers and editors for their valuable advice.
Disclosure statement
No potential conflict of interest was reported by the authors.
Additional information
Funding
Notes on contributors
Yan-Ping Han
Miss YP Han is studying at Northeast Agricultural University, and will shortly complete her MD in Food Science and Technology under the direction of Prof. XH Zhao. Her work focuses on enzymatic modification of gelatin and application of the modified gelatin.
Xin-Huai Zhao
Prof. XH Zhao complected his MD and PhD in Food Science and Chemistry from Northeast Agricultural University and Ocean University of China, respectively. He has been working at Northeast Agricultural University for more than 30 years, and has more than 200 research publications on different domains of food chemistry. His work mostly focuses on modification and bioactivities of food proteins/peptides, health benefits of phytochemicals, and application of food microorganism for bioconversion and biodegradation.
References
- AOAC. (2005). Official Methods of Analysis of Association of Official analytical Chemists International (18th ed.). Gaithersburg, USA: AOAC International.
- Belitz, H.D., Grosch, W., & Schieberle, P. (2009). Food Chemistry (4th Revised and Extended ed.). Berlin, Germany: Springer Verlag.
- Bessho, M., Furuta, M., Kojima, T., Okuda, S., & Hara, M. (2005). Gelatin hydrogels cross-linked by γ-ray irradiation: Materials for absorption and release of dye. Journal of Biomaterials Science Polymer Edition, 16, 715–724. doi:10.1163/1568562053992478
- Bhat, R., & Karim, A.A. (2009). Ultraviolet irradiation improves gel strength of fish gelatin. Food Chemistry, 113, 1160–1164. doi:10.1016/j.foodchem.2008.08.039
- Bigi, A., Cojazzi, G., Panzavolta, S., Roveria, N., & Rubini, K. (2002). Stabilization of gelatin films by crosslinking with genipin. Biomaterials, 23, 4827–4832. doi:10.1016/S0142-9612(02)00235-1
- Bigi, A., Cojazzi, G., Panzavolta, S., Rubini, K., & Roveri, N. (2001). Mechanical and thermal properties of gelatin films at different degrees of glutaraldehyde crosslinking. Biomaterials, 22, 763–768. doi:10.1016/S0142-9612(00)00236-2
- Boanini, E., Rubini, K., Panzavolta, S., & Bigi, A. (2010). Chemico-physical characterization of gelatin films modified with oxidized alginate. Acta Biomaterialia, 6, 383–388. doi:10.1016/j.actbio.2009.06.015
- Cao, N., Fu, Y., & He, J. (2007). Mechanical properties of gelatin films cross-linked, respectively, by ferulic acid and tannin acid. Food Hydrocolloids, 21, 575–584. doi:10.1016/j.foodhyd.2006.07.001
- Chang, C.-H., & Zhao, X.-H. (2012). In vitro digestibility and rheological properties of caseinates treated by an oxidative system containing horseradish peroxidase, glucose oxidase and glucose. International Dairy Journal, 27, 47–52. doi:10.1016/j.idairyj.2012.07.004
- Comfort, S., & Howell, N.K. (2002). Gelation properties of soya and whey protein isolate mixtures. Food Hydrocolloids, 16, 661–672. doi:10.1016/S0268-005X(02)00033-4
- Damodaran, S., & Agyare, K.K. (2013). Effect of microbial transglutaminase treatment on thermal stability and pH-solubility of heat-shocked whey protein isolate. Food Hydrocolloids, 30, 12–18. doi:10.1016/j.foodhyd.2012.04.012
- Davies, K.J., Delsignore, M.E., & Lin, S.W. (1987). Protein damage and degradation by oxygen radical. II. Modification of amino acids. Journal of Biological Chemistry, 262, 9902–9907.
- De Carvalho, R.A., & Grosso, C.R.F. (2004). Characterization of gelatin based films modified with transglutaminase, glyoxal and formaldehyde. Food Hydrocolloids, 18, 717–726. doi:10.1016/j.foodhyd.2003.10.005
- Derkatch, S.R., Petrova, L.A., Izmailova, V.N., & Tarasevitch, B.N. (1999). Properties of emulsion films made from binary aqueous mixtures of gelatin-surfactant: The effect of concentration and pH. Colloids and Surfaces A: Physicochemical and Engineering Aspects, 152, 189–197. doi:10.1016/S0927-7757(98)00615-3
- Gilsenan, P.M., & Ross-Murphy, S.B. (2000). Rheological characterisation of gelatins from mammalian and marine sources. Food Hydrocolloids, 14, 191–195. doi:10.1016/S0268-005X(99)00050-8
- Gómez-Guillén, M.C., Sarabia, A.I., Solas, M.T., & Montero, P. (2001). Effect of microbial transglutaminase on the functional properties of megrim (Lepidorhombus boscii) skin gelatin. Journal of the Science of Food & Agriculture, 81, 665–673. doi:10.1002/jsfa.865
- Gómez-Guillén, M.C., Turnay, J., Fernández-Díaz, M.D., Ulmo, N., Lizarbe, M.A., & Montero, P. (2002). Structural and physical properties of gelatin extracted from different marine species: A comparative study. Food Hydrocolloids, 16, 25–34. doi:10.1016/S0268-005X(01)00035-2
- Hunter, R.J. (1981). Zeta Potential in Colloid Science. London: Academic Press.
- Jiang, P., & Zhao, X.-H. (2014). Gelation and in vitro Digestibility of Soybean Protein Isolate Treated by a Ternary System Containing Horseradish Peroxidase, Glucose Oxidase, and Glucose. International Journal of Food Properties, 17, 2284–2297. doi:10.1080/10942912.2013.798737
- Jongjareonrak, A., Benjakul, S., Visessanguan, W., & Tanaka, M. (2006). Skin gelatin from bigeye snapper and brownstripe red snapper: Chemical compositions and effect of microbial transglutaminase on gel properties. Food Hydrocolloids, 20, 1216–1222. doi:10.1016/j.foodhyd.2006.01.006
- Kadar, E., Batalha, Í.L., Fisher, A., & Roque, A.C.A. (2014). The interaction of polymer-coated magnetic nanoparticles with seawater. Science of the Total Environment, 487, 771–777. doi:10.1016/j.scitotenv.2013.11.082
- Kaewruang, P., Benjakul, S., & Prodpran, T. (2013). Molecular and functional properties of gelatin from the skin of unicorn leatherjacket as affected by extracting temperatures. Food Chemistry, 138, 1431–1437. doi:10.1016/j.foodchem.2012.09.114
- Karim, A.A., & Bhat, R. (2009). Fish gelatin: Properties, challenges, and prospects as an alternative to mammalian gelatins. Food Hydrocolloids, 23, 563–576. doi:10.1016/j.foodhyd.2008.07.002
- Kuijpers, A.J., Engbers, G.H.M., Feijen, J., De Smedt, S.C., Meyvis, T.K.L., Demeester, J., … Dankert, J. (1999). Characterization of the network structure of carbodiimide cross-linked gelatin gels. Macromolecules, 32, 3325–3333. doi:10.1021/ma981929v
- Kuraishi, C., Yamazaki, K., & Susa, Y. (2001). Transglutaminase: Its utilization in the food industry. Food Reviews International, 17, 221–246. doi:10.1081/FRI-100001258
- Laemmli, U.K. (1970). Cleavage of structural proteins during the assembly of the head of bacteriophage T4. Nature, 227, 680–685. doi:10.1038/227680a0
- Lam, R.S.H., & Nickerson, M.T. (2014). The effect of pH and heat pre-treatments on the physicochemical and emulsifying properties of β-lactoglobulin. Food Biophysics, 9, 20–28. doi:10.1007/s11483-013-9313-4
- Luo, Z.L., & Zhao, X.H. (2014). Caseinate-gelatin and caseinate-hydrolyzed gelatin composites formed via transglutaminase: Chemical and functional properties. Journal of the Science of Food & Agriculture. doi:10.1002/jsfa.7042
- Matsuda, S., Iwata, H., Se, N., & Ikada, Y. (1999). Bioadhesion of gelatin films crosslinked with glutaraldehyde. Journal of Biomedical Materials Research, 45, 20–27. doi:10.1002/(ISSN)1097-4636
- Matthews, B.A., & Rhodes, C.T. (1968). The use of the Coulter Counter for investigating the coagulation kinetics of mixed monodisperse particulate systems. Journal of Colloid & Interface Science, 28, 71–81. doi:10.1016/0021-9797(68)90209-9
- Mohtar, N.F., Perera, C.O., Quek, S.-Y., & Hemar, Y. (2013). Optimization of gelatine gel preparation from New Zealand hoki (Macruronus novaezelandiae) skins and the effect of transglutaminase enzyme on the gel properties. Food Hydrocolloids, 31, 204–209. doi:10.1016/j.foodhyd.2012.10.011
- Muyonga, J.H., Cole, C.G.B., & Duodu, K.G. (2004). Extraction and physico-chemical characterisation of Nile perch (Lates niloticus) skin and bone gelatine. Food Hydrocolloids, 18, 581–592. doi:10.1016/j.foodhyd.2003.08.009
- Olijve, J., Mori, F., & Toda, Y. (2001). Influence of the molecular-weight distribution of gelatin on emulsion stability. Journal of Colloid & Interface Science, 243, 476–482. doi:10.1006/jcis.2001.7816
- Schrieber, R., & Gareis, H. (2007). Gelatine handbook: Theory and industrial practice. Weinheim: Wiley-VCH Verlag GmbH. KGaA.
- Silverstein, P.M., Webster, F.X., & Kiemle, D.J. (2005). Spectrometric Identification of Organic Compounds. Hoboken, NJ: John Wiley & Sons Press.
- Sobral, P.J.A., Menegalli, F.C., Hubinger, M.D., & Roques, M.A. (2001). Mechanical, water vapor barrier and thermal properties of gelatin based edible films. Food Hydrocolloids, 15, 423–432. doi:10.1016/S0268-005X(01)00061-3
- Stahmann, M.A., Spencer, A.K., & Honold, G.R. (1977). Cross linking of proteins in vitro by peroxidase. Biopolymers, 16, 1307–1318. doi:10.1002/(ISSN)1097-0282
- Vemulapalli, V., & Hoseney, R.C. (1998). Glucose Oxidase Effects on Gluten and Water Solubles 1. Cereal Chemistry, 75, 859–862. doi:10.1094/CCHEM.1998.75.6.859
- Weber, C., Coester, C., Kreuter, J., & Langer, K. (2000). Desolvation process and surface characterisation of protein nanoparticles. International Journal of Pharmaceutics, 194, 91–102. doi:10.1016/S0378-5173(99)00370-1
- Wu, D., Xu, G., Sun, Y., Zhang, H., Mao, H., & Feng, Y. (2007). Interaction between proteins and cationic gemini surfactant. Biomacromolecules, 8, 708–712. doi:10.1021/bm061033v
- Zhang, J.-J., Gu, M.-M., Zheng, T.-T., & Zhu, J.-J. (2009). Synthesis of gelatin-stabilized gold nanoparticles and assembly of carboxylic single-walled carbon nanotubes/Au composites for cytosensing and drug uptake. Analytical Chemistry, 81, 6641–6648. doi:10.1021/ac900628y