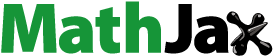
ABSTRACT
Oxidative stress (OS) has been considered as the main mediator of male infertility by causing abnormal sperm production and function, and, therefore, exploration of natural antioxidant has attracted a great deal of attention in human reproduction. Chitosan and its derivatives exhibit great antioxidant potential in various diseases, whereas the protective functions are not defined in spermatogenesis. Here, we show that chitosan oligosaccharide (COS), a degradation product of chitosan with a degree of polymerization of less than 10, is a strong antioxidant in spermatogonia cells. COS treatment reduces t-BHP-induced reactive oxygen species overproduction and programmed necrosis in GC-1 spg cells, and improves mitochondrial functions and cell viability by inhibiting excessive endoplasmic reticulum stress. Our findings provide novel insight into the protective role of COS in germ cell damage.
Introduction
Infertility is a disease defined by the failure of pregnancy after 1 year of regular and unprotected sexual intercourse. The World Health Organization estimates that about 190 million people worldwide suffer from infertility, and male infertility accounts for up to half of infertility cases (Vander Borght & Wyns, Citation2018). Male infertility is related to a variety of factors, including lifestyle (Durairajanayagam, Citation2018), individual genetics (Plaseska-Karanfilska et al., Citation2012), environmental factors (Anifandis et al., Citation2018), and clinical causes such as varicocele, endocrine disorders, and infections (Pichugova et al., Citation2022). It is worth noting that these risk factors are all linked to oxidative stress (OS) (Rashki Ghaleno et al., Citation2021). OS is defined as an imbalance between oxidation and antioxidant effects in the cells and tissues. Specifically, it refers to the excessive production of reactive oxygen species (ROS), which overwhelms the elimination system of antioxidants (Agarwal et al., Citation2018; Bisht et al., Citation2017). With these knowledge in hand, ROS serves as an independent marker of male-factored infertility (Agarwal et al., Citation2006). Remarkably, new data suggests an imbalance in semen pro- and antioxidant components leading to DNA fragmentation and decreased semen quality in men recovered from COVID-19 (Shcherbitskaia et al., Citation2022).
Chitosan oligosaccharides (COS) are prepared from the deacetylation and hydrolysis of chitin, and form by repeating N-acetyl-D-glucosamine combined with β-(1→4) glycosidic bonds, with a polymerization degree between 2 and 20 and an average molecular weight (MW) <10000 Da (Muanprasat & Chatsudthipong, Citation2017). Emerging data from various diseases describe the antioxidant capacity of COS, thereby exerting the effect of anti-inflammation, immunostimulation, anti-tumor, and promoting tissue regeneration (Guan & Feng, Citation2022). However, whether COS can function as antioxidants in spermatogonia cells remains unclear.
Here, using tert-butyl hydroperoxide (t-BHP)-induced GC-1 spg cells as OS model, we show that COS can inhibit programmed necrosis, protect against cellular damage caused by OS, and render COS an appealing agent for improving sperm health.
Materials and methods
Reagents and antibodies
Antibodies against Receptor interacting protein-1 (RIP-1) and Receptor interacting protein-3 (RIP-3) were purchased from ABclonal (Wuhan, China). Antibodies against phosphorylated mixed lineage kinase domain-like protein (p-MLKL) and CHOP were purchased from Affinity (Jiangsu, China). Anti-GAPDH against was purchased from Proteintech (Chicago, U.S.A.). Anti-ATF-4 was purchased from Bioss (Beijing, China). Anti-GRP78 was purchased from Abcam (Cambridge, MA, U.S.A.). Anti-β-Tubulin was purchased from Elabscience (Wuhan, China). The secondary antibodies were purchased from Absin (Shanghai, China). The 4’,6-Diamidino-2-phenylindole (DAPI) was purchased from Solarbio (Beijing, China). Necrostatin-1 (Nec-1) was purchased from Beyotime Biotechnology (Shanghai, China). The t-BHP was purchased from Macklin (Shanghai, China).
Cell culture
The spermatogonia germ cell line GC-1 spg cell (ATCC CRL-2053) was maintained in a medium containing 90% DMEM and 10% FBS and incubated under 37°C, 5% CO2. Cultures were split every 3 days.
Synthesis and characterization of COS
COS was prepared and purified in our laboratory using enzymatic method (Zhang et al., Citation2014). In brief, chitosan was incubated with chitosanase (made in house) with pH 5.5 for 3 hours at 45°C. The chitosanase was then inactivated and removed by sedimentation with trichloroacetic acid. pH value of hydrolysate was adjusted to neutral with sodium hydroxide solution. After spray drying, desalting, and ethanol precipitation, the purified COS was proceeded with infrared spectroscopy (IR) analysis.
Cell proliferation and cytotoxicity assay
CCK-8 assay was performed using Cell Counting Kit-8 Assay (MCE, New Jersey, U.S.A.) according to the manufacturer’s protocol. 9 × 103 cells in 100 μl medium were plated in 1 well of 96-well plate and incubated overnight, mixed with 10 μl CCK-8 solution, and incubated in a cell culture incubator for 2 hours. The optical density (OD) of each well at 450 nm was read using a microplate reader.
Real-time quantitative RT-PCR
RNA was extracted using RNA-easy lsolation Reagent (Vazyme, Jiangsu, China) and reverse transcribed using HiScript III RT SuperMix for qPCR (Vazyme, Jiangsu, China). Reactions were run on a CFX96 instrument using the ChamQ Universal SYBR qPCR Master Mix (Vazyme, Jiangsu, China). All expression values were scaled to GAPDH. The sequences (from 5′ to 3′) of gene-specific primers (forward primer; reverse primer) used in real-time RT-PCR are as follows: Mouse Rip-1 (CGACTTCCAGACACCAAGCCATC; TTTCCACTGCCTTCCCAGGTTTTC); Mouse Rip-3 (AAATGGATTGCCCGAGGGAAACC; AACTGATGTGTCCTGTGCTTGCC); Mouse Gapdh (TCCCACTCTTCCACCTTCGATGC; GGGTCTGGGATGGAAATTGTGAGG).
ROS detection
ROS in cells was measured using Reactive Oxygen Species Assay Kit (Beyotime Biotechnology, Shanghai, China) according to the manufacturer’s protocol. Cells were incubated with DCFH-DA for 20 min in a cell culture incubator at 37°C, washed for three times with serum-free cell culture solution, and imaged on a fluorescence microscope (Nikon, Ni-U).
Mitochondrial membrane potential detection
Mitochondrial membrane potential assay was performed using the JC-1 Mitochondrial Membrane Potential Assay Kit (YEASEN, Shanghai, China) according to the manufacturer’s protocol. Cells were washed with PBS, incubated with JC-1 solution in the incubator at 37°C for 20 min, washed with 1 × JC-1 staining buffer, and imaged on a fluorescence microscope (Nikon, Ni-U). The red-to-green fluorescence ratio was analyzed by ImageJ.
Immunofluorescence assay
Cells were fixed in 4% paraformaldehyde. Slides were blocked in PBS containing 5% normal donkey serum (Solarbio, Beijing, China) and 0.5% Triton X-100 for 1 hour at room temperature, incubated with primary antibodies overnight at 4°C, washed, incubated with secondary antibodies for 2 hours at room temperature, washed, coverslipped with and imaged on a fluorescence microscope (Nikon, Ni-U). The following antibodies (dilution ratio) were used in immunofluorescence assay: p-MLKL (1:100) and GRP78 (1:250).
Immunoblotting assay
Cells were incubated in 1 × RIPA buffer (Epizyme, Shanghai, China) with 1 × protease inhibitor (Solarbio, Beijing, China) for 1 hour on ice. Protein concentration was quantified using BCA protein assay kit (Beyotime Biotechnology, Shanghai, China). Twenty micrograms of protein lysate was resolved on an SDS-PAGE gel and transferred to a PVDF membrane (Bio-rad). Blots were blocked in 5% non-fat dry milk, incubated with primary antibody overnight at 4°C, washed, incubated with secondary antibody for 1 hour at room temperature, washed, and developed with BeyoECL Plus (Beyotime Biotechnology, Shanghai, China). The following antibodies (dilution ratio) were used in WB experiments: GAPDH (1:1000), β-Tubulin (1:1000), RIP-1 (1:1000), RIP-3 (1:1000), p-MLKL (1:1000), ATF-4 (1:1000), GRP78 (1:1000), CHOP (1:1000), and Secondary antibodies (1:2000).
Statistical analysis
All experiments were repeated three times independently. Statistical analysis was performed using GraphPad Prism 8.0 software. Images protein band gray values and fluorescence intensity assays were processed by ImageJ software. Measurement data was represented as ± SD. One-way ANOVA was used for comparison between groups, and the Tukey method was used for pair comparison. p < .05 was considered statistically significant.
Results
COS inhibits t-BHP-induced ROS production in GC-1 spg cells
To mimic OS in spermatogonia cells, we treated GC-1 spg cells with t-BHP, a commonly used hydroperoxides in a variety of oxidation processes (Luo et al., Citation2013). As expected, t-BHP treatment (100 µmol/L) for 24 hours heavily induced the intracellular ROS generation in GC-1 spg cells by fourfold compared with untreated cells, revealed by DCFH-DA assay, and this was inhibited by Necrostatin-1 (Nec-1), a specific allosteric inhibitor of necrotizing apoptosis, which suppress ROS in mice acute lung injury model (Lin et al., Citation2020). Of interest, as well, COS treatment could block ROS production, with statistical significance (p < .001) (). The COS treatment group was equivalent to the Nec-1 treatment effect ().
Figure 1. Effects of COS on ROS production and cell viability in GC-1 spg cells treated with t-BHP. (a) DCFH-DA was used to detect intracellular ROS. Scale, 200 μm. Green fluorescence represents DCFH-DA, which also represents ROS level. (b) Cell quantification was observed by green fluorescence. The results were presented as x ± SD (n = 3). ***p < .001 compared with the t-BHP group, ###p < .001 compared with the control group, ns indicates not statistically significant. (c) Use light microscopy to make morphological observations of each group of cells. Scale, 100 μm. (d) Effects of COS on the viability of t-BHP-induced GC-1 spg cells were detected by CCK-8. The results were presented as x ± SD (n = 3). **p < .01, ***p < .001 compared with the t-BHP group; ####p < .0001 compared with the control group; ns indicates not statistically significant.
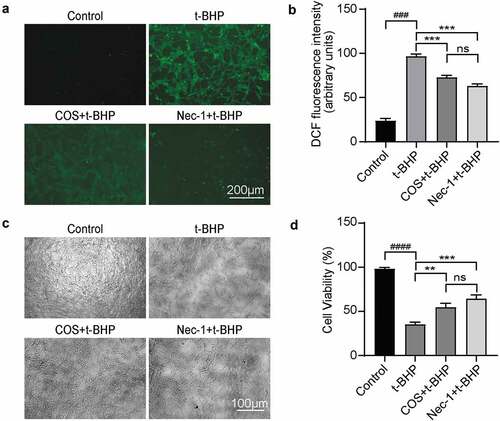
COS raises viability in GC-1 spg cells with t-BHP treatment
Given t-BHP and ROS both trigger general cell death, we tested whether COS treatment could block t-BHP-induced viability reduction in GC-1 spg cells. As shown in , untreated GC-1 spg cells exhibited typical epithelial cell morphology, whereas, when treated with t-BHP, cells shrank, intercellular space volume increased, and cell density reduced. By contrast, when treated with COS or Nec-1 together with t-BHP, cells maintained normal GC-1 spg cell shape with similar cell density. Further CCK-8 assay revealed that, after 24 hours treatment with COS or Nec-1, the cell viability was significantly higher than that of the t-BHP alone treated cells, and there was no significant difference between COS and Nec-1 treatment groups ().
COS exhibits anti-programmed necrosis activity in GC-1 spg cells
Considering that Nec-1 inhibits cell loss in our OS model, we speculate that t-BHP treated cells mainly underwent necrosis, a type of cell death recently identified. To further examine it at the molecular level, we assessed the expression of key molecules in necroptotic signaling pathways. RNA and protein levels of RIP-1, RIP-3 and p-MLKL were markedly increased in t-BHP treated GC-1 spg cells relative to control cells, indicating programmed necrosis occurrence under ROS overproduction (). While COS treatment clearly hampered the activation of necroptotic signaling pathways, and similar results were observed in immunofluorescence staining assay (). Notably, there was no significant difference from the Nec-1 group ().
Figure 2. Effects of COS on cell viability and the expression of p-MLKL, RIP-3, and RIP-1 in GC-1 spg cells treated with t-BHP. (a) The mRNA level of RIP-3 and RIP-1 in GC-1 spg cells was detected by RT-PCR. The results are presented as x ± SD (n = 3). *p < .05, **p < .01, ***p < .001 compared with the t-BHP group; ##p < .01, ###p < .001 compared with the control group; ns indicates not statistically significant. Protein level of p-MLKL, RIP-3, and RIP-1 in GC-1 spg cells was determined by western blot, and the results are presented as x ± SD (n = 3). **p < .01 compared with the t-BHP group; ##p < .01, ###p < .001 compared with the control group; ns indicates not statistically significant. (b) The fluorescence intensity of p-MLKL was detected by immunofluorescence. Scale, 400 μm.
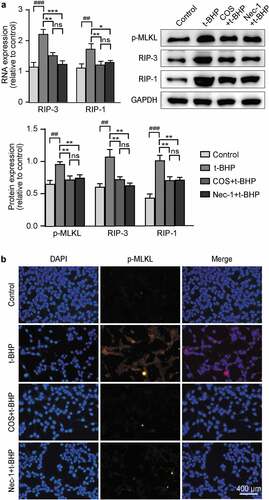
COS reverses mitochondrial membrane potential loss in GC-1 spg cells
High ROS exposure often targets mitochondria (Rizwan et al., Citation2020), thereby mediating programmed necrosis (Velásquez et al., Citation2022). To examine whether COS exerts a regulatory role in GC-1 spg cells by mediating ROS-mitochondrial-necrosis pathways. We applied mitochondrial membrane potential detection to determine mitochondrial membrane potential in the presence of COS. JC-1 staining analysis detected strong red fluorescence and weak green fluorescence in the control GC-1 spg cells. When treated with t-BHP, red fluorescence disappeared, and green fluorescence accumulated in cytoplasm, indicating the loss of mitochondrial membrane potential. Treatment of COS prior t-BHP induction could effectively block the mitochondrial membrane potential loss as compared with the t-BHP induced cells, with significantly increased red fluorescence proportion (0.534 ± 0.012 vs. 0.787 ± 0.014, p < .05) (), and the results were similar to those in the Nec-1 treatment group ().
Figure 3. Effects of COS on mitochondrial membrane potential in GC-1 spg cells treated with t-BHP. (a) JC-1 kit was used to detect mitochondrial membrane potential in GC-1 spg cells treated with t-BHP. Green fluorescence represents JC-1 monomer and red fluorescence represents JC-1 complex. Scale, 500 μm. (b) Cell quantification was observed by green and red fluorescence. The results were presented as x ± SD (n = 3). *p < .05 compared with the t-BHP group, ns indicates not statistically significant.
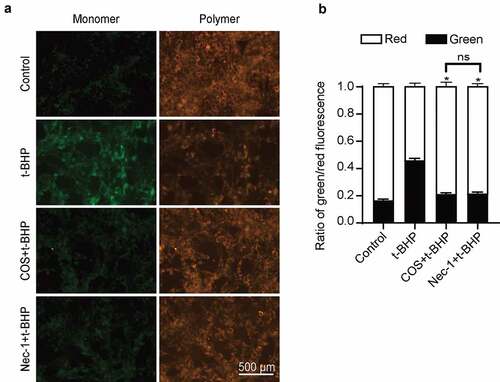
COS reduces endoplasmic reticulum stress in GC-1 spg cells
It is now clear that mitochondrial networks and endoplasmic reticulum (ER) are physically connected, and in particular, mitochondrial metabolism is enhanced by this interaction. To determine whether COS was involved in the anti-ER stress effects of t-BHP, ER stress markers were assessed by western blot. ER stress-relevant proteins ATF-4, CHOP, and GRP78 in the t-BHP treated cells were dramatically upregulated compared with control GC-1 spg cells, whereas pretreatment with COS and Nec-1 similarly decreased the levels of these ER stress markers (). Similar trends were also observed by immunofluorescence staining ().
Figure 4. Effects of COS on the expression of ATF-4, chop, and GRP78 in GC-1 spg cells treated with t-BHP. (a) ATF-4, CHOP, and GRP78 expression levels in GC-1 spg cells were determined by western blot. (b) The results are presented as ATF-4/β-Tubulin, CHOP/β-Tubulin, and GRP78/β-Tubulin. The results are presented as x ± SD (n = 3). **p < .01, ***p < .001 compared with the t-BHP group; ##p < .01, ###p < .001 compared with the control group; ns indicates not statistically significant. (C) The fluorescence intensity of GRP78 was detected by immunofluorescence. Scale, 500 μm.
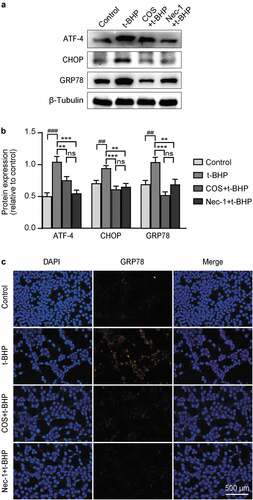
Discussion
As we all know, OS is the major threat to human health, which can lead to damage to the reproductive system, cardiovascular disease, and other diseases (Deluao et al., Citation2022; Rajlic et al., Citation2023). Despite remarkable progress in understanding the endogenously produced antioxidants in semen, exploration of exogenous antioxidants with better efficacy and no side effect attracts a great deal of attention in the field of male infertility (Bui et al., Citation2018; Tremellen, Citation2008). Here, we identify COS as a potential antioxidant which could shield spermatogonia cells from programmed necrosis induced by OS.
ROS plays an important role in regulating various cell functions. However, excessive production of ROS can cause OS in the body, affecting the oxidative phosphorylation level of mitochondria, promoting the inactivation of intracellular proteins and DNA damage, and eventually inducing cell death. During sperm development, excessive ROS can disrupt the intracellular antioxidant system homeostasis, leading to severe cell damage and cell death through activation of necrosis or apoptosis, causing a decline in sperm quality (Chakraborty & Roychoudhury, Citation2022). In this study, t-BHP was used to establish cell oxidative damage model, and it was found that COS treatment counteracted the excessive ROS generation induced by t-BHP and raises cell viability. Our data characterize the antioxidant properties of COS in spermatogonia cells. Although the exact mechanism responsible for scavenging excessively produced ROS is currently unclear, it is proposed that hydroxyl and free amino groups render COS antioxidant activity, probably attributed to reaction of active hydrogen atoms within COS with hydroxyl and superoxide anion to form stable macromolecule radicals (Liu et al., Citation2009; Xia et al., Citation2011), leading to interruption of the radical reaction. Molecular weight is another factor determining the antioxidant properties of COS (Jiang et al., Citation2014; Su et al., Citation2015; Yue et al., Citation2017). Indeed, COS exhibit stronger antioxidant capacity relative to chitosan in other disease models which are associated with OS (Kim & Rajapakse, Citation2005; Su et al., Citation2015; Xia et al., Citation2011).
OS is associated with programmed necrosis in many cell types. Several studies have shown that the accumulation of ROS can promote RIP-1 autophosphorylation and recruit RIP-3 to form necrosome (Wu et al., Citation2014). Necrotic bodies play an important role in the regulation of necrosis. We used Nec-1, a specific inhibitor of programmed necrosis, to confirm the presence of programmed necrosis in t-BHP-induced GC-1 spg cell injury. We found that COS protect GC-1 spg cells from oxidative damage by inhibiting programmed necrosis. t-BHP induced upregulation of proteins and genes for RIP-1, RIP-3, and p-MLKL, and this increase was reversed by COS treatment. The finding that COS inhibits necrosis of GC-1 spg cells by blocking the key molecules in necroptotic signaling pathways suggests that necrosis serves as an intriguing target of spermatogonia cells loss. Programmed necrosis is a novel caspase-independent cell death that is regulated by death signals (Degterev et al., Citation2005) and has been found to be the major hallmark of male infertility (Dong et al., Citation2022). Nec-1 can directly bind the RIP-1/RIP-3 complex to inhibit programmed necrosis (Degterev et al., Citation2008). For spermatogonia cells, Nec-1 and COS exhibit similar anti-necrosis activity, but whether COS inhibits necrosis by acting directly or indirectly on RIP-1 and RIP-3 requires further investigation.
Mitochondria and ER are not only interconnected in physiological functions but also have signaling under pathological conditions that affect each other’s function (Vannuvel et al., Citation2013). In the early stages of ER stress, the ER and mitochondrial network are more physically connected than before stress, favoring calcium transfer between the ER and mitochondria, and enhancing the metabolic parameters such as mitochondrial ATP production, oxygen consumption, and mitochondrial transmembrane potential (Bravo et al., Citation2011). In spermatogonia cells, we find that COS effectively protects against mitochondrial membrane potential loss through targeting excessive ER stress induced by t-BHP. This observation is consistent with COS’s protective role in human renal carcinoma cell death, both in vitro and in vivo, mainly via ROS-dependent ER stress pathways (Zhai et al., Citation2019), highlighting the ways in which COS mediates ER stress, and, consequently, regulates mitochondrial membrane potential.
We show that Nec-1 is a potent inhibitor of necroptosis in spermatogonia cells. However, its half-life is very short (about 1−2 hours) (Cao & Mu, Citation2021), and it also targets other proteins, such as IDO, an enzyme involved in tryptophan metabolism, thereby resulting in metabolic instability. Given the characteristics of COS with water solubility, non-toxicity, and in vivo stability, it displays superior clinical potential in several OS linked diseases, such as cardiovascular diseases, atherogenesis (García-Sánchez et al., Citation2020), cancer (Zou et al., Citation2016), and Parkinson’s disease (Xu et al., Citation2011). Notably, COS can be further modified, and some of the COS derivatives exhibit increased antioxidant capacity. For example, gallic acid-conjugated COS shows significantly higher radical scavenging activity relative to COS induced by H2O2 in human lung epithelial A549 cells (Vo et al., Citation2017). As such, whether gallic acid-conjugated COS will work similarly in spermatogonia cells to what was observed in A549 cells, and what other COS derivatives have relatively high antioxidant activity, compared to COS, will be the subjects of future investigations.
In this study, we found that COS treatment reduced ROS overproduction and programmed necrosis in t-BHP-induced GC-1 spg cells, and improved mitochondrial function and cell viability by inhibiting excessive ER stress. This study provides a theoretical basis for COS in the treatment of oxidative damage in germ cell damage.
Disclosure statement
No potential conflict of interest was reported by the author(s).
Additional information
Funding
References
- Agarwal, A., Rana, M., Qiu, E., AlBunni, H., Bui, A. D., & Henkel, R. (2018). Role of oxidative stress, infection and inflammation in male infertility. Andrologia, 50(11), e13126. https://doi.org/10.1111/and.13126
- Agarwal, A., Sharma, R. K., Nallella, K. P., Thomas, A. J., Jr., Alvarez, J. G., & Sikka, S. C. (2006). Reactive oxygen species as an independent marker of male factor infertility. Fertility and Sterility, 86(4), 878–885. https://doi.org/10.1016/j.fertnstert.2006.02.111
- Anifandis, G., Katsanaki, K., Lagodonti, G., Messini, C., Simopoulou, M., Dafopoulos, K., & Daponte, A. (2018). The effect of glyphosate on human sperm motility and sperm DNA fragmentation. International Journal of Environmental Research and Public Health, 15(6), 1117. https://doi.org/10.3390/ijerph15061117
- Bisht, S., Faiq, M., Tolahunase, M., & Dada, R. (2017). Oxidative stress and male infertility. Nature Reviews Urology, 14(8), 470–485. https://doi.org/10.1038/nrurol.2017.69
- Bravo, R., Vicencio, J. M., Parra, V., Troncoso, R., Munoz, J. P., Bui, M., Quiroga, C., Rodriguez, A. E., Verdejo, H. E., Ferreira, J., Iglewski, M., Chiong, M., Simmen, T., Zorzano, A., Hill, J. A., Rothermel, B. A., Szabadkai, G., & Lavandero, S. (2011). Increased ER–mitochondrial coupling promotes mitochondrial respiration and bioenergetics during early phases of ER stress. Journal of Cell Science, 124(13), 2143–2152. https://doi.org/10.1242/jcs.080762
- Bui, A. D., Sharma, R., Henkel, R., & Agarwal, A. (2018). Reactive oxygen species impact on sperm DNA and its role in male infertility. Andrologia, 50(8), e13012. https://doi.org/10.1111/and.13012
- Cao, L., & Mu, W. (2021). Necrostatin-1 and necroptosis inhibition: Pathophysiology and therapeutic implications. Pharmacological Research, 163, 105297. https://doi.org/10.1016/j.phrs.2020.105297
- Chakraborty, S., & Roychoudhury, S. (2022). Pathological roles of reactive oxygen species in male reproduction. Advances in Experimental Medicine and Biology, 1358, 41–62. https://doi.org/10.1007/978-3-030-89340-8_3
- Degterev, A., Hitomi, J., Germscheid, M., Ch’en, I. L., Korkina, O., Teng, X., Abbott, D., Cuny, G. D., Yuan, C., Wagner, G., Hedrick, S. M., Gerber, S. A., Lugovskoy, A., & Yuan, J. (2008). Identification of RIP1 kinase as a specific cellular target of necrostatins. Nature Chemical Biology, 4(5), 313–321. https://doi.org/10.1038/nchembio.83
- Degterev, A., Huang, Z., Boyce, M., Li, Y., Jagtap, P., Mizushima, N., Cuny, G. D., Mitchison, T. J., Moskowitz, M. A., & Yuan, J. (2005). Chemical inhibitor of nonapoptotic cell death with therapeutic potential for ischemic brain injury. Nature Chemical Biology, 1(2), 112–119. https://doi.org/10.1038/nchembio711
- Deluao, J. C., Winstanley, Y., Robker, R. L., Pacella-Ince, L., Gonzalez, M. B., & McPherson, N. O. (2022). Oxidative stress and reproductive function: Reactive oxygen species in the mammalian pre-implantation embryo. Reproduction, 164(6), F95–f108. https://doi.org/10.1530/rep-22-0121
- Dong, X., Yang, F., Xu, X., Zhu, F., Liu, G., Xu, F., Chen, G., Cao, C., Teng, L., Li, X., Wang, L., & Li, B. (2022). Protective effect of C-phycocyanin and apo-phycocyanin subunit on programmed necrosis of GC-1 spg cells induced by H(2) O(2). Environmental Toxicology, 37(6), 1275–1287. https://doi.org/10.1002/tox.23482
- Durairajanayagam, D. (2018). Lifestyle causes of male infertility. Arab Journal of Urology, 16(1), 10–20. https://doi.org/10.1016/j.aju.2017.12.004
- García-Sánchez, A., Miranda-Díaz, A. G., & Cardona-Muñoz, E. G. (2020). The role of oxidative stress in physiopathology and pharmacological treatment with pro- and antioxidant properties in chronic diseases. Oxidative Medicine and Cellular Longevity, 2020, 2082145. https://doi.org/10.1155/2020/2082145
- Guan, Z., & Feng, Q. (2022). Chitosan and chitooligosaccharide: The promising non-plant-derived prebiotics with multiple biological activities. International Journal of Molecular Sciences, 23(12), 6761. https://doi.org/10.3390/ijms23126761
- Jiang, M., Cheng, Q., Su, W., Wang, C., Yang, Y., Cao, Z., & Ding, F. (2014). The beneficial effect of chitooligosaccharides on cell behavior and function of primary Schwann cells is accompanied by up-regulation of adhesion proteins and neurotrophins. Neurochemical Research, 39(11), 2047–2057. https://doi.org/10.1007/s11064-014-1387-y
- Kim, S.-K., & Rajapakse, N. (2005). Enzymatic production and biological activities of chitosan oligosaccharides (COS): A review. Carbohydrate Polymers, 62(4), 357–368. https://doi.org/10.1016/j.carbpol.2005.08.012
- Lin, B., Jin, Z., Chen, X., Zhao, L., Weng, C., Chen, B., Tang, Y., & Lin, L. (2020). Necrostatin‑1 protects mice from acute lung injury by suppressing necroptosis and reactive oxygen species. Molecular Medicine Reports, 21(5), 2171–2181. https://doi.org/10.3892/mmr.2020.11010
- Liu, H. T., Li, W. M., Xu, G., Li, X. Y., Bai, X. F., Wei, P., Yu, C., & Du, Y. G. (2009). Chitosan oligosaccharides attenuate hydrogen peroxide-induced stress injury in human umbilical vein endothelial cells. Pharmacological Research, 59(3), 167–175. https://doi.org/10.1016/j.phrs.2008.12.001
- Luo, C., Li, Y., Wang, H., Feng, Z., Li, Y., Long, J., & Liu, J. (2013). Mitochondrial accumulation under oxidative stress is due to defects in autophagy. Journal of Cellular Biochemistry, 114(1), 212–219. https://doi.org/10.1002/jcb.24356
- Muanprasat, C., & Chatsudthipong, V. (2017). Chitosan oligosaccharide: Biological activities and potential therapeutic applications. Pharmacology & Therapeutics, 170, 80–97. https://doi.org/10.1016/j.pharmthera.2016.10.013
- Pichugova, S. V., Rozanova, S. M., & Beikin, Y. B. (2022). Diagnosis of bacteriospermia and its impact on spermogram parameters in adolescents with varicocele. Russian Clinical Laboratory Diagnostics, 67(8), 463–470. https://doi.org/10.51620/0869-2084-2022-67-8-463-470
- Plaseska-Karanfilska, D., Noveski, P., Plaseski, T., Maleva, I., Madjunkova, S., & Moneva, Z. (2012). Genetic causes of male infertility. Balkan Journal of Medical Genetics, 15(Supplement), 31–34. https://doi.org/10.2478/v10034-012-0015-x
- Rajlic, S., Treede, H., Münzel, T., Daiber, A., & Duerr, G. D. (2023). Early detection is the best prevention—Characterization of oxidative stress in diabetes mellitus and its consequences on the cardiovascular system. Cells, 12(4), 583. https://doi.org/10.3390/cells12040583
- Rashki Ghaleno, L., Alizadeh, A., Drevet, J. R., Shahverdi, A., & Valojerdi, M. R. (2021). Oxidation of sperm DNA and male infertility. Antioxidants (Basel), 10(1), 97. https://doi.org/10.3390/antiox10010097
- Rizwan, H., Pal, S., Sabnam, S., & Pal, A. (2020). High glucose augments ROS generation regulates mitochondrial dysfunction and apoptosis via stress signalling cascades in keratinocytes. Life Sciences, 241, 117148. https://doi.org/10.1016/j.lfs.2019.117148
- Shcherbitskaia, A. D., Komarova, E. M., Milyutina, Y. P., Ishchuk, M. A., Sagurova, Y. M., Safaryan, G. K., Lesik, E. A., Gzgzyan, A. M., Bespalova, O. N., & Kogan, I. Y. (2022). Oxidative stress markers and sperm DNA fragmentation in men recovered from COVID-19. International Journal of Molecular Sciences, 23(17), 10060. https://doi.org/10.3390/ijms231710060
- Su, X., Wang, H., Kang, D., Zhu, J., Sun, Q., Li, T., & Ding, K. (2015). Necrostatin-1 ameliorates intracerebral hemorrhage-induced brain injury in mice through inhibiting RIP1/RIP3 pathway. Neurochemical Research, 40(4), 643–650. https://doi.org/10.1007/s11064-014-1510-0
- Tremellen, K. (2008). Oxidative stress and male infertility—a clinical perspective. Human Reproduction Update, 14(3), 243–258. https://doi.org/10.1093/humupd/dmn004
- Vander Borght, M., & Wyns, C. (2018). Fertility and infertility: Definition and epidemiology. Clinical Biochemistry, 62, 2–10. https://doi.org/10.1016/j.clinbiochem.2018.03.012
- Vannuvel, K., Renard, P., Raes, M., & Arnould, T. (2013). Functional and morphological impact of ER stress on mitochondria. Journal of Cellular Physiology, 228(9), 1802–1818. https://doi.org/10.1002/jcp.24360
- Velásquez, A. M. A., Bartlett, P. J., Linares, I. A. P., Passalacqua, T. G., Teodoro, D. D. L., Imamura, K. B., Virgilio, S., Tosi, L. R. O., Leite, A. L., Buzalaf, M. A. R., Velasques, J. M., Netto, A. V. G., Thomas, A. P., & Graminha, M. A. S. (2022). New insights into the mechanism of action of the Cyclopalladated Complex (CP2) in Leishmania: Calcium dysregulation, mitochondrial dysfunction, and cell death. Antimicrobial Agents and Chemotherapy, 66(1), e0076721. https://doi.org/10.1128/aac.00767-21
- Vo, T. S., Ngo, D. H., Bach, L. G., Ngo, D. N., & Kim, S. K. (2017). The free radical scavenging and anti-inflammatory activities of gallate-chitooligosaccharides in human lung epithelial A549 cells. Process Biochemistry, 54, 188–194. https://doi.org/10.1016/j.procbio.2017.01.001
- Wu, X. N., Yang, Z. H., Wang, X. K., Zhang, Y., Wan, H., Song, Y., Chen, X., Shao, J., & Han, J. (2014). Distinct roles of RIP1–RIP3 hetero- and RIP3–RIP3 homo-interaction in mediating necroptosis. Cell Death & Differentiation, 21(11), 1709–1720. https://doi.org/10.1038/cdd.2014.77
- Xia, W., Liu, P., Zhang, J., & Chen, J. (2011). Biological activities of chitosan and chitooligosaccharides. Food Hydrocolloids, 25(2), 170–179. https://doi.org/10.1016/j.foodhyd.2010.03.003
- Xu, Y., Zhang, Q., Yu, S., Yang, Y., & Ding, F. (2011). The protective effects of chitooligosaccharides against glucose deprivation-induced cell apoptosis in cultured cortical neurons through activation of PI3K/Akt and MEK/ERK1/2 pathways. Brain Research, 1375, 49–58. https://doi.org/10.1016/j.brainres.2010.12.029
- Yue, S., Zhou, H., Wang, X., Busuttil, R. W., Kupiec-Weglinski, J. W., & Zhai, Y. (2017). Prolonged ischemia triggers necrotic depletion of tissue-resident macrophages to facilitate inflammatory immune activation in liver ischemia reperfusion injury. Journal of Immunology, 198(9), 3588–3595. https://doi.org/10.4049/jimmunol.1601428
- Zhai, X., Yuan, S., Yang, X., Zou, P., Li, L., Li, G., Shao, Y., Abd El-Aty, A. M., Hacımüftüoğlu, A., & Wang, J. (2019). Chitosan oligosaccharides induce apoptosis in human renal carcinoma via reactive-oxygen-species-dependent endoplasmic reticulum stress. Journal of Agricultural and Food Chemistry, 67(6), 1691–1701. https://doi.org/10.1021/acs.jafc.8b06941
- Zhang, P., Liu, W., Peng, Y., Han, B., & Yang, Y. (2014). Toll like receptor 4 (TLR4) mediates the stimulating activities of chitosan oligosaccharide on macrophages. International Immunopharmacology, 23(1), 254–261. https://doi.org/10.1016/j.intimp.2014.09.007
- Zou, P., Yang, X., Zhang, Y., Du, P., Yuan, S., Yang, D., & Wang, J. (2016). Antitumor effects of orally and intraperitoneally administered Chitosan Oligosaccharides (COSs) on S180-bearing/Residual mouse. Journal of Food Science, 81(12), H3035–h3042. https://doi.org/10.1111/1750-3841.13538