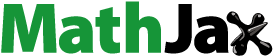
ABSTRACT
This study investigated the inactivation mechanism and kinetics of dielectric barrier discharges (DBD) plasma against Escherichia coli O157:H7. DBD plasma exhibited a remarkable bactericidal effect, and the inactivation kinetics was well described with the Weibull model. Using the ambient air as the working gas, surviving E. coli O157:H7 in phosphate-buffered saline achieved an undetectable level (<10 CFU/mL) after treatment at 18 W for 60 s. DBD plasma altered cell morphology, destructed both the outer and inner membranes, and increased intracellular reactive oxygen species levels. Moreover, DBD plasma significantly decreased the pH of the suspended medium, and increased its oxidation reduction potential and active species levels, which could be mainly responsible for microbial inactivation. The presence of glutathione or N-acetyl-L-cysteine weakened the antibacterial activity of DBD plasma due to their reactive oxygen and nitrogen species (RONS) scavenging capacity, demonstrating that RONS generated by DBD plasma played important roles in decontamination.
1. Introduction
Foodborne diseases are one of the most prominent public health problems worldwide (Rodríguez-Lázaro & Hernandez, Citation2018). Microbial safety is the basis of food safety, and the elimination of microbial contamination in foods is predominant for consumer food safety (WHO, Citation2022). Thermal technology is effective in prolonging the shelf-life of food products, but it is unsuitable for the processing of food ingredients with heat-sensitive nutrients and bioactive compounds (Kubo et al., Citation2023). Nonthermal processing technologies (e.g. ultrasound, supercritical carbon dioxide, cold plasma) have emerged in these years to maintain the original quality of the ingredients while reducing microbial contaminants in processed food. Compared to thermal treatment, non-thermal techniques contribute to creating an equilibrium between microbial safety and minimal processing, which can meet the increased demands of consumers for freshness, flavor, nutrition, and safety (Hernández-Hernández et al., Citation2019).
As one of the emerging non-thermal treatments, cold plasma has been widely investigated in the field of food processing because of many advantages such as low operating temperatures, operation at atmospheric pressure, simple structure, and low power consumption (Dong et al., Citation2021). Plasma is typically generated by employing high voltage to two electrodes until the gap gas is partially or fully ionized, and it consists of various active species, e.g. free radicals, charged particles (electrons and ions), highly reactive neutral species (excited molecules and atoms), and UV photons (López et al., Citation2019). The antimicrobial activity of cold plasma may be closely related to the interaction of ions as well as highly reactive species with cellular biological materials (Gavahian & Khaneghah, Citation2019). The lethal activity of cold plasma to spoilage and pathogenic microbes has also been demonstrated in a variety of liquid and solid food matrixes (apple cider, strawberry, etc.) with minimal impacts on nutritional attributes (Ahmadnia et al., Citation2020; Ozen et al., Citation2022).
Escherichia coli O157:H7 is one of the most virulent foodborne pathogens that can cause health problems in humans worldwide, such as hemorrhagic colitis, hemolytic uremic syndrome, and even death among the young and elderly (Hilborn et al., Citation2000). Serious outbreaks of E. coli O157:H7 infections involved the consumption of various food products including commercial prepackaged cookie dough, leafy greens, milk, beef, and so on (Neil et al., Citation2012). Previous studies have focused on the efficacy of DBD plasma to eliminate E. coli O157:H7 from kinds of food products such as seafood (Choi et al., Citation2022), dried products (Lee & Yoon, Citation2021), and beverages (Ozen et al., Citation2022), and observed the lethal activity on E. coli O157:H7 without significant quality changes. In addition, several studies have adopted the mathematical models to describe the cold plasma-mediated inactivation kinetics of E. coli O157:H7 in diverse food matrixes, such as apple juice and fresh oysters (Choi et al., Citation2022; Liao et al., Citation2018). However, the investigation on the related inactivation mechanism is still limited.
Therefore, to better understand the mechanism underlying microbial inactivation by cold plasma, this work aimed to investigate the cellular changes in DBD plasma-treated E. coli O157:H7 by assessing the structure and function of the cytoplasmic and outer membranes, the transmembrane potential, and the level of intracellular reactive oxygen species (ROS). Furthermore, the changes in the physicochemical characteristics of the liquid medium of E. coli O157:H7 were measured after exposure to DBD plasma. It has been shown that microbial inactivation using cold plasma relies on the production of a diverse mix of reactive oxygen nitrogen species (RONS) with a contribution of UV (López et al., Citation2019). Therefore, this study mainly focused on evaluating the role of RONS generated by DBD plasma in bacterial inactivation through the addition of antioxidants. This study would contribute to promoting the utilization of the DBD plasma technique in food decontamination.
2. Materials and methods
2.1. Materials and chemicals
Agar and tryptic soy broth (TSB) were supplied by Shanghai Maclin Biochemical Co., Ltd. (Shanghai, China). The fluorescence probes (propidium iodide, PI; N-phenyl-1-naphthylamine, NPN), glutathione (GSH), N-acetyl-L-cysteine (NAC), and glutaraldehyde were acquired from Aladdin Scientific Co., Ltd. (Shanghai, China). Ethylenediaminetetraacetic acid (EDTA), 2’,7’-Dichlorodihydrofluorescein diacetate (DCFH-DA), DiBAC4(3), isoamyl acetate, ferrous ammonium sulfate, and other chemicals were obtained from Shanghai Macklin Biochemical Co., Ltd. (China).
2.2. Bacterial culture preparation
E. coli O157:H7 CICC10907 acquired from the China Center of Industrial Culture Collection (Beijing, China) was cultivated in the TSB in a shaker at 37°C overnight, and was collected by centrifugation (6000 × g, 4°C, 10 min). The pellets were then rinsed with sterile saline (0.85% NaCl, w/v) and resuspended in the saline to a concentration of approximately 1.0 × 108 colony-forming unit (CFU)/mL. The viable cell count was estimated by the plating count method after cultivation in TSA media at 37°C for 24 h.
2.3. DBD plasma treatment
The schematic diagram of the DBD plasma device is depicted in . The system was made up of a high-frequency AC power supply (Nanjing Suman Electronic Co., Ltd., Nanjing, China), a voltage regulator, a DBD reactor, and a quartz dish (Dong et al., Citation2021). The discharge voltage and current were detected using an oscilloscope (TDS 1002, Tektronix). The plasma power was calculated using the V-Q Lissajous figure. The ambient air was used as the working gas, and the discharge power was set to 18 W (18 kV, 1.25 A). Bacterial suspension (1 mL, 7–8 log10 CFU/mL) in the quartz dish was exposed to DBD plasma for 20, 40, 50, and 60 s, respectively. After treatment with DBD plasma, collected bacteria cells by centrifugation (12000 × g, 60 s) were resuspended in 0.01 mol/L of sterile phosphate-buffered saline (PBS) (1 mL). The bacteria suspension was plated on the TSB agar (TSA) media and then cultivated in a thermostatic incubator at 37°C for 2 d. The survival of E. coli O157:H7 was determined by the number of colonies and expressed as log10 CFU/mL, and the detection limit was 10 CFU/mL.
2.4. Modeling of survival curve
Survival data of E. coli O157:H7 after DBD plasma treatment were fitted to the Weibull model with the Origin 2017 software version 9.4.2.380 (OriginLab, Northampton, MA). The equation for the Weibull model is as follows (Bai et al., Citation2020):
where Nt is the number of surviving cells at a given time (CFU/mL), N0 is the initial number of E. coli O157:H7 (CFU/mL), t is the plasma exposure time (s), a is a scale parameter, and b is a shape parameter. The b represents the degree of curvilinearity, with b < 1, b = 1, and b > 1 corresponding to concave-upwards (tailings), linear, and concave-downwards (shoulders) survival curves, respectively.
The accuracy of the fitted model was evaluated according to the R2 coefficient given by Origin 2017 and the root mean square errors (RMSE) given below:
where Z1 is the experimentally obtained number of surviving cells (log10 CFU/mL), Z2 is the predicted number of surviving cells with the model, and n is the number of experimental groups.
2.5. Field emission-scanning electron microscopy (FE-SEM)
An FE-SEM was applied to observe the influence of DBD plasma on the morphology of E. coli O157:H7 cells (Xiang et al., Citation2019). In brief, after being treated with DBD plasma (18 W) for 0, 20, 40, and 60 s, respectively, bacteria cells were fixed with 500 μL of 3% glutaraldehyde solution at 4°C for 4 h. Then the cells were washed twice with sterile PBS (0.1 mol/L, pH 7.2). After dehydration with gradient ethanol (10–100%, v/v), the cells were dried overnight and sputter-coated using the gold. E. coli O157:H7 cells with or without treatment were finally observed by using an FE-SEM (Regulus8100, Hitachi High-Tech Corp., Tokyo, Japan) operated at 3.0 kV.
2.6. Measurement of intracellular materials leakage
Intracellular materials leaked from E. coli O157:H7 cells with or without DBD plasma treatment were measured according to a method described by Liu et al. (Citation2021). DBD plasma with a power of 18 W was employed to treat E. coli O157:H7 for various durations (20, 40, 50, and 60 s). The supernatants collected by centrifugation at 10,000 × g for 2 min at 4°C were used to measure the release of intracellular materials. The heat-shocked E. coli cells at 55°C for 15 min was used as the positive controls, the cell membranes of which were considered completely disrupted. The content of UV-absorbing materials leaked from the bacterial cells was determined by detecting the absorbance of the supernatant at 280 and 260 nm, respectively, with a spectrophotometer (NanoDrop 2000, Thermo Scientific, Wilmington, DE, U.S.A.). The intracellular materials leakage was expressed as a ratio to that of the positive controls.
2.7. Assessment of cell membrane damage
E. coli O157:H7 cells were exposed to DBD plasma with a power of 18 W for 20, 40, 50, and 60 s, respectively. The untreated bacterial cells were used as the control.
2.7.1. Assay of the permeability of the outer membrane
The permeability of the outer membrane of E. coli O157:H7 cells was evaluated with the NPN probe (Xiang et al., Citation2019). The collected E. coli O157:H7 cells before and after DBD plasma treatment were suspended in a HEPES solution (1.0 mL, 5 mol/L, pH 7.2). Subsequently, the NPN solution (the final concentration was 10 μmol/L) was added to the bacteria suspension and mixed thoroughly. The mixture was then incubated for 10 min at room temperature. After centrifugation, the cell pellets were rinsed twice with saline and resuspended in the HEPES solution. A multi-mode microplate reader (Spark 20 M, Tecan, Männedorf, Switzerland) was used to measure the fluorescence intensity of NPN at an excitation wavelength of 350 nm and an emission wavelength of 420 nm. The NPN uptake was calculated using the following equation:
where F1 is the measured fluorescence intensity of the untreated bacteria cells, and F2 is the measured fluorescence intensity of DBD plasma-treated cells.
2.7.2. Assay of the permeability of cytoplasmic membrane
The permeability of the cytoplasmic (or inner) membrane of E. coli O157:H7 was detected by PI staining (Niu et al., Citation2022). Briefly, following the treatment with DBD plasma, the PI solution (the final concentration was 3.0 μmol/L) was added to the bacterial suspension and fully mixed, which was incubated in the dark at room temperature for 15 min. The cells were harvested, washed twice with sterile PBS, and resuspended in the same solution. Photomicrographs were acquired using a fluorescent microscope (Eclipse 80i, Nikon, Tokyo, Japan). Meanwhile, the fluorescence intensity was measured at λex/λem of 540 nm/630 nm using a multi-mode microplate reader. The data were expressed in terms of the relative fluorescence intensity of PI to that of the control (100%).
2.7.3. Assessment of cell membrane potential
After exposure to DBD plasma, alteration of the cell membrane potential of E. coli O157:H7 was investigated using the DiBAC4(3) probe (Kim et al., Citation2017). Briefly, following exposure to DBD plasma, the cells were washed, resuspended, and incubated with DiBAC4(3) solution (the final concentration was 0.5 µg/mL) for 15 min at 37°C in the dark. Then, the cells collected by centrifugation were rinsed twice and suspended in PBS. Finally, the fluorescence intensity at λex/λem of 488 nm/525 nm was measured by using a multi-mode microplate reader. The data were expressed in terms of the relative fluorescence intensity of DiBAC4(3) to that of the control (100%).
2.8. Physicochemical properties analysis of DBD plasma-treated saline
After DBD plasma treatment, an immediate evaluation was conducted on the physicochemical characteristics of 0.85% sterile saline. The pH, conductivity, and oxidation reduction potential (ORP) of the saline were determined with a pH meter (Mettler-Toledo, Switzerland), a conductivity meter (INESA Scientific Instrument Co., Ltd, Shanghai, China), and a rechargeable ORP composite electrode (Leici, Shanghai, China), respectively. The concentration of H2O2 was measured using a hydrogen peroxide quantitative detection kit (Sangon Biotech Co., Ltd., Shanghai, China). The level of NO3– and NO2– was determined using a nitrite/nitrate test kit (Beyotime Biotechnology Research Institute, Shanghai, China).
2.9. Analysis of intracellular reactive oxygen species (ROS) levels
The intracellular ROS level was measured with a DCFH-DA probe as described by Pan et al. (Citation2019). Briefly, DBD plasma-treated cells were harvested, washed, and incubated with DCFH-DA at a final concentration of 50 μmol/L in the dark at 37°C for 20 min. Afterward, E. coli O157:H7 cells were thoroughly washed twice in sterile PBS, and the fluorescence intensity was measured with a Tecan Spark 20 M plate reader (λex/λem = 480 nm/525 nm). The data were represented as the relative fluorescence intensity of DCFH-DA to that of the control (100%).
2.10. Effect of exogenous antioxidants on bacterial inactivation by DBD plasma
The impact of ROS generated by DBD plasma on E. coli O157:H7 inactivation was investigated by adding the antioxidant (GSH or NAC) (Zhao et al., Citation2022). The cell suspensions with or without exogenous antioxidants at 10 mmol/L were subjected to DBD plasma at 18 W for 60 s. Subsequently, plate-counting was performed to record the population of bacterial survivals. In the presence of an antioxidant, E. coli O157:H7 cells not exposed to DBD plasma were used as a control to monitor the effect of the antioxidant on cell viability.
2.11. Statistical analysis
Each test was conducted in triplicate and repeated three times independently. The results were expressed as mean ± standard deviation. The significance was analyzed by using one-way analysis of variance (ANOVA) followed by the least significant difference (LSD) post-hoc test at p < .05. All data were analyzed using SPSS for Windows version 22.0 (SPSS, Chicago, IL, U.S.A.).
3. Results and discussion
3.1. Lethal effect of DBD plasma on E. coli O157:H7
The survival curve of E. coli O157:H7 after exposure to DBD plasma is presented in . No significant reduction of cell viability was observed after 20 s of exposure to DBD plasma at 18 W. However, the surviving cell counts of E. coli O157:H7 rapidly declined when prolonged the process time over 20 s. After DBD plasma treatment for 40 and 50 s, E. coli O157:H7 significantly decreased (p < .05) by 1.26 ± 0.12 and 4.02 ± 0.14 log10 CFU/mL, respectively, at initial population of 7.33 ± 0.05 log10 CFU/mL, and the number of E. coli O157:H7 survivals with plasma treatment for 60 s reached to an undetectable level (<10 CFU/mL).
Figure 2. Inactivation curve of DBD plasma-treated E. coli O157:H7 at a power of 18 W. Error bars represent standard deviation for three repetitions. Means with different letters are different significantly at p = .05 according to the LSD test.
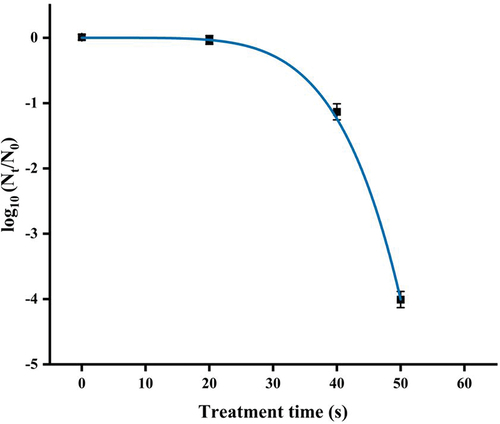
In this study, the survival data of E. coli O157:H7 treated by DBD plasma were fitted to the Weibull model (). The R2 of over 0.999 and low RMSE of 0.071 proved the goodness-of-fit and the suitability of the Weibull model. The scaling parameter a obtained from the Weibull model was 4.61 ± 0.00, which was related to the rate of microbial inactivation. The shape parameter (b value) was greater than 1.0 (b = 5.26 ± 0.05), hence permitted a shoulder effect, suggesting that the survival curve displayed a downward concavity. Remaining population with accumulated injury makes the cells progressively susceptible to lethal treatment, which may explain this downward concave shape. Weibull models have been successfully used to describe the survival curves of microbial inactivation by cold plasma in previous studies. For example, Liao et al. (Citation2018) and Pan et al. (Citation2019) reported that the survival curves of E. coli and Listeria monocytogenes treated with cold plasma successfully described with the Weibull model also appeared downward concavity.
3.2. Morphological changes of DBD plasma-treated E. coli O157:H7 cells
shows the morphological changes of E. coli O157:H7 cells with and without DBD plasma treatment. As shown in , the untreated bacterial cells exhibited an intact structure with a smooth surface. Further, no obvious morphological change in E. coli O157:H7 cells was found after being treated with DBD plasma for 20 s (). However, plasma treatment for 40 s resulted in cell morphological changes such as deformation and holes, indicating that DBD plasma caused damage to the cell envelope (). When extending the treatment time to 60 s, the change in bacterial morphology became more serious (). The shrunken and deformed E. coli O157:H7 cells were more than those presented in , and the bacterial cells were even destructed by DBD plasma treatment for 60 s, which could lead to the leakage of cellular contents from the cell interior. The FE-SEM images showed that the severity of morphological changes of E. coli O157:H7 varied with the treatment time. The stronger the antibacterial action (), the more serious the morphological changes. Similarly, Ozen et al. (Citation2022) found that the shape of E. coli O157:H7 in apple cider was markedly changed by plasma jets for 120 s. Treatment with DBD plasma for 100 s also led to severe morphological destruction in Salmonella Typhimurium cells (Huang et al., Citation2019).
3.3. Alteration of the cell membrane permeability of DBD plasma-treated E. coli O157:H7
The cell membrane is one vital part of microorganisms that separates and protects the cell from the external environment. The disruption of the cell membrane directly influences cell viability. As shown in , the content of extracellular UV-absorbing materials at 260 nm of E. coli O157:H7 significantly increased from 5.00 µg/mL to 16.93 ± 0.08, 25.07 ± 0.09, 31.50 ± 0.11, and 40.43 ± 0.15 µg/mL, respectively (p < .05), after being treated by DBD plasma for 20, 40, 50, and 60 s. The level of extracellular UV-absorbing materials at 280 nm was also dependent on the process time (), which was significantly enhanced by 7.00 ± 1.00 ~ 84.00 ± 4.17 µg/mL (p < .05), after DBD plasma treatment for 20 ~ 60 s. The value of OD280 significantly increased with the extension of the treatment time. The results suggested that treatment with DBD plasma resulted in the permeabilization of E. coli O157:H7 cell membrane. This could be attributed to the destruction of the cell envelope (). The increase in membrane permeability with the extension of treatment time was also consistent with the inactivation of E. coli O157:H7 by DBD plasma (). Similar results reported by Miao and Yun (Citation2011) showed that DBD plasma caused the leakage of protein from E. coli according to the Coomassie brilliant blue staining.
Figure 4. Relative level of extracellular UV-absorbing materials at 260 nm (a) and 280 nm (b) of E. coli O157:H7 with or without exposure to DBD plasma. Error bars represent standard deviation for three repetitions. Means with the same letters are not significantly different according to the LSD test at p = .05.
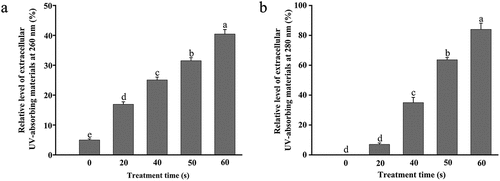
The cell envelope of Gram-negative bacteria comprises the outer membrane and the cytoplasmic (or inner) membrane (Beveridge, Citation1999). To further clarify the influence of DBD plasma on the cell membrane of E. coli O157:H7, the changes in the permeability of two-layer membranes were also investigated. Concerning the outer membrane, it consists of lipopolysaccharides and phospholipids and commonly acts as a barrier to prevent the injury to bacterial cells from harmful factors outside (Dong et al., Citation2016). NPN is a hydrophobic fluorescent probe that exhibits stronger fluorescence in a hydrophobic environment than in an aqueous environment. Normally, the outer membrane can largely exclude the NPN from the cytoplasmic interior, while the NPN uptake will increase with the enhancement of the permeability of the outer membrane (Xiang et al., Citation2019). As shown in , DBD plasma-treated cells showed a time-dependent increase in the fluorescence intensity of NPN. The fluorescence intensity of NPN in E. coli O157:H7 was remarkably raised (p < .05) by 146.58 ± 19.93%, 262.54 ± 16.21%, 315.56 ± 17.42%, and 405.38 ± 9.37% after plasma treatment for 20, 40, 50, and 60 s, respectively, relative to the control (100%). The increase in the NPN uptake in E. coli O157:H7 suggested the permeabilization of the outer membrane induced by DBD plasma. The outer membrane damage would make the bacterial cells more susceptible to environment stress (Li et al., Citation2016), which could be one of the main mechanisms for E. coli O157:H7 inactivation by DBD plasma.
Figure 5. Alteration of the cell membrane permeability of DBD plasma-treated and untreated E. coli O157:H7 cells. (a) Relative fluorescence intensity of NPN (%); (b) relative fluorescence intensity of PI (%); (c) fluorescence microscopic images of E. coli O157:H7 stained with PI (400 ×). Error bars mean standard deviation for three repetitions. Means labeled with the same letters are not significantly different according to the LSD test at p = .05.
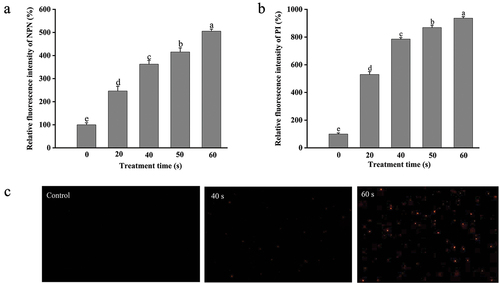
The inner membrane permeability was evaluated using the PI probe that can pass through the cells with destructive inner membrane and bind with the nucleic acids to emit red fluorescence (Gan et al., Citation2021). As exhibited in , after DBD plasma treatment the PI fluorescence intensity in E. coli O157:H7 was significantly increased (p < .05). Compared to that of the untreated group, the plasma treatment for 20–60 s led to an increase of 5.30–8.36-fold in the PI uptake. Moreover, the PI uptake in bacterial cells was also visually observed by using a fluorescence microscope (). The PI-positive E. coli cells were hardly found in the untreated group, while the population of bacteria cells with a red fluorescence significantly enhanced after exposure to DBD plasma. The microscopic images were consistent with the relative values of PI uptake. These results suggested the injury to the inner membrane permeability of E. coli O157:H7 induced by the DBD plasma.
3.4. Alteration of the cell membrane potential of DBD plasma-treated E. coli O157:H7
The alteration of the cell membrane potential has a critical effect on cellular basic physiological functions, such as pH homeostasis, ATP synthesis, membrane translocation, flagellar motility, and so on (Benarroch & Asally, Citation2020). DiBAC4(3), a voltage-sensitive fluorescent probe, can pass through the depolarized cell membrane, bind to the intracellular proteins, and emit strong fluorescence, which is conducive to evaluate changes in the cell membrane potential (Kim et al., Citation2017). shows the changes in the fluorescence intensity of DiBAC4(3) after different treatments. The fluorescence intensity in E. coli O157:H7 without treatment was set as 100%. After exposure to DBD plasma at 18 W for 20, 40, 50, and 60 s, the DiBAC4(3) accumulation in E. coli O157:H7 was remarkably increased (p < .05) by 1.88-, 4.87-, 5.94-, and 6.84-fold, respectively, relative to that of the control. Accumulation of the DiBAC4(3) probe in bacterial cells meant the occurrence of membrane depolarization induced by DBD plasma.
Figure 6. Alteration of the cell membrane potential of DBD plasma-treated E. coli O157:H7. Error bars mean standard deviation for three repetitions. Different letters above the bars represent significant differences among treatment groups according to the LSD test at p = .05.
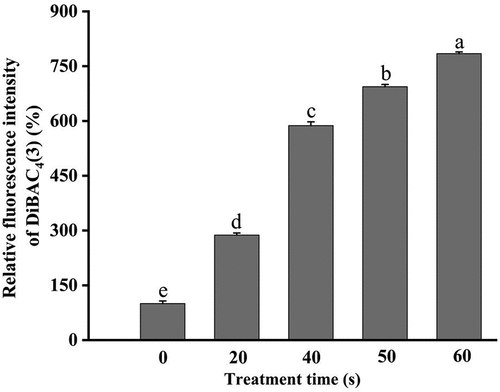
Combined with the observations of cell morphology and cell membranes mentioned above, the structure and function of the cell membranes of E. coli O157:H7 were severely destructed after being treated by DBD plasma. This could be associated with the changes in the physiochemical characteristics of bacteria suspension during the DBD plasma treatment, such as pH, conductivity, and the generation of reactive species, which was analyzed in the subsequent section.
3.5. Changes in the physicochemical properties of the suspended medium after DBD plasma treatment
The influence of DBD plasma treatment on the physiochemical properties of the background solution was analyzed in this study, including pH, ORP, conductivity, and reactive species. As displayed in , the pH of sterile saline was significantly reduced (p < .05) from 6.26 to 3.11 after DBD plasma treatment with a discharge power of 18 W for 60 s, which was coincident with the previous study (Su et al., Citation2018). The variation trend of the pH appropriately reflected the sterilization process of DBD plasma. This could be attributed to the formation of RNS during the plasma discharge, which could further produce nitric acids, leading to increased acidity of the aqueous solution (Lukes et al., Citation2014). Reduced pH of the solution could dramatically affect the bactericidal activity of cold plasma (Yokoyama et al., Citation2019). It has been revealed that at pH < 4.8, the superoxide anion radicals (O2−∙) generated by plasma discharge convert to electrically neutral hydroperoxyl radicals (HOO∙), which easily penetrates through the cell membrane, and acts with higher antibacterial activity (Takai et al., Citation2013). Meanwhile, following treatment with DBD plasma, the values of ORP and conductivity of saline increased notably over time (p < .05) and an increase of about 232 mV and 462 μS/cm was achieved after 60 s of exposure to DBD plasma. The ORP and conductivity are involved in the amounts and activity of the generated highly reactive species; consequently, an increase in the values of ORP and conductivity suggests the enhanced oxidation effect on substances (Zhang et al., Citation2013).
Table 1. The pH, ORP, and conductivity of DBD plasma-treated saline.
It has been proven that stable and long-lived reactive oxygen and nitrogen species (RONS) are formed and concentrated when the discharged air comes into contact with water (Dong et al., Citation2021). H2O2 is one of the representative ROS and mainly comes from H2O2 in plasma air as well as the recombination of hydroxyl radicals (∙OH) dissolved in water from plasma air (Ikai et al., Citation2010). The concentration of H2O2 in the saline solution treated by DBD plasma was found to increase with the process time increasing (). A similar pattern was also observed in the concentration of nitrate and nitrite ions (NO3— and NO2—), which demonstrated that the reactive nitrogen species (RNS) were generated by DBD plasma in a time-dependent manner (Mai-Prochnow et al., Citation2021). RONS generated in the bacterial suspensions could oxidize the membrane-binding proteins and membrane lipids, leading to the injury of the outer membrane, and, in turn, the inner membrane of E. coli O157:H7, i.e. loss of membrane stability, which could explain the increased membrane permeability observed in and the membrane depolarization shown in (Farrugia & Balzan, Citation2012). Generation of NO3− and NO2− from the dissolution of NOX was also the reason for the decrease in pH of the plasma-treated saline. Therefore, the interaction between the antibacterial activity of RONS and the acidic environment are mainly responsible for the microbial inactivation by DBD plasma.
Table 2. Contents of H2O2, NO3−, and NO2− in DBD plasma-treated saline.
3.6. Changes in the intracellular ROS level of DBD plasma-treated E. coli O157:H7
Intracellular ROS at low concentrations usually come from the by-products of cell aerobic metabolism and play crucial roles in microbial signal transduction and maintenance of intracellular homeostasis in biological cells (Liu et al., Citation2021). However, when the amount of intracellular ROS increases to a certain extent that exceeds the scavenging capacity of the cell, cellular oxidative damage will be induced (Kong et al., Citation2019). Due to the increased membrane permeability of E. coli O157:H7 with plasma treatment, the extracellular generated RONS could move across the cell membrane and accumulate in the interior of the bacterial cell. Furthermore, intracellular active species could induce cascade reactions to produce more ROS. For example, O2−∙ can be transformed into H2O2 through dismutation, or HOO∙ through protonation, and the latter can further react with polyunsaturated fatty acyl groups forming fatty acyl radicals (Jastroch et al., Citation2010). Therefore, it was necessary to analyze the changes in the intracellular ROS level of DBD plasma-treated E. coli O157:H7. DCFH-DA is a redox-sensitive fluorescent probe that can freely penetrate the cell membrane and be used to monitor the intracellular ROS level. As depicted in , the fluorescence intensity of DCFH-DA remarkably enhanced with the extension of treatment time, demonstrating the enhancement of the ROS amount in E. coli O157:H7 induced by DBD Plasma. DBD plasma treatment for 20, 40, 50, and 60 s significantly increased (p < .05) the intracellular ROS level in E. coli O157:H7 cells by 105.52 ± 18.34%, 295.11 ± 15.52%, 371.55 ± 10.91%, and 743.95 ± 26.48%, respectively, relative to that in the control cells. Similar results can be visually observed by using a fluorescent microscope (). A similar pattern was also found in the intracellular ROS in E. coli O157:H7 induced by plasma-activated water (Jyung et al., Citation2022). Intracellular ROS accumulation can cause oxidative damage to DNA, proteins, and membrane lipids in the cell, therefore hindering the cell normal metabolic activities (Farrugia & Balzan, Citation2012), which was generally in line with the results of E. coli inactivation by DBD plasma ().
Figure 7. Changes in the intracellular ROS level of DBD plasma-treated E. coli O157:H7. (a) Relative levels of intracellular ROS; (b) fluorescence microscopic images of E. coli O157:H7 dyed with DCFH-DA (400 ×). Error bars represent standard deviation for three repetitions. Different letters above the bars represent significant differences among treatment groups according to the LSD test at p = .05.
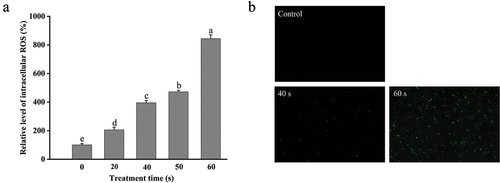
3.7 Role of ROS generated by DBD plasma in E. coli O157:H7 inactivation
In this study, the role of ROS generated by DBD plasma in microbial inactivation was analyzed since there was a good correlation between the ROS level in the background solution and cell inactivation. An exogenous antioxidant (GSH or NAC) was added to the bacteria suspension before the DBD plasma treatment to scavenge the ROS generated in the suspended medium during the plasma discharge. As shown in , GSH or NAC alone had no effect on the survival of E. coli O157:H7 cells (p > .05), while its addition greatly weakened the antibacterial effect of DBD plasma treatment on E. coli O157:H7. After treatment at a discharge power of 18 W for 60 s, the number of surviving cells without any antioxidant fell from 7.67 ± 0.12 log10 CFU/mL to undetectable. In the presence of GSH or NAC in the bacterial suspension, the population of E. coli survivals was only reduced by 2.57 ± 0.20 and 2.37 ± 0.14 log10 CFU/mL (p < .05) after exposure to DBD plasma, respectively. GSH and its precursor, NAC, are powerful antioxidants that can scavenge ROS and prevent oxidative stress damage (Clough, Citation2014; Niu et al., Citation2021). The reduction of bactericidal activity of DBD plasma treatment against E. coli O157:H7 was positively correlated to the decrease in the ROS level in the bacterial suspension with the addition of antioxidants. This demonstrated that the ROS in the background solution generated by DBD plasma played an important role in the plasma disinfection.
4. Conclusion
DBD plasma effectively reduced the number of surviving cells of E. coli O157:H7 in phosphate-buffered saline, and the kinetics of the survival curve was well described by the Weibull model. Through analysis of the impacts of exogenous antioxidants on the plasma sterilization, it was confirmed that the RONS produced by DBD plasma played significant roles in the inactivation process against E. coli O157:H7. DBD plasma-generated chemical species could eventually disrupt the normal life activities of bacterial cells by passing through or attacking the cell envelope. Both the outer and inner membranes of E. coli O157:H7 were structurally and functionally destructed by DBD plasma treatment, which was accompanied by the leakage of intracellular contents. This study preliminarily revealed the cellular targets of DBD plasma action on E. coli O157:H7, which provided a theoretical reference for the development of combined sterilization technology. Results obtained in the present study could be useful for assisting design, evaluation, and optimization of DBD plasma processing for the liquid food industry. To obtain more insights into the bactericidal mechanism against pathogenic microbes, in the future study, the other action targets (e.g. enzymes, organelles, nucleic acids, pH homeostasis) of E. coli O157:H7 by DBD plasma should be also evaluated, and the changes at the molecular level induced by DBD plasma remain to be further explored.
Acknowledgments
The authors would like to acknowledge the contribution of the National Natural Science Foundation of China (NSFC).
Disclosure statement
No potential conflict of interest was reported by the author(s).
Additional information
Funding
References
- Ahmadnia, M. I., Sadeghi, M., Abbaszadeh, R., & Marzdashti, H. R. G. (2020). Decontamination of whole strawberry via dielectric barrier discharge cold plasma and effects on quality attributes. Journal of Food Processing and Preservation, 45(1), 15019. https://doi.org/10.1111/jfpp.15019
- Bai, Y., Muhammad, A. I., Hu, Y. Q., Koseki, S., Liao, X. Y., Chen, S., Ye, X. Q., Liu, D. H., & Ding, T. (2020). Inactivation kinetics of Bacillus cereus spores by plasma activated water (PAW). Food Research International, 131, 109041. https://doi.org/10.1016/j.foodres.2020.109041
- Benarroch, J. M., & Asally, M. (2020). The microbiologist’s guide to membrane potential dynamics. Trends in Microbiology, 28(4), 304–11. https://doi.org/10.1016/j.tim.2019.12.008
- Beveridge, T. J. (1999). Structures of gram-negative cell walls and their derived membrane vesicles. Journal of Bacteriology, 181(16), 4725–4733. https://doi.org/10.1128/jb.181.16.4725-4733.1999
- Choi, M. S., Jeon, E. B., Kim, J. Y., Choi, E. H., Lim, J. S., Choi, J., & Park, S. Y. (2022). Application of dielectric barrier discharge plasma for the reduction of non-pathogenic Escherichia coli and E. coli O157: H7 and the quality stability of fresh oysters (Crassostrea gigas). LWT-Food Science and Technology, 154, 112698. https://doi.org/10.1016/j.lwt.2021.112698
- Clough, S. R. (2014). Cysteine, N-Acetyl-l. Encyclopedia of Toxicology, 1122–1124. https://doi.org/10.1016/b978-0-12-386454-3.00485-1
- Dong, S. S., Fan, L. M., Ma, Y. F., Du, J., & Xiang, Q. S. (2021). Inactivation of polyphenol oxidase by dielectric barrier discharge (DBD) plasma: Kinetics and mechanisms. LWT-Food Science and Technology, 145, 111322. https://doi.org/10.1016/j.lwt.2021.111322
- Dong, H. H., Zhang, Z. Y., Tang, X. D., Huang, S. H., Li, H. Y., Peng, B., & Dong, C. J. (2016). Structural insights into cardiolipin transfer from the inner membrane to the outer membrane by PbgA in gram-negative bacteria. Scientific Reports, 6(1), 30815. https://doi.org/10.1038/srep30815
- Farrugia, G., & Balzan, R. (2012). Oxidative stress and programmed cell death in yeast. Frontiers in Oncology, 2, 64. https://doi.org/10.3389/fonc.2012.00064
- Gan, Z. L., Feng, X. R., Hou, Y. N., Sun, A. D., & Wang, R. X. (2021). Cold plasma jet with dielectric barrier configuration: Investigating its effect on the cell membrane of E. coli and S. cerevisiae and its impact on the quality of chokeberry juice. LWT-Food Science and Technology, 136, 110223. https://doi.org/10.1016/j.lwt.2020.110223
- Gavahian, M., & Khaneghah, A. M. (2019). Cold plasma as a tool for the elimination of food contaminants: Recent advances and future trends. Comprehensive Reviews in Food Science and Food Safety, 60(9), 1581–1592. https://doi.org/10.1080/10408398.2019.1584600
- Hernández-Hernández, H. M., Moreno-Vilet, L., & Villanueva-Rodríguez, S. J. (2019). Current status of emerging food processing technologies in Latin America: Novel non-thermal processing. Innovative Food Science & Emerging Technologies, 58, 102233. https://doi.org/10.1016/j.ifset.2019.102233
- Hilborn, E. D., Mshar, P. A., Fiorentino, T. R., Dembek, Z. F., Barrett, T. J., Howard, R. T., & Cartter, M. L. (2000). An outbreak of Escherichia coli O157: H7 infections and haemolytic uraemic syndrome associated with consumption of unpasteurized apple cider. Epidemiology & Infection, 124(1), 31–36. https://doi.org/10.1017/S0950268899003258
- Huang, M. M., Zhuang, H., Zhao, J. Y., Wang, J. M., Yan, W. J., & Zhang, J. H. (2019). Differences in cellular damage induced by dielectric barrier discharge plasma between salmonella typhimurium and staphylococcus aureus. Bioelectrochemistry, 132, 107445. https://doi.org/10.1016/j.bioelechem.2019.107445
- Ikai, H., Nakamura, K., Shirato, M., Kanno, T., Iwasawa, A., Sasaki, K., Niwano, Y., & Kohno, M. (2010). Photolysis of hydrogen peroxide, an effective disinfection system via hydroxyl radical formation. Antimicrobial Agents and Chemotherapy, 54(12), 5086–5091. https://doi.org/10.1128/AAC.00751-10
- Jastroch, M., Divakaruni, A. S., Mookerjee, S., Treberg, J. R., Brand, M. D., Brown, G. C., & Murphy, M. P. (2010). Mitochondrial proton and electron leaks. Essays in Biochemistry, 47, 53–67. https://doi.org/10.1042/BSE0470053
- Jyung, S., Kang, J. W., & Kang, D. H. (2022). L. monocytogens exhibited less cell membrane damage, lipid peroxidation, and intracellular reactive oxygen species accumulation after plasma-activated water treatment compared to E. coli O157:H7 and S. Typhimurium. Food Microbiology, 108, 104098. https://doi.org/10.1016/j.fm.2022.104098
- Kim, D. K., Kim, S. J., & Kang, D. H. (2017). Bactericidal effect of 266 to 279 nm wavelength UVC-LEDs for inactivation of gram positive and gram negative foodborne pathogenic bacteria and yeasts. Food Research International, 97, 280–287. https://doi.org/10.1016/j.foodres.2017.04.009
- Kong, J., Zhang, Y., Ju, J., Xie, Y. F., Guo, Y. H., Cheng, Y. L., Qian, H., Quek, S. Y., & Yao, W. R. (2019). Antifungal effects of thymol and salicylic acid on cell membrane and mitochondria of rhizopus stolonifer and their application in postharvest preservation of tomatoes. Food Chemistry, 285, 380–388. https://doi.org/10.1016/j.foodchem.2019.01.099
- Kubo, M. T. K., Baicu, A., Erdogdu, F., Poças, M. F., Silva, C. L. M., Simpson, R., Vitali, A. A., & Augusto, P. E. D. (2023). Thermal processing of food: Challenges, innovations, and opportunities. A position paper. Food Reviews International, 39(6), 3344–3369. https://doi.org/10.1080/87559129.2021.2012789
- Lee, Y. J., & Yoon, K. S. (2021). Inactivating effect of dielectric barrier discharge plasma on Escherichia coli O157: H7 and staphylococcus aureus in various dried products. Journal of Food Safety, 41(6), e12940. https://doi.org/10.1111/jfs.12940
- Li, J., Ahn, J., Liu, D. H., Chen, S. G., Ye, X., & Ding, T. (2016). Evaluation of ultrasound-induced damage to Escherichia coli and staphylococcus aureus by flow cytometry and transmission electron microscopy. Applied and Environmental Microbiology, 82(6), 1828–1837. https://doi.org/10.1128/AEM.03080-15
- Liao, X. Y., Li, J., Muhammad, A. I., Suo, Y. J., Chen, S. G., Ye, X. Q., Liu, D. H., & Ding, T. (2018). Application of a dielectric barrier discharge atmospheric cold plasma (Dbd‐Acp) for Escherichia coli inactivation in apple juice. Journal of Food Science, 83(2), 401–408. https://doi.org/10.1111/1750-3841.14045
- Liu, X., Li, Y. F., Wang, S. D., Huangfu, L. L., Zhang, M. Y., & Xiang, Q. S. (2021). Synergistic antimicrobial activity of plasma-activated water and propylparaben: Mechanism and applications for fresh produce sanitation. LWT-Food Science and Technology, 146, 111447. https://doi.org/10.1016/j.lwt.2021.111447
- López, M., Calvo, T., Prieto, M., Múgica-Vidal, R., Muro-Fraguas, I., Alba-Elias, F., & Alvarez-Ordonez, A. (2019). A review on non-thermal atmospheric plasma for food preservation: Mode of action, determinants of effectiveness, and applications. Frontiers in Microbiology, 10, 622. https://doi.org/10.3389/fmicb.2019.00622
- Lukes, P., Dolezalova, E., Sisrova, I., & Clupek, M. (2014). Aqueous-phase chemistry and bactericidal effects from an air discharge plasma in contact with water: Evidence for the formation of peroxynitrite through a pseudo-second-order post-discharge reaction of H2O2 and HNO2. Plasma Sources Science and Technology, 23(1), 015019. https://doi.org/10.1088/0963-0252/23/1/015019
- Mai-Prochnow, A., Zhou, R., Zhang, T., Ostrikov, K., Mugunthan, S., Rice, S. A., & Cullen, P. J. (2021). Interactions of plasma-activated water with biofilms: Inactivation, dispersal effects and mechanisms of action. Npj Biofilms and Microbiomes, 7(1), 11. https://doi.org/10.1038/s41522-020-00180-6
- Miao, H., & Yun, G. (2011). The sterilization of Escherichia coli by dielectric-barrier discharge plasma at atmospheric pressure. Applied Surface Science, 257(16), 7065–7070. https://doi.org/10.1016/j.apsusc.2011.03.014
- Neil, K. P., Biggerstaff, G., MacDonald, J. K., Trees, E., Medus, C., Musser, K. A., Stroika, S. G., Zink, D., & Sotir, M. J. (2012). A novel vehicle for transmission of Escherichia coli O157: H7 to humans: Multistate outbreak of E. coli O157: H7 infections associated with consumption of ready-to-bake commercial prepackaged cookie dough—United States, 2009. Clinical Infectious Diseases, 54(4), 511–518. https://doi.org/10.1093/cid/cir831
- Niu, B., Liao, K., Zhou, Y. X., Wen, T., Quan, G. L., Pan, X., & Wu, C. C. (2021). Application of glutathione depletion in cancer therapy: Enhanced ROS-based therapy, ferroptosis, and chemotherapy. Biomaterials, 277, 121110. https://doi.org/10.1016/j.biomaterials.2021.121110
- Niu, L. Y., Zhang, Y. L., Jie, M. S., Cheng, Y. X., Xiang, Q. S., Zhang, Z. J., & Bai, Y. H. (2022). Synergetic effect of petit-high pressure carbon dioxide combined with cinnamon (Cinnamomum cassia) essential oil against salmonella typhimurium. International Journal of Food Science and Technology, 57(5), 2954–2967. https://doi.org/10.1111/ijfs.15613
- Ozen, E., Kumar, G. D., Mishra, A., & Singh, R. K. (2022). Inactivation of Escherichia coli in apple cider using atmospheric cold plasma. International Journal of Food Microbiology, 382, 109913. https://doi.org/10.1016/j.ijfoodmicro.2022.109913
- Pan, Y. Y., Cheng, J. H., Lv, X. Y., & Sun, D. W. (2019). Assessing the inactivation efficiency of Ar/O2 plasma treatment against listeria monocytogenes cells: Sublethal injury and inactivation kinetics. LWT-Food Science and Technology, 111, 318–327. https://doi.org/10.1016/j.lwt.2019.05.041
- Rodríguez-Lázaro, D., & Hernandez, M. (2018). Emerging biological risks in a global context: An introduction. Advances in Food and Nutrition Research, 86, 1–12. https://doi.org/10.1016/bs.afnr.2018.04.001
- Su, X., Tian, Y., Zhou, H. Z., Li, Y. L., Zhang, Z. H., Jiang, B. Y., Yang, B., Zhang, J. Y., Fang, J., & Schaffner, D. W. (2018). Inactivation efficacy of nonthermal plasma-activated solutions against Newcastle disease virus. Applied and Environmental Microbiology, 84(9), e02836–17. https://doi.org/10.1128/AEM.02836-17
- Takai, E., Ikawa, S., Kitano, K., Kuwabara, J., & Shiraki, K. (2013). Molecular mechanism of plasma sterilization in solution with the reduced pH method: Importance of permeation of HOO radicals into the cell membrane. Journal of Physics D: Applied Physics, 46(29), 295402. https://doi.org/10.1088/0022-3727/46/29/295402
- WHO. (2022). World Health Organization. Retrieved, from https://www.who.int/news-room/fact-sheets/detail/food-safety
- Xiang, Q. S., Wang, W. J., Zhao, D. B., Niu, L. Y., Li, K., & Bai, Y. H. (2019). Synergistic inactivation of Escherichia coli O157: H7 by plasma-activated water and mild heat. Food Control, 106, 106741. https://doi.org/10.1016/j.foodcont.2019.106741
- Yokoyama, T., Ikawa, S., & Kitano, K. (2019). Plasma disinfection via the reduced-pH method using an ex vivo porcine contaminated skin model. Journal of Physics D: Applied Physics, 52(26), 265401. https://doi.org/10.1088/1361-6463/ab1740
- Zhang, Q., Liang, Y. D., Feng, H. Q., Ma, R. N., Tian, Y., Zhang, J., & Fang, J. (2013). A study of oxidative stress induced by non-thermal plasma-activated water for bacterial damage. Applied Physics Letters, 102(20). https://doi.org/10.1063/1.4807133
- Zhao, D. B., Wang, S. D., Hu, Y. S., Liu, X., Tao, J., Sagratini, G., & Xiang, Q. S. (2022). Insight into the antibacterial activity of lauric arginate against Escherichia coli O157: H7: Membrane disruption and oxidative stress. LWT-Food Science and Technology, 162, 113449. https://doi.org/10.1016/j.lwt.2022.113449