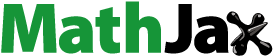
ABSTRACT
This study was conducted to optimize a process of low-temperature plasma treatment for the decontamination of Aspergillus flavus (A. flavus) on corn kernels. Impacts of plasma-treated time (30, 60, 90 s), plasma-treated power (300, 400, 500 W), and vacuum degree (85, 90, 95 Pa) on the decontamination effect in this process were explored. The optimal conditions included vacuum degree at 90 Pa, plasma treating time of 90 s, and plasma treating power of 500 W, under which the A. flavus spores was 4.03 log (cfu/g). Plasma treatment triggered antioxidative defense system, broke cell structures, and dispersed cell contents. A large number of free radicals act on lipids and proteins, and malondialdehyde (MDA) accumulate in large quantities, further leading to damage to cell structures.
1. Introduction
Globally, corn (Zea mays L.) is a major cereal crop and is widely planted (Miano et al., Citation2017). However, corn kernels are a favoured host for A. flavus at harvest or during postharvest operations and storage (B. G. Dasan et al., Citation2017). Aflatoxins produced by A. flavus are the most toxic class of mycotoxins, which are toxigenic, carcinogenic, and mutagenic to humans (Dijksterhuis, Citation2017; Gao et al., Citation2022). Aflatoxins with stable molecular structures are unremovable (Los et al., Citation2020). Thus, elimination of A. flavus before aflatoxin formation is necessary to ensure corn safety and quality (Fountain et al., Citation2014; Zheng et al., Citation2017). Prevention of in-field mycotoxin contamination is the primary aim in agricultural and food sectors, and the common way for this aim is to prevent growing crops from fungal infection (Dasan, Mutlu, et al., Citation2016).
Recently, many approaches have been developed to decontaminate A. flavus growth, such as UV, γ ray processing, ozone, heat treatment, antifungal chemicals, and biological methods (Ma et al., Citation2017; Wu et al., Citation2021). Despite the ability to restrict surface pollution, these approaches may trigger food safety problems, such as changing nutritional or sensory quality (flavor, color, and texture), unsatisfactory efficacy, and cost implications (Dasan, Mutlu, et al., Citation2016; Ma et al., Citation2019). Therefore, the shortcomings above have driven the establishment of novel methods that are highly efficient, nontoxic, and compatible to a wide range of materials (Makari et al., Citation2021; Suhem et al., Citation2013).
Low-temperature plasma, a non-thermal treatment, is a flow of ionized gases that contain various atoms, ions, electrons, neutral molecules, and exited species (Amini & Ghoranneviss, Citation2016; Hojnik et al., Citation2019; Los et al., Citation2018; Xu et al., Citation2021). Low-temperature plasma was first adopted in surface engineering to strengthen the antimicrobial ability (Niemira, Citation2012; Sarangapani et al., Citation2015). Recently, low-temperature plasma in degradation of food contaminants has drawn considerable attention (Gavahian & Khaneghah, Citation2020; Gavahian et al., Citation2020; Wielogorska et al., Citation2019). Different antimicrobial substances such as atomic oxygen, ozone, hydroxyl radical, reactive N, O, or N species, and UV photons will be generated, which account for various bioreactions from intercellular DNA fracturing and protein decomposition to outer membrane oxidation (Von Woedtke et al., Citation2013; Zhao et al., Citation2023). The antimicrobial action of plasma treatment can be explained by three primary mechanisms: (1) chemical bonds of cells are broken by reactive species and charged particles (Fernández & Thompson, Citation2012); (2) reactive oxygen species interact with vital cellular bio-molecules, altering the function of biological membranes via interaction with lipids (Misra & Jo, Citation2017); (3) UV-mediated DNA strand is broken and reduced in cell replication. While one mode of action may be dominant in different plasma systems, the highest antimicrobial effectiveness will be derived from several mechanisms (Doshi & Šerá, Citation2023).
Several eliminate approaches of aflatoxins have been studied such as chemical fungicides, washing (Hussain et al., Citation2011), irradiation (Ribeiro et al., Citation2011), ultraviolet, pulsed light processing, and microwave heating (Basaran & Akhan, Citation2010; Czylkowski et al., Citation2013). Khalil et al. (Citation2021) studied the effect of gamma irradiation doses on the inhibition and reduction of aflatoxins, and the results showed that the gamma-irradiated dose of 4.5 kGy was found to reduce the production of their mycotoxins. After 80 s of pulsed light treatment (0.52 J/cm2/pulse) on rough rice, AFB1 and AFB2 were reduced by 75.0% and 39.2%, respectively. However, a treatment time of 15 s on rice bran reduced AFB1 and AFB2 by 90.3% and 86.7%, respectively (Wang et al., Citation2016). Under the conditions of dry-heat and wet-heat, the degradation effect of microwave heating on aflatoxins B1 is 5 ~ 8% higher than that of traditional heating (Shi, Citation2016).
Compared with traditional methods, low-temperature plasma has many advantages, including short sterilization time, good sterilization effect, and avoidance of chemical reagent residues. In addition, plasma species only attack the surface of matrices, which is contributive, as a shallow penetrating depth helps maintain corn nutrition (Li et al., Citation2019). Recently, research on potential applications of plasma has been developed in food engineering and food processing. Plasma has been potentially applied in food engineering and processing. One recent work reported that Pichia manshurica reduced by 2.38 and 5.11 lg CFU/mL after 4.5 and 7.5 min dielectric barrier discharge cold plasma treatment (80 kV, 50 Hz) (Hou et al., Citation2023). Escherichia coli in water significantly reduced by 1.7 MPN/100 mL of plasma treatment at the discharge power of 200 W and 6 min (Bakhshzadmahmoudi et al., Citation2022). After Brassica napus seeds were exposed to DBD plasma or indirect plasma plasma-treated air for 15 min, Bacillus atrophaeus was reduced 0.5 or 5.2 log (Schnabel et al., Citation2012). The review on the sterilizing effect of non-thermal plasma on material surface concentrated on bacteria (Zhang et al., Citation2023). Compared with bacteria, the impact of non-thermal air plasma on aflatoxigenic fungi in foods was basically ignored (Scholtz et al., Citation2015). Trebulová et al. (Citation2023) found the inhibitory effects of plasma are directly proportional to the plasma-treated time, plasma-treated power, and the overall functioning of the plasma source. Dasan, Boyaci, et al. (Citation2016) reported that atmospheric pressure fluidized bed plasma can inactivate A. flavus spores decontamination of corn kernels, and they found that plasma reduced 5.48 log (cfu/g) of spores after 5 min of air plasma treatment. In addition, after hazelnuts, peanuts, and pistachio nuts inoculated with Aspergillus parasiticus were subjected to air gases plasma, the reduction rates after 5 min were 1 log. The plasma treatment was more effective on Aspergillus parasiticus at the initial stage than the later stage. Moreover, Dasan, Mutlu, et al. (Citation2016) found that 5 min of dry air plasma treatment at 655 W significantly reduced the levels of A. flavus inoculated on hazelnuts by 4.50 after 5 min of treatments at 655 W using dry air as the plasma forming gas. Plasma exposure at power of 40 W for 20 min reduced mold growth by 4 log cfu/g in brown rice cereal bars, and prevented mycelium growth on the surface for at least 20 days (Suhem et al., Citation2013).
Given the low processing and non-destructiveness in the nutrition of plasma, the present study focused on modeling the effects of process indices (e.g. plasma treating time, plasma power, vacuum degree) on the elimination of A. flavus in corn kernels. Response models were built with the testing data and used to optimize the conditions for corn kernel inactivation of aflatoxigenic fungi and to maximize the inactivation efficacy.
2. Materials and methods
2.1. Corn samples
Corn was purchased from a local shop in Jilin, China. Samples in weight of 1.8 ± 0.2 g and density of 0.87 ± 0.03 g/cm3 were kept at 4°C.
2.2. Microbial strains, inoculation, and sample preparation
A. flavus (Y2–3) was obtained from the Microbiology Laboratory, College of Biological and Agricultural Engineering, Jilin University. Corn kernels were treated with 70% ethanol solution for 10 min to remove microorganisms on the surface. Then, the samples were cultured for 5 days at 25–28°C on the plates of Yeast Extract Glucose Chloramphenicol (YGC). After that, no cultivable spore was found on YGC agar. A 10 g sample of corn kernels was put on A. flavus, respectively, cultures planted on YGC agar, agitated for 30 s artificiality, and inoculated homogenously with mold spores to a level of 108 CFU/g. The samples were transferred to a sterile Petri dish and cultured at 25–28°C for 24 h in advance to ensure mold spore attachment.
2.3. Plasma equipment
The SY-DT02S cold plasma system (Ops Technology Co., Ltd., Suzhou, China, ) consists of a chamber, a high-voltage transformer, and a pressure supply control system. Plasma was ignited inside equipped with electrodes in the stainless steel chamber (260 × 260 mm2) (). In the chamber, the pressure measured by an attached vacuum gauge can evacuate maximally to 10 Pa and was modulated via a vacuum pump along with a pressure-control valve. The plasma device can operate under a reference voltage of 220 V. The input power can be altered within 0–500 W by tuning the cold plasma producer, which can be run with two working gases from 40% to 100% standard voltage and 40 kHz frequency at an air flow rate of 50–300 mL/min.
2.4. Experimental procedure of the plasma-treated spore survivors on corn kernels surface
Corn kernels were artificiality polluted with A. flavus cultured for 24 h at 25–28°C for plasma exposure. Then, the samples were treated at 150 mL/min air flow and 40 kHz for certain time. After that, the corn kernels were dispersed into a 0.85% sterile saline solution with 0.1% Tween 80 and cleaned for 15 min under shaking. Mold spores were counted with surface plating (100 μL) on YGC agar. All plates were cultured at 25–28°C for 5–7 days before the counting (Dasan, Boyaci, et al., Citation2016). All tests of the colony count were replicated at least two times. The colony count can be presented as the colonies forming units (CFU) (EquationEquation 1(1)
(1) ).
where Y represents CFU; N0 represents the number of colonies before plasma treatment; N represents the number of colonies after plasma treatment.
2.5. Experimental design and response surface methodology (RSM)
RSM was used to optimize and build the plasma operation to determine the total colony counts of A. flavus on corn kernels. The ranges and levels of the three independent factors in the drying assays, which were determined empirically, are listed in . The yield response as colony counts, including A. flavus spores(Y), were assumed to be affected by three independent variables: X1 for plasma-treated time, X2 for plasma-treated power, and X3 for vacuum degree. The design matrix involved 17 trials, including five central points. Experimental values and coded levels of the three factors are shown in .
Table 1. Levels of independent variables chosen for the experimental design.
Table 2. Box–Behnken design: effects of process variables on decontamination effect parameters.
The quadratic polynomial equation is obtained through RSM analysis, which represents the response (Yn) as a function of factors (EquationEquation 2(2)
(2) ) (Hosseinzadeh Samani et al., Citation2020; Lee & Yoon, Citation2021; Martínez et al., Citation2017).
where Y represents predicted response; βi represents the coefficients of the linear terms; βii represents the quadratic parameter; βij represents the interactive components; Xi and Xj represent the levels of the independent variables; k represents the number of input variables and ε represents the standard random error.
Regression models were set up from the testing data. The coefficient of determination (R2) and the adjusted R2 were analyzed as criteria to evaluate the quality of the model, which reveal the ranges of variance of the tested factors that can be interpreted by the model.
2.6. Conductivity determination
A 10 g sample of corn kernels contaminated with A. flavus was treated with plasma at 90 Pa and 500 W for 0, 30, 60, and 90 s, respectively. Then, the samples were cultured for 4 h at 25–28°C on plates of YGC. Extracellular conductivity was measured every 1 hour. All tests were replicated at least three times (Shen et al., Citation2015).
2.7. Antioxidant enzyme activity assays
Superoxide dismutase (SOD) enzyme activity was measured using a superoxide dismutase detection kit (Nanjing Jiancheng Biology Company, Nanjing, China). Catalase (CAT) was measured using a catalase detection kit (Nanjing Jiancheng Biology Company, Nanjing, China).
2.8. Malondialdehyde (MDA)
MDA content was measured using a superoxide dismutase detection kit (Nanjing Jiancheng Biology Company, Nanjing, China).
2.9. Scanning electron microscope (SEM) observations
The morphology of the control and plasma-treated (at 90 Pa and 500 W for 90 s) A. flavus spores was tested via SEM (EVO-18, Carl Zeiss, Germany) in a vacuum mode and at an accelerating voltage of 10 kV. The fungal spores were fixed overnight in 2.5% glutaraldehyde at 4°C. Next, the spores were cleaned first with a 0.1 M sodium phosphate buffer and then with water two times (10 min each). After that, the samples were dehydrated in a gradient of ethanol concentrations (10%, 30%, 50%, 70%) for 15 min. The dehydration was completed by washing the samples in 100% ethanol three times for 10 min. After air-drying in a desiccator, the samples were sputter-coated with gold palladium (Dasan, Mutlu, et al., Citation2016).
2.10. Statistical analysis
The experimental results were statistically analyzed using ANOVA using Design-Expert program (V10). Duncan multiple range tests were used to determine the differences between mean values at level of 95% confidence.
3. Results
3.1. Initial concentrations of A. flavus
Corn samples were manually polluted with A. flavus and grown at 25–28°C for 5 days to allow the spores to attach to the corn surface. The initial fungal load was 2.84 ± 1.58 × 108 CFU/g.
3.2. Modeling of A. flavus response
The influence of low-temperature plasma treatment on A. flavus counts is shown in . The F of 144.54 implies the model is significant. The model of drying rate has R2 of 0.9946, adjusted R2 of 0.9878, and root mean square error (RMSE) of 2.90. The good R2 and RMSE indicate the testing data accord with the model (Abasi et al., Citation2017; Khoshtaghaza et al., Citation2015). The A. flavus count was impacted by the plasma treating time, plasma power, and vacuum degree. The significant model terms for the A. flavus count are X1, X2, X3, X1X2, X1X3, X2X3, X12 and X32 (p < .001), whereas the effects of the second order of plasma power are insignificant (p > .05). The small deviations for the A. flavus counts from the five central levels of the test design (4, 10, 14, 16, 17 runs in ) indicate the A. flavus count can be reproduced.
Table 3. Results obtained in the assays for validation of the conditions optimized for low-temperature plasma decontamination of corn kernels.
The A. flavus counts of the samples treated at low-temperature, plasma-treated powers of 300, 400, and 500 W range from 4.31 to 6.44, 4.50 to 6.55, and 4.13 to 4.56 log (cfu/g), respectively. Thus, the decontamination condition at a vacuum degree of 90 Pa and a plasma-treated time of of 90 s is preferable when low-temperature, plasma-treated power is 500 W (). According to the Box–Behnken design, the model was obtained (EquationEquation 3(3)
(3) ).
The actual data are the responses detected from a special run, and the predicted data were obtained from the model. The lines in show that the predicted A. flavus count well fit the actual data, implying that the model can well acquire the relationship between the factors and the A. flavus count.
shows the 3D interactive effects of low-temperature, plasma-treated time, plasma-treated power, and vacuum degree on the decontamination process. Basically, higher plasma power leads to more severe inactivation (Dasan, Mutlu, et al., Citation2016).
With increasing plasma power, the total density of active species formed from the plasma rose, thus killing microbes and intensifying the lethal effect. With the increasing plasma-treated power, the total density of the active species generated during the plasma rose, causing the killing of microorganisms, and thus the lethal affect was also intensified. The prolonged plasma time also considerably impacted the decontamination, as treatment by the charged energetic particles on the corn surface was more severe with longer plasma treating times. This result agrees with the findings from Deeyai et al. (Citation2016), who investigated the effect of air pressure plasma jet on the decrease of A. flavus in bread molds. When the low-temperature plasma time and vacuum degree were constant, the A. flavus count was reduced with an increase in low-temperature plasma power, because high plasma power caused severe damage to the cell membranes of A. flavus. Similarly, Dasan, Mutlu, et al. (Citation2016) found that plasma treatment had a fungicidal impact on A. flavus spores.
3.3. Process optimization
The decontamination indices were optimized on Design-Expert V10 to acquire suitable conditions. Based on the model from , the optimal conditions were identified to be a vacuum degree of 90 Pa, plasma-treated time of 90 s, and plasma-treated power of 500 W (). Under the optimal decontamination conditions, the A. flavus count was 4.03 log (cfu/g).
3.4. Conductivity determination
shows that the conductivity of A. flavus spores significantly increased in the first hour (p < .05). Thereafter, the values were almost unchanged after a slight decrease. The conductivity values of A. flavus samples treated with 0, 30, 60, and 90 s of plasma at 1 h were 15.9 ± 0.85, 23.1 ± 0.47, 34.4 ± 0.64, and 43.6 ± 0.88 ms/cm, respectively. The increase might be due to various degrees of damage caused by plasma treatment on the integrities of the A. flavus spores cell membranes. Cell wall permeability was intensified because of the destruction of the cell membrane, which led to a large amount of electrolyte leakage on the spore cells of A. flavus. The effect herein is in accordance with a previous work. The membrane damage of Escherichia coli treated with plasma jet and analyzed that plasma treatment damages the cell wall and breaks the chemical bonds in microbial cells.
3.5. Antioxidant enzyme activity assays
The curves in represented SOD and CAT activity of A. flavus spores changing with the plasma-treated time. As expected, the results show that plasma treatment affects the SOD and CAT activity. The increase in detected SOD enzymes activities was detected after 30, 60, and 90 s of plasma treatment (). The SOD activity of the samples treated at plasma treatment time of 0, 30, 60, and 90 s was 245 ± 14.77, 425 ± 18.53, 560 ± 26.27, and 634 ± 18.03 U/mgprot, respectively. As can be seen in , CAT activity decreased after plasma treatment in comparison to the control group. The CAT activity of A. flavus treated with plasma for 30, 60, and 90 s was 145 ± 06.78, 289 ± 10.67, and 346 ± 7.98 U/mgprot, and 86 ± 7.01 U/mgprot for the the control group, respectively. The higher antioxidant activity of SOD and CAT values might attributed to plasma treatment causing an increase in intracellular ROS levels, resulting in a mismatch between the levels required for consumption and the production capacity of ROS (Ji et al., Citation2022) (). This is in agreement with the results reported by Šimončicová et al. (Citation2018), who reported the effect of cold atmospheric-pressure plasma on hyphae of A. flavus, revealing an increase in SOD and CAT activities and a decrease in glutathione.
3.6. MDA content
The MDA content was 21.7 ± 0.18 nmol/g for the control group, and for samples after plasma treatment for 30, 60, and 90 s, the MDA content was 29.5 ± 0.29, 36.9 ± 0.89, and 40.2 ± 0.99 nmol/g, respectively (). The results indicate that with an increase in plasma duration, the MDA content of A. flavus samples increased. The results indicate that plasma treatment increases the MDA content. The values increased with increasing plasma-treated time, which might be attributed to a large number of free radicals acting on lipids produced by plasma, and oxidative end products such as MDA and protein carbonyl groups accumulate in large quantities, leading to the damage of the cell membrane components.
3.7. Topographical observations (SEM) images
The SEM images of A. flavus spores before and after plasma process are shown in . As can be seen, the higher the power of plasma treatment, the more severe the destruction of spores. Evidently, the integrity of the cellular structures of A. flavus was completely damaged by the active species formed during the plasma process, whereas the untreated spores were round-shaped as individual spheres. As clearly shown in , exposure to plasma treatment caused severe physical damage with the shapes of the A. flavus spores while the untreated spores were individually spread. After plasma treatment, cell contents were dispersed into the environment following cytoplasmic leakage. The integrity of the spore structure was collapsed. The actions of plasma chemical species caused the disappearance of cell contents and loss of functionality.
Figure 9. SEM images of the surface of control and low-temperature, plasma-treated A. flavus spores. (a) Control , (b) 300 W-30 s, (c) 400 W-30 s, and (d) 500 W-30 s. 1, change in the shape of cells; 2, disruption of spore’s coat; and 3, spore debris due to the bursting of spores.
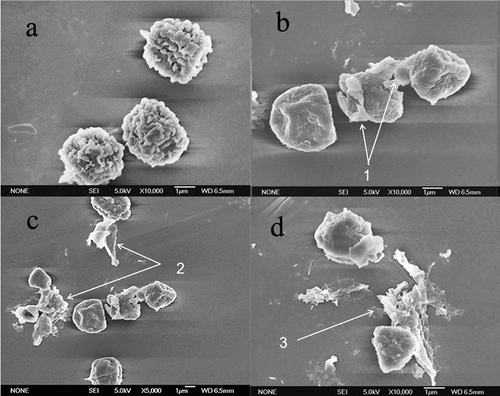
Plasma treatment caused certain damage to fungal spore surface at the micron level. Cell wall permeability was intensified, and cell walls were destroyed due to the erosion mechanism, which led to considerable perforation and disintegration on the spore cells of A. flavus. The antimicrobial effects of low-temperature plasma treatment on microorganisms included (1) lipid peroxidation resulting from superoxide anion and hydrogen peroxide; (2) protein oxidation through the susceptibility of amino acids to oxidation and loss of enzyme activity; (3) nucleic acids damage resulting from DNA and RNA reduction in cell replication (Mai-Prochnow et al., Citation2014). SEM results show the morphological changes in morphology of spores or their lysis in the morphological structures caused by the plasma process.
4. Conclusions
Low-temperature plasma treatment provides the advantage of high microbial inactivation. Response surface methodology was successfully used to optimize the effects of plasma treatment conditions for inhibiting A. flavus on corn kernels. The suitable decontamination effect on A. flavus on the surface of corn kernels was determined by optimizing the effects of these factors on Design-Expert. Response surface regression showed that plasma treating time, plasma power, and vacuum degree were significantly related to A. flavus counts. The testing and predicted data were not significantly different. The optimal decontamination conditions were vacuum degree of 90 Pa, plasma-treated power of 500 W, and plasma-treated time of 90 s, and the corresponding A. flavus was 4.03 log (cfu/g). These results indicate that low-temperature plasma treatment can inactivate A. flavus on corn kernels. Plasma treatment triggered antioxidative defense system, increasing the content of SOD and CAT. SEM showed that low-temperature plasma treatment broke cell structures and disperse cell contents. A large number of free radicals act on lipids and proteins, and malondialdehyde accumulates in large quantities, further leading to damage to cell structures.
Author contributions
Shuai Li and Jingxin Song designed the study. Shuai Li collected test data and drafted the manuscript. Haiyang Zou and Mo Niu performed the data analyses. Yingnan Zeng approved the final version.
Disclosure statement
We declare that we have no financial and personal relationships with other people or organizations that can inappropriately influence our work, there is no professional or other personal interest of any nature or kind in any product, service and/or company that could be construed as influencing the position presented in, or the review of, the manuscript entitled.
Additional information
Funding
References
- Abasi, S., Minaei, S., & Khoshtaghaza, M. H. (2017). Effect of desiccant system on thin layer drying kinetics of corn. Journal of Food Science and Technology, 54(13), 4397–10. https://doi.org/10.1007/s13197-017-2914-z
- Amini, M., & Ghoranneviss, M. (2016). Effects of cold plasma treatment on antioxidants activity, phenolic contents and shelf life of fresh and dried walnut (Juglans regia L.) cultivars during storage. LWT-Food Science and Technology, 73, 178–184. https://doi.org/10.1016/j.lwt.2016.06.014
- Bakhshzadmahmoudi, M., Morshedian, N., Mehramiz, A., & Kharaghani, M. (2022). Inactivation of Escherichia coli by atmospheric pressure plasma jet in water. Journal of Water and Health, 20(6), 962–971. https://doi.org/10.2166/wh.2022.011
- Basaran, P., & Akhan, Ü. (2010). Microwave irradiation of hazelnuts for the control of aflatoxin producing Aspergillus parasiticus. Innovative Food Science & Emerging Technologies, 11(1), 113–117. https://doi.org/10.1016/j.ifset.2009.08.010
- Czylkowski, D., Hrycak, B., Jasiński, M., Dors, M., & Mizeraczyk, J. (2013). Atmospheric pressure microwave microplasma microorganism deactivation. Surface & Coatings Technology, 234, 114–119. https://doi.org/10.1016/j.surfcoat.2013.04.010
- Dasan, B. G., Boyaci, I. H., & Mutlu, M. (2016). Inactivation of aflatoxigenic fungi (Aspergillus spp.) on granular food model, maize, in an atmospheric pressure fluidized bed plasma system. Food Control, 70, 1–8. https://doi.org/10.1016/j.foodcont.2016.05.015
- Dasan, B. G., Boyaci, I. H., & Mutlu, M. (2017). Nonthermal plasma treatment of Aspergillus spp. spores on hazelnuts in an atmospheric pressure fluidized bed plasma system: Impact of process parameters and surveillance of the residual viability of spores. Journal of Food Engineering, 196, 139–149. https://doi.org/10.1016/j.jfoodeng.2016.09.028
- Dasan, B. G., Mutlu, M., & Boyaci, I. H. (2016). Decontamination of Aspergillus flavus and Aspergillus parasiticus spores on hazelnuts via atmospheric pressure fluidized bed plasma reactor. International Journal of Food Microbiology, 216, 50–59. https://doi.org/10.1016/j.ijfoodmicro.2015.09.006
- Deeyai, P., Amnuaycheewa, P., & Kerdtongmee, P. (2016). Effect of atmospheric pressure argon plasma jet on the growth of bread molds. Key Engineering Materials, 675, 744–748. https://doi.org/10.4028/www.scientific.net/KEM.675-676.744
- Dijksterhuis, J. (2017). The fungal spore and food spoilage. Current Opinion in Food Science, 17, 68–74. https://doi.org/10.1016/j.cofs.2017.10.006
- Doshi, P., & Šerá, B. (2023). Role of non-thermal plasma in fusarium inactivation and mycotoxin decontamination. Plants, 12(3), 627. https://doi.org/10.3390/plants12030627
- Fernández, A., & Thompson, A. (2012). The inactivation of Salmonella by cold atmospheric plasma treatment. Food Research International, 45(2), 678–684. https://doi.org/10.1016/j.foodres.2011.04.009
- Fountain, J. C., Scully, B. T., Ni, X., Kemerait, R. C., Lee, R. D., Chen, Z. Y, and Guo, B. (2014). Environmental influences on maize-Aspergillus flavus interactions and aflatoxin production. Frontiers in Microbiology, 5, 76491. https://doi.org/10.3389/fmicb.2014.00040
- Gao, F., Li, Y., Zhao, H., Liang, Y., & Liu, Z. (2022). Sub-chronic, low dose co-exposure to aflatoxin B1 and microcystin-LR in C57BL/6 mice significantly alters the cytokine response in serum and liver. CyTA-Journal of Food, 20(1), 271–284. https://doi.org/10.1080/19476337.2022.2130436
- Gavahian, M., & Khaneghah, A. M. (2020). Cold plasma as a tool for the elimination of food contaminants: Recent advances and future trends. Critical Reviews in Food Science and Nutrition, 60(9), 1581–1592. https://doi.org/10.1080/10408398.2019.1584600
- Gavahian, M., Pallares, N., Al Khawli, F., Ferrer, E., & Barba, F. J. (2020). Recent advances in the application of innovative food processing technologies for mycotoxins and pesticide reduction in foods. Trends in Food Science & Technology, 106, 209–218. https://doi.org/10.1016/j.tifs.2020.09.018
- Hojnik, N., Modic, M., Ni, Y., Filipič, G., Cvelbar, U., & Walsh, J. L. (2019). Effective fungal spore inactivation with an environmentally friendly approach based on atmospheric pressure air plasma. Environmental Science & Technology, 53(4), 1893–1904. https://doi.org/10.1021/acs.est.8b05386
- Hosseinzadeh Samani, B., Behruzian, A., Khoshtaghaza, M. H., Behruzian, M., & Ansari Ardali, A. (2020). The investigation and optimization of two combined pasteurization methods of ultrasonic‐pulse electric field and hydrodynamic‐pulse electric field on sour cherry juice using RSM‐TOPSIS. Journal of Food Processing and Preservation, 44(9), e14700. https://doi.org/10.1111/jfpp.14700
- Hou, X., Wang, J., Mei, Y., Ge, L., Qian, J., Huang, Y., & Zhao, N. (2023). Antibiofilm mechanism of dielectric barrier discharge cold plasma against Pichia manshurica. Innovative Food Science & Emerging Technologies, 85, 103340. https://doi.org/10.1016/j.ifset.2023.103340
- Hussain, A., Lutfullah, G., & Khan, S. (2011). Reduction of aflatoxin B1 contamination in Pakistani wheat varieties by physical methods. Pakistan Journal of Scientific and Industrial Research, 54(1), 23–28. https://doi.org/10.52763/PJSIR.BIOL.SCI.54.1.2011.23.28
- Ji, M., Li, J., & Fan, L. (2022). Study on the antifungal effect and mechanism of oregano essential oil fumigation against Aspergillus flavus. Journal of Food Processing and Preservation, 46(11), e17026. https://doi.org/10.1111/jfpp.17026
- Khalil, O. A. A., Hammad, A. A., & Sebaei, A. S. (2021). Aspergillus flavus and Aspergillus ochraceus inhibition and reduction of aflatoxins and ochratoxin a in maize by irradiation. Toxicon, 198(1), 111–120. https://doi.org/10.1016/j.toxicon.2021.04.029
- Khoshtaghaza, M. H., Khojastehnazhand, M., Mojaradi, B., Goodarzi, M., & Saeys, W. (2015). Texture quality analysis of rainbow trout using hyperspectral imaging method. International Journal of Food Properties, 19(5), 974–983. https://doi.org/10.1080/10942912.2015.1042111
- Lee, J. J., & Yoon, K. Y. (2021). Optimization of ultrasound-assisted extraction of phenolic compounds from bitter melon (Momordica charantia) using response surface methodology. CyTA-Journal of Food, 19(1), 721–728. https://doi.org/10.1080/19476337.2021.1973110
- Li, S., Chen, S., Liang, Q., Ma, Z., Han, F., Xu, Y., Jin, W., & Wu, W. (2019). Low temperature plasma pretreatment enhances hot‐air drying kinetics of corn kernels. Journal of Food Process Engineering, 42(6), e13195. https://doi.org/10.1111/jfpe.13195
- Los, A., Ziuzina, D., Boehm, D., Cullen, P. J., Bourke, P., & Dudley, E. G. (2020). Inactivation efficacies and mechanisms of gas plasma and plasma-activated water against Aspergillus flavus spores and biofilms: A comparative study. Applied and Environmental Microbiology, 86(9), e02619–19. https://doi.org/10.1128/AEM.02619-19
- Los, A., Ziuzina, D., & Bourke, P. (2018). Current and future technologies for microbiological decontamination of cereal grains. Journal of Food Science, 83(6), 1484–1493. https://doi.org/10.1111/1750-3841.14181
- Mai-Prochnow, A., Murphy, A. B., McLean, K. M., Kong, M. G., & Ostrikov, K. K. (2014). Atmospheric pressure plasmas: Infection control and bacterial responses. International Journal of Antimicrobial Agents, 43(6), 508–517. https://doi.org/10.1016/j.ijantimicag.2014.01.025
- Makari, M., Hojjati, M., Shahbazi, S., Askari, H., & Wan, C. (2021). Elimination of Aspergillus flavus from pistachio nuts with dielectric barrier discharge (DBD) cold plasma and its impacts on biochemical indices. Journal of Food Quality, 2021, 1–12. https://doi.org/10.1155/2021/9968711
- Martínez, M. L., Bordón, M. G., Lallana, R. L., Ribotta, P. D., & Maestri, D. M. (2017). Optimization of sesame oil extraction by screw-pressing at low temperature. Food and Bioprocess Technology, 10(6), 1113–1121. https://doi.org/10.1007/s11947-017-1885-4
- Ma, W., Zhao, L., & Xie, Y. (2017). Inhibitory effect of (E)-2-hexenal as a potential natural fumigant on Aspergillus flavus in stored peanut seeds. Industrial Crops and Products, 107, 206–210. https://doi.org/10.1016/j.indcrop.2017.05.051
- Ma, W., Zhao, L., Zhao, W., & Xie, Y. (2019). (E)-2-hexenal, as a potential natural antifungal compound, inhibits Aspergillus flavus spore germination by disrupting mitochondrial energy metabolism. Journal of Agricultural and Food Chemistry, 67(4), 1138–1145. https://doi.org/10.1021/acs.jafc.8b06367
- Miano, A. C., Ibarz, A., & Augusto, P. E. D. (2017). Ultrasound technology enhances the hydration of corn kernels without affecting their starch properties. Journal of Food Engineering, 197, 34–43. https://doi.org/10.1016/j.jfoodeng.2016.10.024
- Misra, N., & Jo, C. (2017). Applications of cold plasma technology for microbiological safety in meat industry. Trends in Food Science & Technology, 64, 74–86. https://doi.org/10.1016/j.tifs.2017.04.005
- Niemira, B. A. (2012). Cold plasma decontamination of foods. Annual Review of Biomedical Engineering, 3(1), 125–142. https://doi.org/10.1146/annurev-food-022811-101132
- Ribeiro, J., Cavaglieri, L., Vital, H., Cristofolini, A., Merkis, C., Astoreca, A., & Rosa, C. A. R. (2011). Effect of gamma radiation on Aspergillus flavus and Aspergillus ochraceus ultrastructure and mycotoxin production. Radiation Physics & Chemistry, 80(5), 658–663. https://doi.org/10.1016/j.radphyschem.2010.12.017
- Sarangapani, C., Devi, Y., Thirundas, R., Annapure, U. S., & Deshmukh, R. R. (2015). Effect of low-pressure plasma on physico-chemical properties of parboiled rice. LWT-Food Science and Technology, 63(1), 452–460. https://doi.org/10.1016/j.lwt.2015.03.026
- Schnabel, U., Niquet, R., Krohmann, U., Winter, J., Schlüter, O., Weltmann, K. D., & Ehlbeck, J. (2012). Decontamination of microbiologically contaminated specimen by direct and indirect plasma treatment. Plasma Processes and Polymers, 9(6), 569–575. https://doi.org/10.1002/ppap.201100088
- Scholtz, V., Pazlarova, J., Souskova, H., Khun, J., & Julak, J. (2015). Nonthermal plasma—A tool for decontamination and disinfection. Biotechnology Advances, 33(6), 1108–1119. https://doi.org/10.1016/j.biotechadv.2015.01.002
- Shen, S., Zhang, T., Yuan, Y., Lin, S., Xu, J., & Ye, H. (2015). Effects of cinnamaldehyde on Escherichia coli and staphylococcus aureus membrane. Food Control, 47, 196–202. https://doi.org/10.1016/j.foodcont.2014.07.003
- Shi, H. (2016). Investigation of methods for reducing aflatoxin contamination in distillers grains [ Doctoral dissertation]. Purdue University
- Šimončicová, J., Kaliňáková, B., Kováčik, D., Medvecká, V., Lakatoš, B., Kryštofová, S., & Zahoranová, A. (2018). Cold plasma treatment triggers antioxidative defense system and induces changes in hyphal surface and subcellular structures of Aspergillus flavus. Applied Microbiology and Biotechnology, 102(15), 6647–6658. https://doi.org/10.1007/s00253-018-9118-y
- Suhem, K., Matan, N., Nisoa, M., & Matan, N. (2013). Inhibition of Aspergillus flavus on agar media and brown rice cereal bars using cold atmospheric plasma treatment. International Journal of Food Microbiology, 161(2), 107–111. https://doi.org/10.1016/j.ijfoodmicro.2012.12.002
- Trebulová, K., Krčma, F., Skoumalová, P., Kozáková, Z., & Machala, Z. (2023). Effects of different cold atmospheric‐pressure plasma sources on the yeast Candida glabrata. Plasma Processes and Polymers, 20(12), e2300048. https://doi.org/10.1002/ppap.202300048
- Von Woedtke, T., Reuter, S., Masur, K., & Weltmann, K. D. (2013). Plasmas for medicine. Physics Reports, 530(4), 291–320. https://doi.org/10.1016/j.physrep.2013.05.005
- Wang, B., Khir, R., Pan, Z. L., Wu, B., Ma, H., Zhao, L., & Mahoney, N. E. (2016). Effectiveness of pulsed light treatment for degradation and detoxification of aflatoxin B-1 and B-2 in rough rice and rice bran. Food Control, 59, 461–467. https://doi.org/10.1016/j.foodcont.2015.06.030
- Wielogorska, E., Ahmed, Y., Meneely, J., Graham, W. G., Elliott, C. T., & Gilmore, B. F. (2019). A holistic study to understand the detoxification of mycotoxins in maize and impact on its molecular integrity using cold atmospheric plasma treatment. Food Chemistry, 301, 125281. https://doi.org/10.1016/j.foodchem.2019.125281
- Wu, Y., Cheng, J. H., & Sun, D. W. (2021). Blocking and degradation of aflatoxins by cold plasma treatments: Applications and mechanisms. Trends in Food Science & Technology, 109, 647–661. https://doi.org/10.1016/j.tifs.2021.01.053
- Xu, H., Zhu, Y., Du, M., Wang, Y., Ju, S., Ma, R., & Jiao, Z. (2021). Subcellular mechanism of microbial inactivation during water disinfection by cold atmospheric-pressure plasma. Water Research, 188, 116513. https://doi.org/10.1016/j.watres.2020.116513
- Zhang, H., Zhang, C., & Han, Q. (2023). Mechanisms of bacterial inhibition and tolerance around cold atmospheric plasma. Applied Microbiology and Biotechnology, 107(17), 5301–5316. https://doi.org/10.1007/s00253-023-12618-w
- Zhao, L., Wang, J., Sheng, X., Li, S., Yan, W., Qian, J., & Raghavan, V. (2023). Non-thermal plasma inhibited the growth and aflatoxins production of Aspergillus flavus, degraded aflatoxin B1 and its potential mechanisms. The Chemical Engineering Journal, 475, 146017. https://doi.org/10.1016/j.cej.2023.146017
- Zheng, A., Zhang, L., & Wang, S. (2017). Verification of radio frequency pasteurization treatment for controlling Aspergillus parasiticus on corn grains. International Journal of Food Microbiology, 249, 27–34. https://doi.org/10.1016/j.ijfoodmicro.2017.02.017