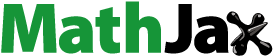
ABSTRACT
This work aimed to study the kinetics and mechanisms of Botrytis cinerea conidia inactivation by aqueous ozone treatments (2.5 ± 0.8 mg O3 L−1 and 4.2 ± 0.2 mg O3 L−1, 0.5–16 min), using conventional plate count technique, flow cytometry analysis (FCM), and transmission electron microscopy (TEM). The effect of treatments on the mold incidence on two artificially contaminated strawberries cultivars over refrigerated storage (6 ± 1 °C) was also investigated. The inactivation pattern of B. cinerea conidial suspensions depended on initial level of the microorganism, dissolved ozone concentration and treatment time. Non-linear survival curves were obtained and fitted successfully with the Weibullian model. FCM and TEM analysis revealed alterations in the physiological state and ultrastructure of ozonated conidia that depended on the ozone dose. Ozonation (4.4 mg O3L−1, 5–10 min) reduced B. cinerea incidence throughout storage in strawberries of cv. Fortuna, but no effect was found in cv. Aroma.
1. Introduction
Botrytis cinerea is a generalist necrotrophic pathogen responsible of gray mold disease in a wide range of plant species (Bi et al., Citation2023). It is among the most important plant pathogen due to its impacts on pre- and post-harvest of many crops in different regions, causing important economic losses in agriculture (Dean et al., Citation2012; Orozco-Mosqueda et al., Citation2023; Petrasch et al., Citation2019). B. cinerea infection can occur by different pathways which can differ depending on the plant species, tissue type, and external conditions (Bi et al., Citation2023). It is assumed that infection involves different stages such as adhesion and germination of B. cinerea conidia on the host surface, cell death of plant tissue in a local region, and finally, accumulation of fungal biomass and spread of the disease (Bi et al., Citation2023; Veloso & van Kan, Citation2018). The infection strategies in the different stages include the secretion of cell wall-degrading enzymes and non-specific phytotoxic metabolites, oxygen reactive species (ROS), and molecules which induce the plant hypersensitive response (Veloso & van Kan, Citation2018).
Grey mold is one of the main diseases affecting strawberry crops worldwide. Strawberry fruits are most resistant to infection in their immature stage, where the fungus can remain in a quiescent state. But susceptibility to infection increases as ripening progresses (Petrasch et al., Citation2019). Differences in the incidence and severity of B. cinerea infection among strawberry cultivars have been described (Bestfleisch et al., Citation2015; Li et al., Citation2022). Current disease management relies mainly in the application of synthetics fungicides. Nonetheless, this control strategy is being questioned in strawberry and various horticultural crop production due to the increasing emergence of fungicide-resistant Botrytis strains, as well as the risks they pose to human health and the environment (Bardas et al., Citation2010; Cosseboom et al., Citation2019; Petrasch et al., Citation2019). Therefore, new effective and safe fungal control strategies are needed to be evaluated.
Ozone has been extensively investigated to control postharvest diseases in fruit and vegetables due to its strong oxidative properties (Aslam et al., Citation2020; Pandiselvam et al., Citation2019). It has gained great interest in the food industry because its decomposition does not leave residues and presents a flexibility of application methods (gas or aqueous phase). Microorganisms’ inactivation by ozone is a complex process that involves damage to different cellular structures and cell content constituents (Xue et al., Citation2023).
The antimicrobial efficacy depends on intrinsic factors (e.g. strain of microorganism, age and size of the microorganism population, food characteristics) and extrinsic factors (e.g. temperature and pH of the medium, ozone concentration, contact time, amount of organic matter; Epelle et al., Citation2023). Several authors have demonstrated the antimicrobial activity of ozone on Botrytis cinerea (Minas et al., Citation2010; Pagès et al., Citation2015; Zhou et al., Citation2019), but further research is needed to determine the influence on inactivation of different intrinsic and extrinsic factors, as well as to better elucidate the mechanisms of inactivation. Moreover, their effectiveness to inhibit or retard the growth of Botrytis cinerea on strawberries has been evaluated mainly in treatment performed in gas phase (Nadas et al., Citation2003; Tuffi et al., Citation2012; Tzortzakis, Citation2023), but little research has been conducted in the aqueous phase (Contigiani et al., Citation2020).
This research was aimed to: a) analyze the influence of the microorganism load and ozone concentration on the inactivation of Botrytis cinerea conidia in suspension, b) determine the effect of treatments on the physiological state, morphology and ultrastructure of conidia by flow cytometry and transmission electron microscopy studies, and c) evaluate the impact of aqueous ozone treatments on B. cinerea development in two artificially contaminated strawberries cultivars stored under refrigeration.
2. Materials and methods
2.1. Chemicals and culture medium
Malt extract agar (MEA) was purchased from Britania (Argentina). Oxygen (99.5% of purity) was obtained from Oxígeno Central (Argentina). Sodium thiosulfate (9% v/v) was obtained from Biopack (Argentina). Fluorescein diacetate solution (FDA) was from Calbiochem (Germany). Propidium iodide (PI), uranyl acetate and lead citrate were purchased from Sigma Aldrich (USA). Epon 812 resin was purchase from Shell Chemical Company (USA).
2.2. Microorganism culture and preparation of conidial suspensions
Botrytis cinerea strain BAFC 3003, provided by the Faculty of Exact and Natural Sciences of the Buenos Aires University, was used in the study. Fungal conidia were cultivated on malt extract agar (MEA) and incubated for 14 days at 25 ± 1°C. Afterward, the spores were collected by scraping them from the agar with a sterile spatula and suspended in sterile distilled water. The suspension was subsequently filtered through sterile gauzes to eliminate large mycelium fragments and diluted to obtain final concentrations of approximately 103 and 105 conidia mL−1. These suspensions were immediately used to perform the experiments.
2.3. Botrytis cinerea inoculation in strawberries
Strawberries (Fragaria x ananassa Duch cv. Fortuna (°Brix = 6.2 ± 0.7; pH = 3.9 ± 0.3; acidity = 0.63 ± 0.03% w/w citric acid) and Aroma (°Brix = 5.7 ± 0.1; pH = 3.5 ± 0.2; acidity = 0.96 ± 0.02% w/w citric acid) maturity index 100% red color were obtained from a local orchard and immediately transferred to the laboratory. Fruits were classified by size and those that presented physiological abnormalities, mechanical damage, or microbiological deterioration were discarded. Subsequently, strawberries were immersed into a hypochlorite solution (200 mg L−1) for 2 min, rinsed with sterile distilled water, and dried with an absorbent paper. The inoculation of B. cinerea in the decontaminated fruit was performed by a spot-inoculation method (Romero-Bernal et al., Citation2019). Two superficial injuries on each fruit skin were made, approximately midway between the calyx and the equatorial zone. In each injury, 10 µL of the conidia suspension (≈105 conidia mL−1) were added. For each cultivar, a total of 120 inoculated strawberries were randomly placed in in 26 × 19× 6 cm trays (10 fruit per tray) and kept inside a biosafety cabinet for 2 h to promote the fixation of the conidia in the fruit. The trays were subsequently kept closed at 20 ± 1°C overnight prior to the treatments.
2.4. Ozone treatments
Ozone treatments were performed in a bubble column with an inbuilt diffuser, using a corona discharge ozone generator (UTK-O-4, Unitek S.A., Argentina) supplied with oxygen (99.5% of purity; 0.62 bar), as described by Garcia-Loredo et al. (Citation2015). Ozonation of conidia suspensions (≈103 and 105 conidia/mL; 0.5 L) were performed applying two ozone levels at the inlet gas flow (9.2 ± 0.4 or 16.5 ± 0.8 mg O3 L−1; flow rate = 5 L min−1; temperature = 20 ± 1 °C) and different exposure times (1–16 min). Experiments were performed in duplicate. Treated and untreated suspensions (control) were stored in sterile glass bottles containing a neutralizing solution of 0.005 M sodium thiosulfate (9% v/v) until analysis.
Strawberries inoculated with B. cinerea were ozonized using 1.25 L of sterile distilled water and 16.5 mg O3 L−1 in the column inlet gas supply. Five strawberries (~150 g) were immersed into the distilled water and exposed to ozone for 5 and 10 min at 20 ± 1°C. After treatments, the fruit were gently dried with absorbent paper, packed in groups of 5 strawberries in air-permeable polypropylene trays (26 cm × 19 cm × 6 cm), and stored at 6 ± 1°C for 10 days. Forty strawberries were analyzed for each treatment condition and cultivar. Ozonized strawberries were compared against untreated inoculated fruit (control).
For both in vitro and in vivo assays, the concentration of dissolved ozone as a function of treatment time in the bubble column was evaluated by the indigo spectrophotometric method, as was previously described by Contigiani et al. (Citation2018). A stabilization period of 3–5 min was required to reach a constant ozone concentration inside the column. The following maximum dissolved ozone concentrations were obtained for the different column inlet ozone concentration (IOC) and volume of liquid used: 2.5 ± 0.8 mg L−1 (0.5 L cell suspension, IOC: 9.2 mg O3 L−1), 4.2 ± 0.2 mg L−1 (0.5 L cell suspension, IOC: 16.5 mg O3 L−1), and 4.9 ± 0.2 mg L−1 (1.25 L distilled water, IOC: 16.5 mg O3 L−1). In treatments carried out with fruits (1.25 L distilled water, 5 strawberries, IOC: 16.5 mg O3 L−1), the dissolved ozone concentration was reduced to 4.4 ± 0.5 mg O3 L−1 due to the interaction of ozone with the organic matter contained in the samples. These maximum concentrations were used to identify the respective treatments when describing the results.
2.5. Survival curves and modelling of B. cinerea conidia inactivation by ozone
Serial dilutions of treated and untreated conidial suspensions were made with sterile peptone water 0.1% (w/v). Subsequently, 0.1 mL of each dilution was surface plated onto MEA contained in Petri dishes. Conidial suspensions without dilution (1 mL) were also pour-plated into MEA. Plates were incubated for 5 days at 25 ± 1°C. Counts were done in duplicate and results were expressed as CFU mL−1. Survival curves were obtained by plotting Log N/N0 versus ozone dose (exposure time x dissolved ozone concentration obtained at each time), where N is the number of CFU mL−1 at a given ozone dose and N0 the initial number of CFU mL−1.
Survival curves were modelled with the cumulative form of a Weibull type distribution of resistances (Peleg & Cole, Citation1998):
where S(d) is the fraction of survivors at a given ozone dose and b and n are the scale and the shape parameters, respectively. The parameter b is linked to the inactivation rate of microbial cells, whereas n is associated with the concavity of the survival curve (n > 1 indicates a downward concavity and n < 1, an upward concavity; a log linear shape is a special case when n = 1). The values of b and n were then used to generate the resistance frequency curves using the following equation:
where dc is the inactivation dose (a measure of the organism’s resistance or sensitivity to the treatment) and is the Weibull distribution corresponding to dc. Other statistical parameters (distribution mode, dcm; mean,
; variance, σdc2; and coefficient of “‘skewness’”, υ1) were calculated from the equations reported by Peleg and Cole (Citation1998) and Peleg (Citation1999). The distribution mode, dcm, represents the ozone dose at which the majority of population dies or is inactivated. The mean,
, corresponds to the inactivation dose on average with its variance, σdc2. The “‘skewness’” coefficient, υ1, represents the skew of the distribution.
2.6. Flow cytometry analysis
Samples processing for flow cytometry analysis (FCM) were carried out following the methodology described by Romero-Bernal et al. (Citation2019). Briefly, conidia suspension (≈105 conidia/mL) exposed or not to ozone were concentrated by two-step centrifugation (1 mL, 7880 g, 5 min) (Eppendorf, model 5804 R, Hamburg, Germany), washed with 1 mL of PBS (pH 7), and resuspended in 100 µL of PBS. Cells were then stained with fluorescein diacetate solution (FDA) (5 mg/mL of acetone, 2 µL) for 30 min at 37°C in the dark. Following incubation with FDA, cell were washed three times with PBS, resuspended in 500 µL of PBS, and stained with propidium iodide (PI) (1 mg/mL of distilled water, 2 µL) for 15 min at 25 ± 1°C and protected from light. Non-treated conidia heated at 100°C for 60 min and stained with PI were used as PI- positive control, whereas non-treated conidia stained only with FDA were employed as FDA positive control. A non-stained-non-treated control was used for determining the autofluorescence of conidia.
A flow cytometer BD FACSAria II (Becton Dickinson, USA), equipped with an argon laser at 488 nm, was used for analysis. Cells were passed through the equipment at a rate of 200 per second, registering a total of 20,000 events per sample. Green fluorescence (F) was detected by the 530/30-FITC filter and red fluorescence (PI) by the 585/42-IP and 695/40-PerCP filters. Measurements were performed in duplicate. Data analyses were carried out using the FlowJo 7.6 (Ashland, USA). Results were represented in two-dimensional dot plot of red fluorescence (PI) versus green fluorescence (F) and side scatter light (SSC) values (associated with complexity) versus forward scatter light (FSC) values (associated with particle size).
2.7. Microscopic observations
Suspensions of untreated and ozone-treated conidia (≈105 conidia mL−1; 100 mL) were centrifuged 45 min at 10,000 rpm. The pellets were resuspended in 1 mL of sterile distilled water and centrifuged (10,000 rpm, 5 min) again. Subsequently, concentrated samples were fixed with glutaraldehyde, dehydrated in a graded ethanol series, embedded in Epon 812 resin (Shell Chemical Company, USA), cut in ultrathin sections, and further stained with uranyl acetate and lead citrate, according to the procedure described by Romero-Bernal et al. (Citation2019). Observations were made with a transmission electron microscope (JOEL, JEM-1200 EX II, Japan) at 80 kV.
2.8. Development of Botrytis cinerea in inoculated strawberries
The growth of Botrytis cinerea on inoculated strawberries was recorded daily by visual inspection over 10 days of storage, taking into account the presence or absence of fungal mycelium development beyond the severity of the infection. Results were reported as percentage of infected fruits.
2.9. Statistical analysis
Results were expressed as mean ± standard deviation. Statistical analyses were performed using the software Infostat v2016 (Córdoba, Argentina). Survival curves fitting was made using Origin Pro 9.1 (Origin Lab Corporation, Northampton USA). Model fit and performance were evaluated by means of the adjusted determination coefficient (R2adj) and root-mean-square error (RMSE). Results of B. cinerea incidence in inoculated strawberries were analyzed by a two-way ANOVA (factors: treatment and storage day) and comparison of means was performed by Duncan´s test. A confidence level of 95% was used.
3. Results and discussion
3.1. Inactivation kinetics of B. cinerea by ozone exposure
Survival curves of B. cinerea conidia suspensions (≈103 and 105 conidia mL−1) ozonized with 2.5 ± 0.8 mg O3 L−1 − 0.5–16 min and 4.2 ± 0.2 mg O3 L−1 − 0.5–12 min are shown in , respectively. The assayed doses ranged between 0.4 and 42.8 mg O3 min L−1 for the ozonization with 2.5 ± 0.8 mg O3 L−1, and between 0.7 and 49.6 for the treatments performed with 4.2 ± 0.2 mg O3 L−1. The inactivation patterns depended on both the initial level of conidia and the ozone dose used in the treatments. Higher inactivation rates were achieved when higher ozone concentration and lower cellular concentration were used. For the 103 conidia mL−1 suspension ozonized with the lowest ozone concentration, no detectable growth was observed after 3 min of ozonization (6.8 mg O3 min L−1) (), whereas for the 105 conidia mL−1 suspension, undetectable growth was found after 10 min (22.3 mg O3 min L−1) (). For the highest ozone concentration, the inactivation times to reduce the cellular population to non-detectable levels were diminished to 1 min (2.7 mg O3 min L−1) and 6 min (23.9 mg O3 min L−1) for the suspensions of 103 and 105 conidia mL−1, respectively (). Zhou et al. (Citation2019) and Pagès et al. (Citation2015) found that even lower ozone concentration than those evaluated in this work (0.3 mg L−1 − 5 min and 1 mg L−1 − 2 min, respectively) were effective in significantly reducing the germination of 105 or 107 conidia mL−1 B. cinerea conidia suspensions by more than 70%.
Figure 1. Experimental survival curve (points) and fitted values derived from Weibull type model (solid line) of Botrytis cinerea conidial suspensions treated with ozone in aqueous phase. a) 103 conidia.mL−1; b) 105 conidia.mL−1. (

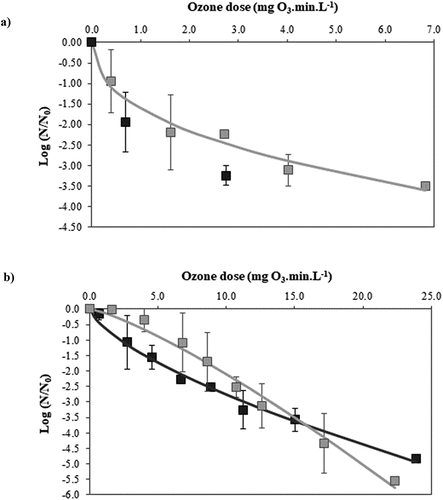
In are also plotted the inactivation curves predicted by the Weibull-type distribution model, except for the survival curve of 103 conidia mL−1 suspension exposed to 4.2 ± 0.2 mg O3 min L−1, which could not be modeled because of the limited experimental data. The shape (n) and scale (b) parameters obtained from the adjustment with the model and the statistical parameters considered to assess the fit goodness are presented in .
Table 1. Weibull-type distribution parameters and statistical descriptors obtained from modeling inactivation curves of Botrytis cinerea conidia in suspension treated with ozone in aqueous phase.
The inactivation curves were successfully fitted with the model, as evidenced by the high values of R2adj and low RMSE values attained. The b parameter raised as the overall decline of the survival curves increased, showing the lowest value the inactivation curve of 103 conidia mL−1 suspension treated with 2.5 mg O3 L−1. In addition, n values < or > 1 were obtained, in accordance with the upward and downward concavity of the curves, respectively. The survival curves of 103 conidia mL−1 suspension treated with 2.5 mg O3 L−1 and 105 conidia mL−1 suspension exposed to 4.2 mg O3 L−1 exhibited n values < 1, thus evidencing that the most sensitive population members were rapidly inactivated, while the remaining cells were intrinsically more resistant or adapted to the stress factor. On the contrary, a value of n > 1 was attained when the lowest ozone concentration was applied to high conidial loads (2.5 mg O3 L−1, 105 conidia mL−1), reflecting a slight inactivation at low ozone doses and an increased cell sensitivity at higher doses.
The frequency distributions of conidia resistance to the different treatments were strongly skewed towards low ozone doses, showing a slight mode only the inactivation curve of conidial suspensions of 105 cells mL−1 exposed to 2.5 mg O3 L−1 (Supplementary Figure S1; ). The mean inactivation dose diminished when ozonization was performed on suspensions with low conidial load and high ozone concentration. Moreover, the distributions corresponding to ozone treatments of the highest microorganism’s concentration showed a high variance, which would indicate a greater heterogeneity in the response of the population to the treatments ().
3.2. Physiological changes of ozone-treated B. cinerea conidia
A dual labeling with FDA and PI were used in flow cytometry analysis in order to assess the viability and membrane integrity of conidia subjected to ozone treatments. FDA is a non-polar molecule that can cross the cell membranes and in the cytoplasm of metabolically active cells is hydrolyzed by non-specific intracellular esterases to release a polar fluorescein molecule (F), which accumulates in the cytoplasm and gives a green fluorescence. Otherwise, PI can pass through cells via damaged membranes of injured or dead cells, and binds to DNA and RNA generating red fluorescence when excited by blue light (Butt et al., Citation1989).
The fluorescence density plots of B. cinerea conidia staining with PI (y-axis) and FDA (x axis) after being exposed to different ozone doses are shown in . As expected, most of the non-treated (control) conidia population (59.7%) presented esterase activity and intact membranes (quadrant 4) (). A smaller percentage of control cells (27.9%) were located in quadrant 3 (esterase activity not detectable, intact membranes) (), which could be linked with poor labeling of conidia with the fluorescence probes and/or the presence of dormant cells with no esterase activity (Brul et al., Citation1997). The exposure to 2.5 mg O3 L−1 − 2 min led to a displacement of a large percentage of conidia population from quadrant 4 to quadrant 2 (43.9%) (). Thus, this subpopulation still retained the metabolic activity despite showing signs of membrane injury. As previously reported, double staining with F and PI would reveal the occurrence of sublethal cell damage and the presence of viable but not-culturable cells (VBNC state), which could be repaired and undergo recovery under suitable conditions (Ramamurthy et al., Citation2014; Romero-Bernal et al., Citation2019). Furthermore, 27.1% and 7.9% of the population gated in quadrant 4 (PI-, F+) and quadrant 1 (PI+, F-), respectively, thus demonstrating the heterogeneous response of conidia to this ozone dose. Increasing the ozone exposure to 4 min resulted in a marked damage of conidia population (), showing in the 53.8% of cells the loss of esterase activity and compromised membranes (quadrant 1).
Figure 2. Fluorescence density plots of B. cinerea conidia stained with PI and FDA and exposed to aqueous ozone treatments at different concentration and times. a) control; b) 2.5 mg O3L−1 −2 min; c) 2.5 mg O3L−1 −4 min; d) 4.2 mg O3L−1 −2 min; e) 4.2 mg O3L−1 −4 min; f) 4.2 mg O3L−1 −10 min.
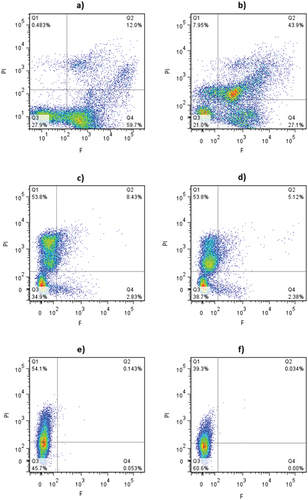
Conidia treated with the highest ozone concentration (4.2 mg O3 L−1) showed a similar response with increasing ozonization time as that described above, but the rise in the population PI (+) and F (-) was observed at shorter treatment times (). Curiously, for extended ozone exposure times, the percentage of conidia solely labelled with PI diminished, increasing simultaneously the number of unstained cells gated in quadrant 3 (). Fröhling and Schlüter (Citation2015) also reported a similar effect when monitoring by multiparametric flow cytometry the impact of aqueous ozone treatments (3.8 mg O3 L−1 − 2 min) in Escherichia coli, Listeria innocua, and Pectobacterium carotovarum spp. carotovarum. The authors attributed the loss of staining to an excessive permeabilization of the bacteria by ozone, which could have led to cell lysis, or severe nucleic acids damage that could have prevented the intercalation of PI probe with the DNA/RNA.
Dot plots of side-scattered light (SSC) vs forward-scattered light (FSC) parameters also revealed modifications in the complexity and size of conidia population exposed to different ozone doses (). Control cell population showed a high heterogeneity in size (FSC) and granularity or complexity (SSC) (). A direct relationship between cell size and cellular complexity was observed, i.e. smaller conidia showed less complexity. The same relationship has also been reported by other authors in different microorganisms (Hewitt et al., Citation2001). Furthermore, heterogeneity within the control population could be attributed to the presence of spores of different age and, consequently, with different size and cytoplasmic composition (Brul et al., Citation1997; Dijksterhuis, Citation2017). Conidia population exposed to ozone tended to shift towards lower SSC and slightly higher FSC values than control samples with increasing ozone concentration and treatment time ().
3.3. Ultrastructure features of ozone treated B. cinerea conidia
Electron micrographs of untreated and ozonized B. cinerea conidia are shown in . Control conidia, oval in shape, were observed with well-preserved and defined structures (). The bilayer architecture of the cell walls with the plasma membrane closely attached to them, and numerous mitochondria, nuclei, lipid and storage bodies, and stellate vacuoles were recognized in the cells ().
Figure 4. Transmission electronic microscopy images of Botrytis cinerea conidia exposed to aqueous ozone at different concentration and treatment times. (a,b) control; (c,d) 2.5 mg O3 L−1 −2 min; (e,f) 2.5 mg O3 L−1 −4 min; (g,h) 4.2 mg O3 L−1 −2 min; (i,j) 4.2 mg O3 L−1 −4 min; (k,l) 4.2 mg O3 L−1 −14 min. Scale: a, b, c, 0.5 µm; d, f, g, l, 1 µm; e, h, i, j, k, 2 µm. ITW: inner wall; OTW: outer wall; pr: surface protuberance; p: plasmalemma; N: nuclei; L: lipid body; SB: storage body; SV: stellate vacuole; cd: cell debris. The arrow indicates broken cell wall (H).
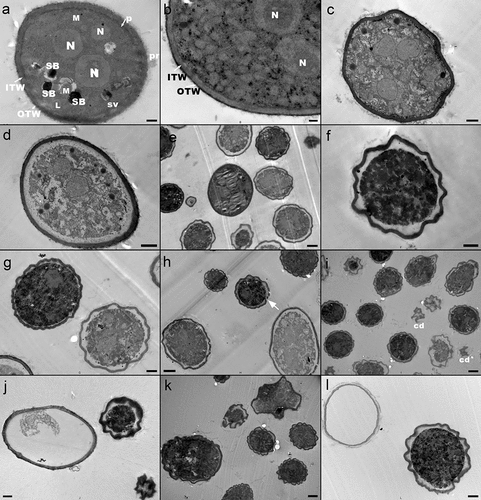
Ozone-exposed B. cinerea conidia showed varying levels of structural modifications as a function of ozone concentration and treatment time, which were in agreement with flow cytometry studies (). Cells treated with 2.5 mg O3 L−1- 2 min appeared with heterogeneous structure alterations (), consistent with the diverse labeling observed in the physiology of this population in FCM. Some cells were seen with slight cell walls folding and mild disruption of plasmalemma (), while others showed a completely disrupted plasmalemma and deteriorated cytoplasm with damaged organelle membranes (). Nonetheless, most of conidia appeared with conspicuous staining of the inner and outer cell walls, which may indicate that the treatment caused a rearrangement or chemical modifications of the cell wall polymers. With increasing exposure time (2.5 mg O3 L−1 − 4 min), cells were observed with the cytoplasm detached from the cell wall and severely disrupted, and with electrodense but noticeably undulated cell walls (). This wrinkling effect of ozone on cell surface was also seen in other fungal spores (Kang et al., Citation2015; Wen et al., Citation2020) and bacteria (Hunt & MariñMariñAs, Citation1999; Rangel et al., Citation2022).
Exposure of conidia to higher ozone concentration (4.2 mg O3 L−1) led to more severe damage to their structure, which became already evident at shorter exposure times (). After 4 min-treatment, cells were visualized in general with marked folding in cell walls, but strikingly still showed good electron density. Some cells appeared with disruptions in cell walls, absent or barely detectable cytoplasmic content, or completely collapsed (). The effect of ozone on the cytoplasmic content could explain the loss of staining with the PI probe and the depletion of SSC values of conidia exposed to increasing ozone doses. The disorder in cell walls was accentuated by the application of 14-min treatment (). TEM image in clearly showed a cell without intracellular content and low electronic-dense walls with several episodes of rupture.
These findings demonstrated that ozone attacks multiple cellular components in a dose-dependent manner. Oxidative damage to cellular structures would be mediated by molecular ozone (direct pathway), and free radicals, especially hydroxyl radicals, generated by the ozone breakdown (indirect pathway) (Glaze, Citation1986). These species would promote the oxidation of different cellular biomolecules, including proteins, lipids and nucleic acids, leading to cell death, and ultimately to cell lysis (Xue et al., Citation2023). In fungal spores, Pagès et al. (Citation2015) found a correlation between the inactivation rates and peroxidation of the membrane polyunsaturated phospholipids that would relate to cell membrane disruption. Moreover, a significant increment of intracellular ROS in response to the exposure to low ozone doses has been described in fungal spores and bacteria, which would contribute to the oxidative damage to cellular biomolecules (Kang et al., Citation2015; Rangel et al., Citation2022; Savi & Scussel, Citation2014)
3.4. Incidence of Botrytis cinerea in ozone-treated strawberry cultivars
The percentage of ozone treated and untreated strawberries of the cultivars Fortuna and Aroma with grey mold growth throughout refrigerated storage are presented in , respectively. No significant interactions between the factors “treatment” and “storage day” were found in both strawberry cultivars (p > .8). In cv. Fortuna, although there was a significant increase in the percentage of infected fruit in control and treated samples over storage, B. cinerea incidence was significantly lower in fruits ozonized for 5 and 10 min with 4.4 mg O3 L−1 until 8 day of storage, and no significant differences between treatment times were found (). In addition, the 5 min-treatment resulted in a 4-day delay on the onset of infection compared to the control. The percentage of infected fruits in the 5 and 10 min-ozonized strawberries stored for 8 days was about 20% and 13%, respectively, while in the control 33%. At the end of storage (day 10), B. cinerea incidence raised up in all samples to 60% (). Unlike cv. Fortuna, ozonized and non-ozonized strawberries of cv. Aroma showed a pronounced increase in the percentage of infection from day 1 of storage, reaching 90–100% of infection after 8-day storage (). Moreover, no significant differences in B. cinerea decay among the ozonized samples (5 and 10 min) and control were found throughout the whole storage period.
Figure 5. Botrytis cinerea incidence over storage at 6 ± 1°C in artificially inoculated strawberries cv. Fortuna (a) and Aroma (b) subjected to aqueous ozone (4.4 mg O3 L−1) during different exposure times. (●) Control; (◼) 5 min O3; (▲) 10 min O3.
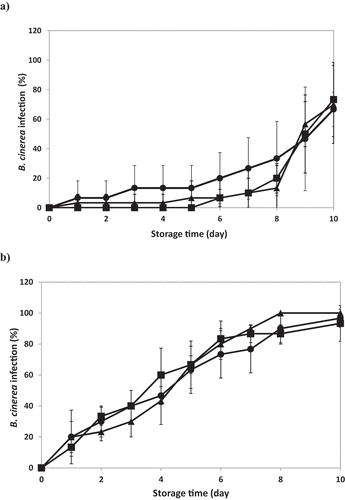
In our previous studies carried out with strawberries cv. Albion inoculated with B. cinerea and subjected to aqueous ozone treatments under similar operative conditions than those used in this work, we found a positive effect on the reduction of the mold growth similar to that observed for the cv. Fortuna (Contigiani et al., Citation2020). In addition, the treatment did not significantly affect the colour, mechanical and antioxidant properties of the fruits (Contigiani et al., Citation2018, Citation2020). To the best of our knowledge, there are no other studies in which the effect of aqueous ozone treatments on the development of B. cinerea on strawberries has been evaluated.
When comparing the effects of aqueous ozone treatments on B. cinerea conidia in suspension and inoculated in strawberries, it could be observed, as expected, that the effectiveness of treatments was considerably lower when applied to the fruit than in planktonic systems. This loss of effectiveness could be a consequence of the interaction of ozone with other components of the fruit matrix, as well as to the presence of cracks and/or irregularities on the fruit surface that would protect the microorganisms from the direct contact with the ozone.
Many factors can explain, at least partially, the different response found in the Aroma and Fortuna cultivars. The effectiveness of ozone when applied to fruits may be due not only to the direct oxidative damage caused to microorganisms, but also to the induction of complex defense response mechanisms (both constitutive and inducible resistance mechanisms) in the fruit. This response has been shown to be dose-dependent and would involve the induction of antioxidant systems, the accumulation of phenolic compounds with antimicrobial activity and pathogenesis-related proteins, and the reinforcement of tissues cell walls (Kangasjärvi et al., Citation1994; Muthusamy & Lee, Citation2024; Sandermann et al., Citation1998; Zhao et al., Citation2022). Phenolic acids, flavonoids, stilbenoids, and lignans have demonstrated to be involved in abiotic stress responses and their role in defense systems are commonly attributed to their antioxidant activity. Plant cell wall is the first line of defense that B. cinerea faces, and the fungus should secrete cell wall – degrading enzymes to degrade pectic polysaccharides of the fruit cell wall to initiate infection. Induced resistance depends on the interaction of the fungus with the host’s ability to initially inhibit the degradation of pectic substances. The ability for ozone-induced resistance may be dependent on retaining or increasing preformed antifungal defenses and on slowing the rate of cell wall depolymerization. For example, Contigiani et al. (Citation2018) reported that the most effective ozone dose for reducing the growth of B. cinerea on strawberries cv. Albion fruit led to an increase in the thickness of the cuticle membrane of fruit epidermal cells, a phenomenon that could be associated with the stimulation of defense mechanisms.
Genetic, environmental, and agronomic factors influence secondary plant metabolites (Bestfleisch et al., Citation2015; Muthusamy & Lee, Citation2024). Fruit cultivars (genetic background) have distinctive structure and chemical, physical, and phytochemical properties (Šamec et al., Citation2021, Citation2016). In addition, soil, preharvest nutrition, and harvesting time are not minor variables. Thus, the development of constitutive, active, and inducible defense systems to mitigate the stressors is very complex and the efficacy of ozone would be individually assessed for each cultivar.
4. Conclusions
This study allows a better understanding of the effect of aqueous ozone on B. cinerea conidia and its potential application for reducing the fungus incidence during strawberries postharvest. The findings can be summarized as follows:
– High inactivation efficiency of ozone (2.5 ± 0.8 and 4.2 ± 0.2 mg O3 L−1, 0.5–16 min) was demonstrated in in vitro tests. Non-linear inactivation kinetics of conidial aqueous suspensions was successfully fitted with the Weibullian model, indicating a distribution of the resistance of conidia in the population. Inactivation parameters and distribution statistic descriptors varied with the ozone level, the contact time and the conidia load. The fungal population was inactivated at lower exposure times, the higher the concentration of dissolved ozone and the lower the concentration of conidia.
– The mode of action of ozone depended on the dose and included various structure targets and changes in the physiological state, size, and complexity of the conidia cells. FCM revealed that the response of the conidia population to ozone was very heterogeneous at low doses, coexisting cell populations of varying levels of membrane damage and/or loss of metabolic activity. However, high ozone doses caused death of spores by destroying the integrity of the cell membrane and inhibiting the enzymatic activity. Two subpopulations were labeled in this case, one corresponding to cells with membrane disruption and one without fluorescence, which would be integrated by cells without cytoplasmic content-including nucleic acids- and cellular debris. Images of treated conidia obtained by TEM were in agreement with FCM findings. In general, at low exposure times, ozone affected the walls and damaged membranes, although changes were not uniform in the population. As ozone dose increased, a severe damage to walls and membranes, degradation of organelles and intracellular material and loss of cytoplasmic content were registered.
– Ozonation (4.4 mg O3L−1, 5–10 min) delayed the onset of infection (at least 4-day delay) and reduced the incidence of B. cinerea during 8-day storage compared with the untreated fruit in Fortuna cv., but the treatment was not effective in Aroma cv. The effect of the cultivar on the performance of aqueous ozone treatments requires further elucidation.
Supplemental Material
Download MS Word (35.1 KB)Disclosure statement
No potential conflict of interest was reported by the author(s).
Supplementary material
Supplemental data for this article can be accessed online at https://doi.org/10.1080/19476337.2024.2339446
Additional information
Funding
References
- Aslam, R., Alam, M. S., & Saeed, P. A. (2020). Sanitization potential of ozone and its role in postharvest quality management of fruits and vegetables. Food Engineering Reviews, 12(1), 48–11. https://doi.org/10.1007/s12393-019-09204-0
- Bardas, G. A., Veloukas, T., Koutita, O., & Karaoglanidis, G. S. (2010). Multiple resistance of Botrytis cinerea from kiwifruit to SDHIs, QoIs and fungicides of other chemical groups. Pesticide Management Science, 66(9), 967–973. https://doi.org/10.1002/ps.1968
- Bestfleisch, M., Luderer-Pfimpfl, M., Höfer, M., Schulte, E., Wünsche, J. N., Hanke, M. V., & Flachowsky, H. (2015). Evaluation of strawberry (Fragaria L.) genetic resources for resistance to Botrytis cinerea. Plant Pathology, 64(2), 396–405. https://doi.org/10.1111/ppa.12278
- Bi, K., Liang, Y., Mengiste, T., & Sharon, A. (2023). Killing softly: A roadmap of Botrytis cinerea pathogenicity. Trends in Plant Science, 28(2), 211–222. https://doi.org/10.1016/j.tplants.2022.08.024
- Brul, S., Nussbaum, J., & Dielbandhoesing, S. K. (1997). Fluorescence probes for wall porosity and membrane integrity in filamentous fungi. Journal of Microbiological Methods, 28(3), 169–178. https://doi.org/10.1016/S0167-7012(97)00975-5
- Butt, T. M., Hoch, H. C., Staples, R. C., & Leger, R. J. (1989). Use of fluorochromes in the study of fungal cytology and differentiation. Experimental Mycology, 13(4), 303–320. https://doi.org/10.1016/0147-5975(89)90026-1
- Contigiani, E. V., Jaramillo- Sánchez, G., Castro, M. A., Gómez, P. L., & Alzamora, S. M. (2018). Postharvest quality of strawberry fruit (Fragaria x Ananassa Duch cv. Albion) as affected by ozone washing: Fungal spoilage, mechanical properties, and structure. Food and Bioprocess Technology, 11(9), 1639–1650. https://doi.org/10.1007/s11947-018-2127-0
- Contigiani, E. V., Kronberg, M. F., Jaramillo-Sánchez, G., Gómez, P. L., Garcia-Loredo, A. B., Munarriz, E., & Alzamora, S. M. (2020). Ozone washing decreases strawberry susceptibility to Botrytis cinerea while maintaining antioxidant, optical and sensory quality. Heliyon, 6(11), e05416. https://doi.org/10.1016/j.heliyon.2020.e05416
- Cosseboom, S. D., Ivors, K. L., Schnabel, G., Bryson, P. K., & Holmes, G. J. (2019). Within-season shift in fungicide resistance profiles of botrytis cinerea in California Strawberry fields. Plant Disease, 103(1), 59–64. https://doi.org/10.1094/PDIS-03-18-0406-RE
- Dean, R., Van Kan, J. A. L., Pretorius, Z. A., Hammond-Kosack, K. E., DiPietro, A., Spanu, P. D., Rudd, J. J., Dickman, M., Kahmann, R., Ellis, J., & Foster, G. D. (2012). The top 10 fungal pathogens in molecular plant pathology. Molecular Plant Pathology, 13(4), 414–430. https://doi.org/10.1111/j.1364-3703.2011.00783.x
- Dijksterhuis, J. (2017). The fungal spores and food spoilage. Current Opinion in Food Science, 17, 68–74. https://doi.org/10.1016/j.cofs.2017.10.006
- Epelle, E. I., Macfarlane, A., Cusack, M., Burns, A., Okolie, J. A., Mackay, W., Rateb, M., & Yaseen, M. (2023). Ozone application in different industries: A review of recent developments. Chemical Engineering Journal, 5(454), 140188. https://doi.org/10.1016/j.cej.2022.140188
- Fröhling, A., & Schlüter, O. (2015). Flow cytometric evaluation of physico-chemical impact on gram-positive and gram-negative bacteria. Frontiers in Microbiology, 6, 939. https://doi.org/10.3389/fmicb.2015.00939
- Garcia-Loredo, A. B., Guerrero, S. N., & Alzamora, S. M. (2015). Inactivation kinetics and growth dynamics during cold storage of Escherichia coli ATCC 11229, listeria innocua ATCC 33090 and Saccharomyces cerevisiae KE162 in peach juice using aqueous ozone. Innovative Food Science and Emerging Technologies, 29, 271–279. https://doi.org/10.1016/j.ifset.2015.02.007
- Glaze, W. H. (1986). Reaction products of ozone: A review. Environmental Health Perspectives, 69, 151–157. https://doi.org/10.1289/ehp.8669151
- Hewitt, C. J., Bellara, S. R., Andreani, A., Nebe von Caron, G., & Mcfarlane, C. M. (2001). An evaluation of the anti-bacterial action of ceramic powder slurries using multiparameter flow cytometry. Biotechnology Letters, 23(9), 667–675. https://doi.org/10.1023/A:1010379714673
- Hunt, N. K., & MariñMariñAs, B. J. (1999). Inactivation of Escherichia coli with ozone: Chemical and inactivation kinetics. Water Research, 33(11), 2633–2691. https://doi.org/10.1016/S0043-1354(99)00115-3
- Kang, M. H., Pengkit, A., Choi, K., Jeon, S. S., Choi, H. W., Shin, D. B., Choi, E. H., Uhm, H. S., Park, G., & Wang, Z. (2015). Differential inactivation of fungal spores in water and on seeds by ozone and arc discharge plasma. PLOS ONE, 10(9), e0139263. https://doi.org/10.1371/journal.pone.0139263
- Kangasjärvi, J., Talvinen, J., Utriainen, M., & Karjalainen, R. (1994). Plant defence systems induced by ozone. Plant, Cell & Environment, 17(7), 783–794. https://doi.org/10.1111/j.1365-3040.1994.tb00173.x
- Li, H., Larsen, D. L., Cao, R., van de Peppel, A. C., Tikunov, Y. M., Marcelis, L. F. M., Woltering, E. J., van Kan, J. A. L., & Schouten, R. E. (2022). The association between the susceptibility to Botrytis cinerea and the levels of volatile and non-volatile metabolites in red ripe strawberry genotypes. Food Chemistry, 393, 133252. https://doi.org/10.1016/j.foodchem.2022.133252
- Minas, I. S., Karaoglanidis, G. S., Manganaris, G. A., & Vasilakakis, M. (2010). Effect of ozone application during cold storage of kiwifruit on the development of stem-end rot caused by botrytis cinerea. Postharvest Biology and Technology, 58(3), 203–210. https://doi.org/10.1016/j.postharvbio.2010.07.002
- Muthusamy, M., & Lee, S. I. (2024). Abiotic stress-induced secondary metabolite production in Brassica: Opportunities and challenges. Frontiers in Plant Science, 14, 1323085. https://doi.org/10.3389/fpls.2023.1323085
- Nadas, A., Olmo, M., & García, J. M. (2003). Growth of Botrytis cinerea and strawberry quality in ozone‐enriched atmospheres. Journal of Food Science, 68(5), 1798–1802. https://doi.org/10.1111/j.1365-2621.2003.tb12332.x
- Orozco-Mosqueda, M. C., Kumar, A., Fadiji, A. E., Babalola, O. O., Puopolo, G., & Santoyo, G. (2023). Agroecological management of the grey mould fungus Botrytis cinerea by plant growth-promoting bacteria. Plants, 12(3), 637. https://doi.org/10.3390/plants12030637
- Pagès, M., Kleiber, D., Pierron, R. J., & Violleau, F. (2015). Ozone effects on Botrytis cinerea conidia using a bubble column: Germination inactivation and membrane phospholipids oxidation. Ozone: Science & Engineering, 38(1), 62–69. https://doi.org/10.1080/01919512.2015.1074856
- Pandiselvam, R., Subhashini, S., Banuu Priya, E. P., Kothakota, A., Ramesh, S. V., & Shahir, S. (2019). Ozone based food preservation: A promising green technology for enhanced food safety. Ozone: Science & Engineering, 41(1), 17–34. https://doi.org/10.1080/01919512.2018.1490636
- Peleg, M. (1999). On calculating sterility in thermal and non-thermal preservation methods. Food Research International, 32(4), 271–278. https://doi.org/10.1016/S0963-9969(99)00081-2
- Peleg, M., & Cole, M. B. (1998). Reinterpretation of microbial survival curves. Critical Reviews in Food Science and Nutrition, 38(5), 353–380. https://doi.org/10.1080/10408699891274246
- Petrasch, S., Knapp, S. J., van Kan, J. A. L., & Blanco-Ulate, B. (2019). Grey mould of strawberry, a devastating disease caused by the ubiquitous necrotrophic fungal pathogen Botrytis cinerea. Molecular Plant Pathology, 20(6), 877–892. https://doi.org/10.1111/mpp.12794
- Ramamurthy, T., Ghosh, A., Pazhani, G. P., & Shinoda, S. (2014). Current perspectives on viable but non-culturable (VBNC) pathogenic bacteria. Frontiers in Public Health, 2, 103. https://doi.org/10.3389/fpubh.2014.00103
- Rangel, K., Cabral, F. O., Lechuga, G. C., Carvalho, J. P. R. S., Villas- BôBôAs, M. H. S., Midlej, V., & De Simone, S. G. (2022). Detrimental effect of ozone on pathogenic bacteria. Microorganisms [Internet], 10(1), 40. https://doi.org/10.3390/microorganisms10010040
- Romero-Bernal, A. R., Contigiani, E. V., González, H. H. L., Alzamora, S. M., Gómez, P. L., & Raffellini, S. (2019). Botrytis cinerea response to pulsed light: Cultivability, physiological state, ultrastructure and growth ability on strawberry fruit. International Journal of Food Microbiology, 309, 1–8. https://doi.org/10.1016/j.ijfoodmicro.2019.108311
- Šamec, D., Karalija, E., Šola, I., Vujčić Bok, V., & Salopek-Sondi, B. (2021). The role of polyphenols in abiotic stress response: The influence of molecular structure. Plants, 10(1), 118. https://doi.org/10.3390/plants10010118
- Šamec, S., Maretic’, M., Lugaric’, I., Mešic’, A., Salopek-Sondi, B., & Duralija, B. (2016). Assessment of the differences in the physical, chemical and phytochemical properties of four strawberry cultivars using principal component analysis. Food Chemistry, 194, 828–834. https://doi.org/10.1016/j.foodchem.2015.08.095
- Sandermann, H. J., Ernst, D., Heller, W., & Langebartels, C. (1998). Ozone: An abiotic elicitor of plant defense reactions. Trends in Plant Science Reviews, 3(2), 47–50. https://doi.org/10.1016/S1360-1385(97)01162-X
- Savi, G. D., & Scussel, V. M. (2014). Effects of ozone gas exposure on toxigenic fungi species from fusarium, Aspergillus, and Penicillium genera. Ozone: Science & Engineering, 36(2), 144–152. https://doi.org/10.1080/01919512.2013.846824
- Tuffi, R., Lovino, R., Canese, S., Cafiero, L. M., & Vitali, F. (2012). Effects of exposure to gaseous ozone and negative air ions on control of epiphytic flora and the development of Botrytis cinerea and Penicillium expansum during cold storage of strawberries and tomatoes. Italian Journal of Food Science, 4(2), 102.
- Tzortzakis, N. (2023). Preservation of fresh strawberries in an ozone-enriched atmosphere. Acta Horticulturae, 1363(1363), 212–220. https://doi.org/10.17660/ActaHortic.2023.1363.32
- Veloso, J., & van Kan, J. A. L. (2018). Many shades of grey in Botrytis–host plant interactions. Trends in Plant Sciences, 23(7), 613–622. https://doi.org/10.1016/j.tplants.2018.03.016
- Wen, G., Liang, Z., Xu, X., Cao, R., Wan, Q., Ji, G., Lin, W., Wang, J., Yang, J., & Huang, T. (2020). Inactivation of fungal spores in water using ozone: Kinetics, influencing factors and mechanisms. Water Research, 185, 116218. https://doi.org/10.1016/j.watres.2020.116218
- Xue, W., Macleod, J., & Blaxland, J. (2023). The use of ozone technology to control microorganism growth, enhance food safety and extend shelf life: A promising food decontamination technology. Foods, 12(4), 814. https://doi.org/10.3390/foods12040814
- Zhao, Y., Vlasselaer, L., Ribeiro, B., Terzoudis, K., Van den Ende, W., Hertog, M., Nicolaï, B., & De Coninck, B. (2022). Constitutive defense mechanisms have a major role in the resistance of woodland strawberry leaves against botrytis cinerea. Frontiers in plant science, 13, 912667. https://doi.org/10.3389/fpls.2022.912667
- Zhou, D., Wang, Z., Peng, S., Chen, J., & Tu, K. (2019). Effects of cold plasma, UV-C or aqueous ozone treatment on Botrytis cinerea and their potential application in preserving blueberry. Journal of Applied Microbiology, 127(1), 175–185. https://doi.org/10.1111/jam.14280