ABSTRACT
The neonatal intestinal tract is immature and can be easily infected by pathogens causing inflammation. Maternal diet manipulation is a promising nutritional strategy to enhance the gut health of offspring. A fermented diet is a gut microbiota targeting diet containing live probiotics and their metabolites, which benefit the gut and overall health host. However, it remains unclear how a maternal fermented diet (MFD) affects neonatal intestinal inflammation. Here, in vivo and in vitro models together with multi-omics analysis were applied to investigate the impacts and the underlying mechanism through which an MFD prevents from gut inflammation in neonates. An MFD remarkably improved the performance of both sows and piglets and significantly altered the gut microbiome and milk metabolome of sows. In addition, the MFD significantly accelerated the maturation of the gut microbiota of neonates and increased the abundance of gut Lactobacillus and the microbial functions of amino acid-related enzymes and glucose metabolism on the weaning day. Notably, the MFD reduced susceptibility to colonic inflammation in offspring. The fecal microbiota of sows was then transplanted into mouse dams and it was found that the mouse dams and pups in the MFD group alleviated the LPS-induced decrease in gut Lactobacillus abundance and barrier injury. Milk L-glutamine (GLN) and gut Lactobacillus reuteri (LR) were found as two of the main MFD-induced sow effectors that contributed to the gut health of piglets. The properties of LR and GLN in modulating gut microbiota and alleviating colonic inflammation by inhibiting the phosphorylation of p38 and JNK activation of Caspase 3 were further verified. These findings provide the first data revealing that an MFD drives neonate gut microbiota development and ameliorates the colonic inflammation by regulating the gut microbiota. This fundamental evidence might provide references for modulating maternal nutrition to enhance early-life gut health and prevent gut inflammation.
Introduction
Early life is a critical period in which the gut microbiota, the physical barrier functions of the intestine, and the immune system are under development in infants. There is increasing evidence that the maturation and health of the neonatal gut may play an important role in programming health and disease later in life.Citation1 Disturbances in early-life gut development have been found to be associated with the occurrence of inflammatory gut injury in both children and adults.Citation2–4 Maternal diet can affect early postnatal gut development and health primarily through milk and gut microbiota.Citation5,Citation6 Maternal milk affects the maturation of the gut in infants by supplying basic nutrients and bioactive components such as immunoglobulin, oligosaccharides, short-chain fatty acids, antimicrobial peptides, and microbes.Citation7 The maternal gut microbiota shapes the early-life gut microbiota of the offspring through the entero-mammary axisCitation8 and drives early postnatal innate immune development and metabolic phenotypes.Citation9,Citation10
Dietary variables can dramatically and rapidly affect the gut microbiome.Citation11 Fermented foods, such as kombucha, yogurt, and kimchi, constitute one type of gut microbiota–targeting diet, containing not only foundational nutrients (carbohydrates, proteins, and fats) but also probiotics, prebiotics, and microbial metabolites.Citation12 Large cohort studies and limited interventional studies have correlated fermented food consumption with weight maintenance and reduced risk of diabetes, cancer and cardiovascular disease.Citation13,Citation14 A recent study found differences in gut microbial structure and metabolome between consumers of fermented and non-fermented foods.Citation15 The benefits of a fermented diet on gut and host health have been reported,Citation16 while the effects of a maternal fermented diet (MFD) on gut health in offspring remain undetermined.
In the present study, in vivo and in vitro models were used to investigate how MFD protects against early-life intestinal inflammation by applying multi-omics, fecal microbiota transplantation (FMT), and lipopolysaccharide (LPS)-induced colonic inflammation. These results will expand our knowledge of how MFD influences the gut health of infants during early life and provide underlying strategies to prevent early-life gut inflammatory injury with lasting consequences.
Materials and methods
Production of maternal fermented diet
The substrate for fermentation consisted of corn, soybean meal, and wine lees (2:2:1). Sterile water was added to achieve an optimal moisture content of 40%. Bacillus subtilis CW4 (NCBI Accession No. MH885533, 1 × 108 CFU/g) and Enterococcus faecalis CWEF (NCBI Accession No. MN038173, 1 × 108 CFU/g) were included in the diet to promote fermentation over the course of 3 days. Moist samples (approximately 100 g) were collected to determine the numbers of microorganisms and 16S rRNA gene sequencing, and the remainder of the sample material was dried at 60°C for 24 h, cooled, ground, and subjected to conventional nutrient analysis. Dried samples were collected for further analysis of the crude protein (CP), neutral detergent fiber (NDF), acid detergent fiber (ADF) and amylose content using the AOAC International guidelines.Citation17 Lactate was detected using a lactic acid assay kit (Nanjing Jiancheng Bioengineering Institute, Nanjing) following the manufacturer’s instructions. The nutrient content of the MFD is shown in Table S1.
Experimental design
In vivo
All the animal assays were approved by the Institutional Animal Care and Use Committee at Zhejiang University.
Sixty Yorkshire × Landrace sows were randomly divided into three groups and fed different diets from 7 days before parturition to the day of weaning (). The groups were as follows: (i) control (CON) group (control diet, n = 20), (ii) MFD group (basal diet + 10% MFD, n = 20) and (iii) probiotic (PROB) group (basal diet + equal amounts of B. subtilis and E. faecalis, n = 20). For the PROB group, 3.4 g/kg of B. subtilis powder and 1.3 g/kg of E. faecalis powder were supplied in the drinking water. The diets had the same amounts of crude protein and digestive energy and met the NRC (2012) nutrient requirement.Citation18 The ingredients and nutritional values are shown in Table S3. Sow feces were collected on day 28, and piglet feces were obtained on days 7, 14 and 28 (with the count beginning 7 days before parturition).
Figure 1. Study design for the whole experiment. (a) Schematic diagram of the fermented diet and probiotics administered to sows (n = 20). (b) Schematic diagram of the susceptibility of piglets to LPS-induced colonic inflammation (n = 6). (c) Schematic diagram of the effects of the transplantation of sows’ fecal microbiota on gut health in mouse dams and pups (n = 6). (d) Schematic diagram of the effects of LR and GLN on the gut health of mice (n = 6). (e) Schematic diagram of the effects of LR and GLN on inflammation in 3D4/2 and Caco-2 cells (n = 3). PBS, phosphate-buffered saline; LPS, lipopolysaccharides; FMT, fecal microbiota transplantation; LR, Lactobacillus reuteri; GLN, L-glutamine.
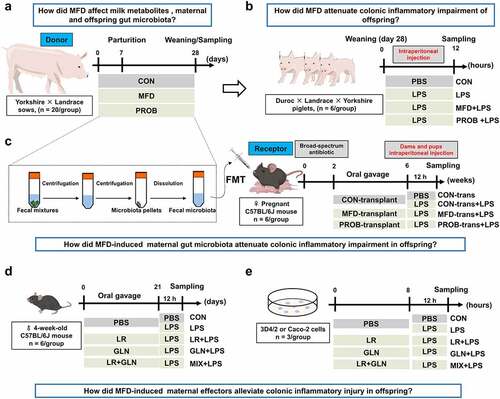
To examine how MFD affects colonic inflammation in offspring, piglets were challenged with lipopolysaccharide (LPS, Sigma–Aldrich, St. Louis, MO, USA) (). On day 28, healthy piglets with similar weights were randomly selected from the CON, MFD, and PROB groups. Piglets in each group were intraperitoneally injected with 10 mg/kg LPS (n = 6) Citation19 for 12 h or, in the case of six piglets in the CON group, an equivalent amount of PBS (n = 6). Piglets were divided into CON, LPS, MFD + LPS, and PROB + LPS groups; slaughtered and sampled 12 h after LPS challenge.
To further investigate whether an MFD could affect early-life gut health by regulating the maternal gut microbiome, FMT was performed in a mouse model (). Twenty-four healthy, pregnant, specific-pathogen-free (SPF) female C57BL/6J mice were divided into four groups, with 6 mice per group. After 2 weeks of broad-spectrum antibiotic treatment (50 µg/mL clindamycin, 50 µg/mL metronidazole, 50 µg/mL penicillin, 25 µg/mL vancomycin and 50 µg/mL neomycin (Sigma, St Louis, USA)) in sterile drinking water, the dams were intragastrically dosed with a sow fecal microbiota suspension every other day (n = 6). At the time of weaning on day 21, the dams and pups (n = 6) were intraperitoneally injected with 10 mg/kg LPS, and feces and colon samples were collected 12 h later. Dams and pups were divided into CON-trans, CON-trans + LPS, MFD-trans + LPS and PROB-trans + LPS groups.
Mice were further used to verify the properties of maternal effectors in vivo (). Thirty healthy 4-week-old SPF C57BL/6J male mice were allotted into five groups (n = 6). They were pre-fed for one week and treated with 1 ×108 CFU/d Lactobacillus reuteri (LR, ATCC 53608), 300 mg/kg L-glutamine (GLN, Solarbio, Beijing, China), or a combination of the two agents (MIX) for 21 days. Thereafter, the mice were intraperitoneally injected with 10 mg/kg LPS for 12 h, and fecal and colon samples were collected. The groups were CON, LPS, LR + LPS, GLN + LPS, and MIX + LPS.
In vitro
Porcine 3D4/2 macrophages and human Caco-2 cells were selected to investigate the effects of maternal effectors on LPS-induced inflammation in vitro (). Cells were treated with 10 µg/mL LPS or an equivalent amount of PBS for 12 h after treatment (optimal concentration: 1 ×108 CFU/mL LR or 2.5 mM GLN) for 8 h (n = 3). Then, the total protein of the cells were collected by using a total protein extraction kit (KeyGen BioTECH, Nanjing, China).
16S sequencing and data analysis
Microbial DNA was obtained from fecal homogenates using the E.Z.N.A. Stool DNA Kit (Omega Bio-Tek, Norcross, GA, USA). To ensure that no contamination had occurred, the concentration and purity of the DNA samples were measured with a NanoDrop 2000 UV–vis spectrophotometer (Thermo Scientific, Wilmington, MA, USA) and examined by electrophoresis using 1% agarose gels. The V4 gene regions of the bacterial 16S rRNA gene were amplified with the primer pairs 515 F (5’-GTGCCAGCMGCCGCGG-3’) and 806 R (5’-GGACTACHVGGGTWTCTAAT-3’) using the Illumina MiSeq platform at Shanghai Majorbio Biopharm Technology Co., Ltd. (Shanghai, China).
The reads were preprocessed, denoised, quality controlled, and merged in DADA2.Citation20 UPARSE 7.1 and QIIME2ʹs q2-feature-classifier plugin were used to cluster the operational taxonomic units (OTUs) with 97% and 100% similarity cutoffs,Citation21 respectively, and chimeric sequences were identified and removed. The taxonomies from the phylum to genus levels were assigned by the Greengenes database.Citation22 Sequences of OTUs of interest were further confirmed through NCBI BLAST analysis. Diversity analyses were performed using QIIME1 and QIIME2.Citation23 In addition, the significantly different genera were selected by the linear discriminant analysis effect size (LEfSe) method (https://huttenhower.sph.harvard.edu/galaxy/).Citation24 To predict metabolic genes during the process, PICRUSt 1 and 2 were used to obtain functions for the genes that were predicted to be present in the samples and to assign the genes to Kyoto Encyclopedia of Genes and Genomes (KEGG) metabolic pathways.Citation25 Pearson’s rank correlation coefficient was calculated with R version 3.6.3 to evaluate the relationships among the data. To identify age-related bacteria in the gut microbiota, a random forest model was used to train the data in the control group, and then the age of the microbial community was modeled for those same taxa in all groups. The maturation index of the intestinal microbiota was calculated by the method described in a previous article.Citation26 The significangtly different microbes of the MFD are shown in Table S3.
Metabolomic profiling by LC-TOF/MS
Milk samples randomly selected from six sows in each group were used for extraction and sent for metabolomic analysis (Shanghai Biotree Biotech Co., Ltd., Shanghai, China). An equivalent volume was aliquoted from each sample, mixed to prepare the quality control sample and dried in a vacuum concentrator. In addition, methoxymethyl amine salt was mixed with the dried samples, and bis(trimethylsilyl)trifluoroacetamide was added. After the samples cooled to room temperature, fatty acid methyl ester was added to each sample and mixed. Ultra-high-performance liquid chromatography (1290 Infinity series UHPLC System, Agilent Technologies, Santa Clara, CA, USA) was applied for LC-TOF/MS analysis. A UPLC BEH amide column (internal diameter, 2.1 × 100 mm, 1.7 μm, Waters, Milford, MA, USA) was used for separation. The obtained data were applied for sparse partial least squares discriminant analysis (sPLS-DA). Differential metabolites were identified with variable importance projection (VIP) > 1.0 and P < 0.05. To further interpret the biological significance of metabolites, metabolic pathway analyses were performed by an online analysis platform in MetaboAnalyst 5.0 (https://www.metaboanalyst.ca/). KEGG analysis was conducted using the enrichment analysis sections of MetaboAnalyst.
Intestinal morphology and histology
Approximately 2 cm of the proximal colon was fixed in 4% paraformaldehyde. Colonic tissues were stained with hematoxylin and eosin. The Leica DM3000 Microsystem, and Leica Application Suite 3.7.0 (Leica, Wetzlar, Germany) were applied to observe the colonic morphology. Histological scoring was performed as described by Geng et al.Citation19
A colonic tissue sample of suitable size was placed in 2.5% glutaraldehyde and fixed overnight. The fixed sample was washed with PBS, soaked in 1% osmic acid solution for 2 h and washed with PBS. Subsequently, the sample was dehydrated in different concentrations of alcohol and dehydrated twice with absolute ethanol. The sample was transferred to a 1:1 volume mixture of ethanol and isoamyl acetate for 30 min and then transferred to pure isoamyl acetate for 1 h. After the critical point drying, the samples were coated with gold-platinum film and observed with a field emission scanning electron microscope.
The colonic tissue fixed in the osmic acid solution was washed with PBS and dehydrated in different concentrations of alcohol and acetone. The sample was placed in a mixture of pure acetone and embedding agent for 1 h, transferred to a mixture of pure acetone and embedding agent for 3 h, and finally transferred to embedding agent for 12 h. The embedded sample was heated and then sectioned with an ultrathin microtome. Finally, the sections were stained and treated with uranyl acetate and alkaline lead citrate. A suitable field of view was identified and imaged with a JEM-1011 transmission electron microscope (JEOL USA, Peabody, MA, USA).
Immunofluorescent staining and TUNEL
Colonic slides were incubated with antigen recovery solution (Vector Laboratories, Inc. Burlingame, CA, USA) for 15 min, and nonspecific binding was blocked with 5% BSA. Next, the slides were incubated with anti-ZO-1 and anti-β-catenin primary antibodies (ab96587 and ab32572, Abcam, Cambridge, MA, USA) at 4°C overnight. After being washed with PBS, slides were incubated with fluorescent dye-conjugated secondary antibodies (Abcam) for 1 h and protected from light. Finally, 4,6-diamidino-2-phenylindole (DAPI) was used to stain the nucleus. Sections were examined with a DM5000 B fluorescence microscope (Leica, Wetzlar, Germany).
After the colonic sections were deparaffinized, DNase-free proteinase K (20 μg/mL) was applied, and the sections were incubated at 37°C for 20 min. After washing with PBS, the TUNEL detection solution was added dropwise, and sections were incubated in a 37°C incubator for 2 h. After the stop solution was added to terminate the reaction, the sections were washed with PBS. Thereafter, the streptavidin-HRP working solution was added dropwise, and sections were incubated for 30 min. The sections were washed with PBS, then treated with 0.2–0.5 mL of DAB and incubated at room temperature. The specific time was determined according to the degree of color development, and images were acquired for analysis.
Western blotting
Total protein was obtained using a Protein Extraction Kit (KeyGen BioTECH, Nanjing, China). SDS–PAGE separates proteins of different sizes and electroporates them onto PVDF membranes (Millipore, Bedford, MA, USA). Membranes were blocked with 5% skimmed milk and incubated with anti-ZO-1(ER41204), anti-Occludin(R1510-33), anti-Claudin 1(ER1906-37), anti-Cleaved caspase 3(ET1602-47), anti-Bax(ER0907), anti-Bcl-2(ER1802-97), anti-iNOS(ER1706-89), anti-Arg1(ET1605-8), anti-p-p38(ER2001-52), anti-p38(ET1602-26), anti-p-JNK(ET1609-42), anti-JNK(ET1601-28), anti-p-ERK1/2(ET1603-22), anti-ERK1/2(ET1601-29), and anti-β-actin(R1207-1) antibodies at 4°C overnight(HUABIO, Hangzhou, China). After being washed with Tris Buffered Saline with Tween20 (TBST), the membranes were incubated with secondary antibodies for 1 h. Protein bands were visualized with an enhanced chemiluminescence (ECL) assay kit (Biosharp, Hangzhou, China) and measured with ImageJ software (NIH, Bethesda, MD, USA).
ELISA
Approximately 0.1 g of colonic tissue was homogenized after being centrifuged at 12,000 × g for 10 min at 4°C. Cytokine (IL-β, IFN-γ, IL-6, IL-10, TNF-α, TGF-β) concentrations in supernatants were measured with ELISA kits (Jiangsu Meibiao Biological Technology Co., Ltd., Jiangsu, China).
In vitro fermentation
Approximately 1 g of predigested MFD was suspended in 25 mL of sterile fermentation medium and boiled for 10 min. The boiled MFD solution was introduced into the anaerobic chamber, cooled to room temperature, and reduced for 2 h. Thereafter, 5 mL of sterile fermentation medium was combined with 0.1 g of fecal sample and homogenized, followed by the mixing of 2.5 mL of the homogenized fecal suspension and 2.5 mL of the MFD solution and incubation under anaerobic conditions at 37°C with 125 rpm shaking for 14 h. All fermentation steps were conducted in an anaerobic chamber (Bactron, Sheldon Manufacturing, Inc., Cornelius, OR, USA) in an anaerobic environment containing 5% CO2, 5% H2, and 90% N2.
Quantitative real-time PCR and absolute quantification of L. reuteri
Primers were used to measure the copy numbers of the 16S rRNA gene of specific bacteria (V3-V4). The Lreu gene was selected to analyze the abundance of L. reuteri. Forward and reverse primers were used as follows: 5′-CAGACAATCTTTGATTGTTTAG-3′ and 5′-GCTTGTTGGTTTGGGCTCTTC-3′.Citation27 To obtain a standard curve, plasmids containing target DNA PCR fragments of 16S rRNA and Lreu genes were diluted 10-fold. qPCR and analysis were conducted as previously described.Citation24 The ΔCt method was used to calculate the copy numbers of Lreu genes from the standard curve of 16S rRNA.
Cell culture
Caco-2 and 3D4/2 cells were obtained from ATCC Cell Bank (Shanghai, China). DMEM-F12 and RPMI-1640 medium supplemented with 10% fetal bovine serum and two antibiotics (100 U/mL penicillin and 100 µg/mL streptomycin sulfate) in 6- and 12-well plates at 37°C was used for Caco-2 and 3D4/2 cells, respectively. Cells were incubated in an incubator (Thermo Fisher Scientific, MA, USA) containing 5% CO2 until they reached approximately 80% confluence before the treatments. The specific activators of p38 (S2266), JNK (S7409), and ERK1/2 (S1013) were purchased from Selleck (TX, USA).
Transepithelial electrical resistance (TER) and in vitro gut paracellular permeability
Transwell filters (Corning, NY, USA) were used to culture Caco-2 cells. When the TER stabilized, the cells were used for subsequent experiments. The TER was determined using a Millicell ERS Voltohmmeter (Millipore, Burlington, MA, USA) at 0, 2, 4, 6, 8, 10, and 12 h after LPS stimulation. Changes were analyzed as a fold of TER at 0 h. Approximately 100 µL of 4-kDa FD4 (1 mg/mL) was added to the apical chamber after the last TER measurement. The plates were placed in a humidified incubator for 30 min. The prepared FD4 solution was serially diluted to produce concentrations of 5, 10, 20, 40, 80, 160, 320, 640, and 1,280 ng/mL. Subsequently, the level of FD4 in the basolateral chamber was measured (excitation wavelength, 492 nm; emission wavelength, 520 nm).
Statistical analysis
The nutritional value of the MFD, animal performance and molecular biological data are presented as the mean ± standard error of the mean as analyzed by SPSS 26.0 (SPSS Inc. Chicago, IL, USA) using one-way ANOVA and Duncan’s multiple tests to compare the significant differences. The “corrplot” package in R (R Core Team, 2014) was used to obtain the Pearson correlation coefficient and significance. Differential gut microbes and milk metabolites were verified by ANOVA and Benjamini–Hochberg FDR adjustment in STAMP (version 2.1.3). The contribution of maternal factors to the gut microbiota in offspring was calculated by variance partitioning analysis (VPA) using the “varpart” package of R.
Results
MFD improved the performance of sows and piglets
Swine (Sus scrofa), as an animal biomedical model, have been extensively used to study the gut microbiota, its host interactions, and the consequences for gut health because their gut is physiologically and structurally similar to that of humans and because their diets are easily manipulated. The nutritional value and microbe content of the experimental MFD are presented in Tables S1 and S3. The levels of crude protein, small peptides, lactic acid and probiotics significantly increased, after fermentation, while the levels of NDF, ADF and amylose markedly decreased (P < 0.05). Enterococcus, Bacillus and Pseudomonas were the three most enriched genera in the MFD. The impact of the MFD on the performance of sows and piglets was specifically examined. As shown in Table S4, the MFD significantly improved the average daily feed intake (P = 0.01) and milk yield (P = 0.03) in sows. Piglets in the MFD group showed a significant increase in weaning weight gain (P = 0.04) and a decrease in diarrhea incidence (P = 0.00). Probiotic treatment tended to increase the average daily feed intake and milk yield in sows, as well as piglet weaning weight gain, and notably decreased the incidence of diarrhea (P = 0.03).
MFD changed the composition of the sow gut microbiota
Regarding the maternal aspects, the effects of the MFD on the gut microbiota in sows were investigated. Compared with the CON group, the α-diversity of the maternal gut microbiota in the MFD group on day 28 had a trend-level increase, whereas it was significantly increased in the PROB group (P = 0.04) (). Principal coordinates analysis (PCoA) analysis showed that the β-diversity of the maternal gut microbiota on day 28 was significantly different among the groups (, P = 0.001). A heatmap of the most differentially abundant genera revealed that the abundance of gut Lactobacillus and Succiniclasticum significantly increased in the MFD group on day 28, whereas the abundance of Mitsuokella and Erysipelotrichaceae increased in the PROB group (, LDA > 3.0). The MFD enhanced the functions of sow gut microbiota related to the metabolism of carbohydrates, proteins, and fats (Fig. S1a). To explain the impacts of MFD on the gut microbiota of sows, we analyzed the correlations of the significantly different nutritional and microbial content of the MFD with the sow gut microbiota (). The results indicated that small peptides and the pH of the MFD significantly induced the abundance of sow gut Lactobacillus and Succiniclasticum (P < 0.05).
Figure 2. The effects of MFD on the gut microbiota and milk metabolome of sows (n = 6). (a) Chao1 index dilution curve of α-diversity. (b) Principal coordinates analysis (PCoA) boxplot of β-diversity based on the Unweighted unifrac distance (ANOSIM analysis, P < 0.001). (c) Distribution of significantly different gut microbe genera in individual sows (LEfSe analysis, LDA > 3.0). (d) Correlations between the MFD and sow gut microbiota. (e) sPLS-DA plot of maternal milk metabolites (ANOSIM analysis, P < 0.001). (f) Distribution of differential milk metabolites in individual sows based on Pearson distance and average clustering (VIP > 1.0, P < 0.05). (g) The significantly different metabolite-related metabolic pathways of milk metabolites. ***: P < 0.001, **: 0.001 < P < 0.01, *: 0.01 < P < 0.05 indicate significance.
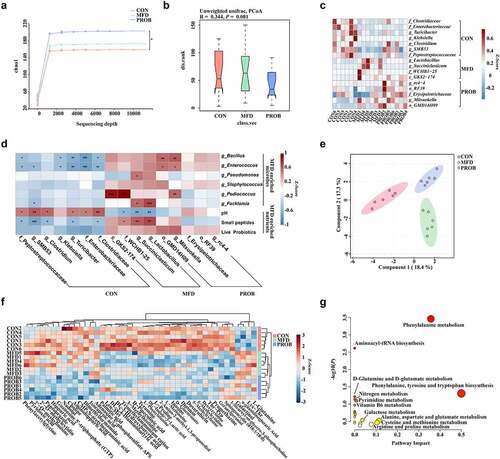
MFD changed the composition of the sow milk metabolome
MFD also changed the milk metabolome in sows. The sPLS-DA plot of cluster analysis showed that the clusters between the three groups were clearly separated (, P < 0.001). Among the 43 differential metabolites, MFD significantly increased the concentrations of α-guanidine glutaric acid, hippuric acid, L-glutamine (GLN), and pyridoxic acid in the milk but significantly reduced the concentrations of geranylgeraniol, 1-Stearoyl-sn-glycero-3-phosphocholine, deoxycytidine, trimethylammonium cation, and 5-methylcytosine (, variable importance projection (VIP) > 1.0, P < 0.05). The significantly different metabolites were taken to indicate the significantly different metabolic pathways reflected in the milk metabolome (, P < 0.05). Phenylalanine metabolism; phenylalanine, tyrosine and tryptophan biosynthesis; aminoacyl-RNA biosynthesis; D-glutamine and D-glutamate metabolism; and alanine, aspartic acid and glutamate metabolism were significantly different among the groups. Among them, D-glutamine and D-glutamate metabolism, aminoacyl-RNA biosynthesis, and phenylpropane metabolism were the top three significantly enriched metabolic pathways. The top 15 significantly different metabolites and metabolite-related metabolic pathways of sow’s milk are shown in Fig. S1b and c.
MFD improved the longitudinal and horizontal assembly of early-life gut microbiota in piglets
The early gut microbiota is essential for gut development and health. Therefore, the effects of the MFD on the longitudinal and horizontal assembly of the gut microbiota in piglets were investigated. The α-diversity of the gut microbiota of piglets continued to increase as aging during lactation (). There were no differences of the α and β-diversity of the gut microbiota on day 7 and 14, but there were significant differences on day 28 (, Fig. S2b, P < 0.05). At the phylum level, the MFD increased and then maintained the abundance of gut Firmicutes in piglets, whereas this taxon showed an increasing trend followed by a decreasing trend in other groups (). Interestingly, the maturation patterns of the gut microbiota, as indicated by the microbiota maturation index of each group, were different (). The piglet gut microbial development curve of the MFD was the fastest group, reaching a plateau as early as day 14 of lactation. To better understand how the MFD significantly affects interactions between microbes in the gut microbiota of piglets, the co-occurrence network of gut microbial interactions at different time points was analyzed. The abundance and correlations with other taxa (edge number) of Lactobacillus, Bifidobacterium, Prevotella and Oscillatoria were higher than those of other genera (Fig. S3). In addition, MFD significantly reduced the significance of LPS biosynthesis and bacterial toxins of piglet gut microbiota over time (Fig. S2b). The gut microbiota and functions of the three groups of piglets on day 28 were further analyzed. Compared to the other groups, the MFD increased the abundance of gut Lactobacillus in piglets (, LDA > 2.5) and metabolic processes such as those involving amino acid-related enzymes and glucose metabolism (Fig. S2c).
Figure 3. The effects of MFD on the longitudinal and horizontal assembly of early-life gut microbiota in piglets (n = 6). (a) Chao1 index of α-diversity. (b) PCoA plots of β-diversity based on the Bray–Curtis index (ANOSIM analysis, P < 0.001). (c) Area plots at the phylum on days 7, 14 and 28. (d) Maturation curve of gut microbiota. (e) Distribution of significantly different gut genera in offspring individuals on day 28 (LEfSe analysis, LDA>3.0).
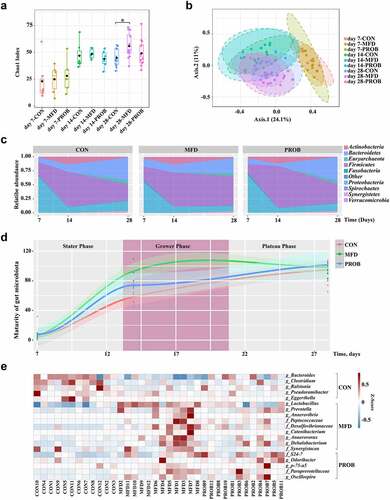
MFD reduced the susceptibility of piglets to LPS-induced colonic inflammation
Neonates are sensitive to pathogenic infection because their digestive system is not yet completely developed, making them prone to colonic inflammation. To assess the resistance of offspring to colonic inflammation, piglets were challenged with LPS. The results of hematoxylin and eosin staining and electron microscopy revealed that the MFD significantly alleviated the LPS-induced injury of the piglet colon, that is, the morphology of the microstructure (, P < 0.05). Similarly, the MFD restored the decreased protein levels of ZO-1, β-catenin, Occludin, and Claudin 1 in the piglet colon induced by LPS (, P < 0.05). In addition, a decrease in the colonic apoptotic index and cleaved caspase 3 protein expression, and an increase in Bcl2 protein expression in piglets of the MFD group were observed compared to the LPS group (, P < 0.05). In terms of colonic macrophage status, the results showed that the MFD alleviated the decrease in Arg1 and increase in iNOS caused by LPS (, P < 0.05). The MFD alleviated the LPS-induced increase in IL-1β and IFN-γ levels and the decrease in IL-10 and TGF-β levels in the colon (, P < 0.05). The overall mitigatory effects were greater in the MFD group than in the PROB group.
Figure 4. The impacts of an MFD on the susceptibility of piglets to LPS-induced colonic epithelial inflammation (n = 6). (a) Colonic histological morphology images and pathological scores. Scale bar of hematoxylin and eosin staining images: 200 μm; scale bar of scanning electron microscope (SEM) images: 1 μm; scale bar of transmission electron microscope (TEM) images: 0.2 μm. (b) Images of immunofluorescence of ZO-1 and β-catenin. (c) Colonic tight junction protein expression. (d) TUNEL image and statistical analysis. (e) Apoptosis-related protein expression in the colon. (f) M1 and M2 macrophage surface protein expression in colon. (g) Colonic cytokines. ***: P < 0.001, **: 0.001 < P < 0.01, *: 0.01 < P < 0.05 indicate significance.
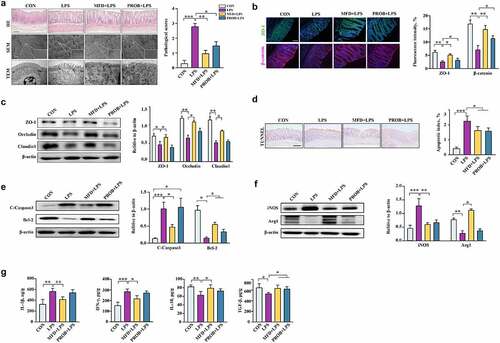
MFD programmed the early-life gut health of offspring by altering the maternal gut microbiota
To verify whether the MFD improves early-life gut health by regulating the maternal gut microbiota, FMT assay was performed. Fecal microbiota samples from sows of the CON, MFD, and PROB groups were transplanted to antibiotic-treated mouse dams for 4 weeks. The mouse dams and pups were intraperitoneally injected with LPS on the weaning day of pups. The β-diversity of the gut microbiota of mouse dams transplanted with sow fecal microbiota was significantly different after LPS treatment (, P = 0.049). The abundance of gut Lactobacillus and Clostridium in mouse dams in the MFD-trans group was remarkably enriched compared to that in the other groups, whereas the abundance of Streptococcus, Pantoea, and Flexispixa was enriched in the CON, LPS, and PROB groups, respectively (, LDA > 3.0). With regard to the morphology of the gut in mouse dams, maternal FMT from the MFD group alleviated LPS-induced colonic injury and the decrease in ZO-1, Occludin, and Claudin 1 protein levels (, P < 0.05). The results of mouse pups after LPS treatment were similar to those of dams. However, there was no difference in the weaning weight among the groups (Fig. S4a). An increasing tendency was found in the weaning weight gain of pups (Fig. S4a, P = 0.067). Maternal FMT markedly differentiated the β-diversity of the gut microbiota in mouse pups after LPS challenge (, P = 0.007). The abundance of gut Lactobacillus and Streptococcus in mouse pups of the MFD-trans group was notably enriched compared with that in the other groups after LPS treatment, whereas the abundance of Anaerobacillus, Sutterella, and Burkholderia was enriched in the CON-trans, CON-trans+LPS, and PROB-trans+LPS groups, respectively (, LAD > 2.5). The LPS-induced injury to the morphology of the colon, the increase in gut permeability, and the decline in ZO-1, Occludin and Claudin 1 protein levels in pups were reversed in the MFD transplanted group (; Fig. S4b, P < 0.05). The transplantation of fecal microbiota from the MFD mitigated the LPS-induced increase in serum DAO, DLA, colonic IL-6 and TNF-α and the decrease in colonic IL-10 of pups (Fig. S4c, P < 0.05). The overall colonic status of both mouse dams and pups in the MFD-trans group after LPS challenge was better than that of the PROB-trans group.
Figure 5. The effects of maternal FMT from MFD-fed sows on the susceptibility of mouse dams and pups to LPS-induced colonic epithelial inflammatory impairment (n = 6). (a) β-diversity of dam gut microbiota based on Bray–Curtis index (ANOSIM analysis, P = 0.049). (b) Distribution of differentially abundant genera in the gut of mouse dams (LEfSe analysis, LDA>3.0). (c) Colonic histological morphology images of dams and pathological scores. Scale bar of hematoxylin and eosin staining images: 200 μm; scale bar of scanning electron microscope (SEM) images: 1 μm; scale bar of transmission electron microscope (TEM) images: 0.2 μm. (d) Colonic tight junction protein expression of dams. (e) β-diversity of pup gut microbiota based on Bray–Curtis index (ANOSIM analysis, P = 0.007). (f) Distribution of differentially abundant genera in the gut of mouse pups (LEfSe analysis, LDA>2.5). (g) Colonic histological morphology images of pups and pathological scores. Scale bar of hematoxylin and eosin staining images: 200 μm; scale bar of scanning electron microscope (SEM) images: 1 μm; scale bar of transmission electron microscope (TEM) images: 0.2 μm. (h) Colonic tight junction protein expression of pups. ***: P < 0.001, **: 0.001 < P < 0.01, *: 0.01 < P < 0.05 indicate significance.
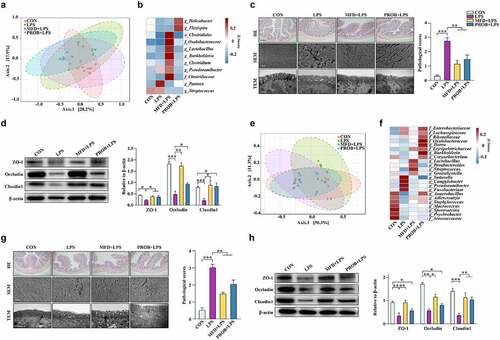
Sow’s gut Lactobacillus reuteri and milk L-GLN are two MFD-induced effectors that enhance gut health in piglets
Maternal gut microbiota and milk play critical roles in early-life gut health. The neonatal gut microbiota is essential in the maintenance of gut health. Therefore, correlation analysis was performed to investigate the effectors of sow’s gut microbiota and milk metabolites that affected the gut health of piglets. The results indicated that there was a significant positive correlation between gut Lactobacillus levels in sows and piglets (, P < 0.05). A significant positive correlation between GLN in the milk metabolite fraction and gut Lactobacillus in piglets was also identified (, P < 0.05). Next, the main maternal variables that shaped the gut microbiota of piglets were explored. The variation of piglets’ gut microbiota explained by the sow gut microbiota and milk metabolites were 0.86% and 1.36%, respectively (Fig. S5). The combined explanation of both sow gut microbiota and milk metabolites reached 93.52%, and the unexplainable factor accounted for 4.26%. The result suggested that the combined effects of sow gut microbiota and milk metabolites were the most important variables for gut microbiota assembly in piglets. Additionally, correlation heatmap analysis of piglet gut microbiota and piglet phenotypic indices showed that gut Lactobacillus was significantly positively correlated with piglet weaning weight gain, serum growth hormone, and TGF-β concentration and negatively correlated with the diarrhea rate, zonulin expression, and TNF-α concentration (, P < 0.05). In addition, the relative abundance of gut Lactobacillus in sows and piglets and the concentration of GLN in the milk metabolite fraction were enriched in the MFD group (, P < 0.05). The absolute concentration of milk GLN increased in the MFD group (, P < 0.05). The sequences of the most significantly different OTUs of gut Lactobacillus in sows and piglets were BLASTed against the NCBI database and found that Lactobacillus reuteri (LR) has a high score suggesting this is the most likely taxa associated with the OTU (Table S5, 6). In addition, the absolute quantification of LR in sow and piglet feces was enriched in the MFD group (, P < 0.05). Furthermore, the in vitro fermentation of maternal fecal microbiota from the CON group indicated that the MFD could enhance the proliferation of LR compared to the CON diet (, P < 0.05).
Figure 6. The sow’s effectors that contribute to piglets gut health (n = 6). (a) Correlations between sow gut microbiota and piglet gut microbiota based on Pearson coefficient. (b) Correlations between milk metabolites and piglet gut microbiota based on Pearson coefficient. (c) Correlations between piglet gut microbiota and piglet serum indexes based on Pearson coefficient. (d) The relative abundance of the most differentially abundant OTUs in both the sow and piglet gut microbiota and the concentration of GLN in the milk. (e) The absolute concentration of milk GLN. (f) The absolute quantity of fecal LR of sow and piglets. (g) The absolute quantity of LR in thesupernatant of the MFD in vitro fermentation system. ***: P < 0.001, **: 0.001 < P < 0.01, *: 0.01 < P < 0.05 indicate significance.
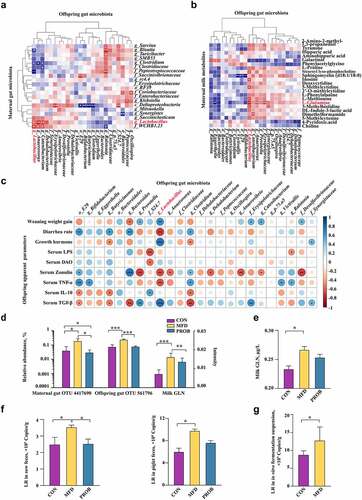
LR and GLN alleviated LPS-induced colonic inflammation by suppressing phosphorylation in the p38 MAPK/JNK pathway
The effects of LR and GLN on LPS-induced colonic inflammation were further examined in mice and cells. No difference was found in mouse weight gain among the groups (Fig. S6a). The β-diversity results showed that the structures of the gut microbiota in mice were clearly distinguishable among the groups (, P < 0.001). The combination of LR and GLN (MIX) alleviated the LPS-induced decrease in the abundance of Lactobacillus reuteri (, LDA > 3.0). MIX restored the morphology of the injured colon and increased the gut permeability caused by LPS (, Fig. S6b, P < 0.05). MIX treatment alleviated the LPS-induced decrease in ZO-1, Occludin, and Claudin 1 protein levels (, P < 0.05). MIX restored the LPS-induced increase in IL-6 and TNF-α concentrations and the decrease in IL-10 and TGF-β concentrations (, P < 0.05). The effects of MIX were better than those of either treatment alone.
Figure 7. Validation of the effects of LR and GLN on LPS-induced inflammatory injury in the colon of mice (n = 6). (a) β-diversity of gut microbiota based on Bray–Curtis index (ANOSIM analysis, P < 0.001). (b) Distribution of differentially abundant gut microbe genera in mice (LEfSe analysis, LDA>3.0). (c) Colonic histological morphology images and pathological scores. Scale bar of hematoxylin and eosin staining images: 200 μm; scale bar of scanning electron microscope (SEM) images: 1 μm; scale bar of transmission electron microscope (TEM) images: 0.2 μm. (d) Colonic tight junction protein expression. (e) Colonic cytokines. ***: P < 0.001, **: 0.001 < P < 0.01, *: 0.01 < P < 0.05 indicate significance.
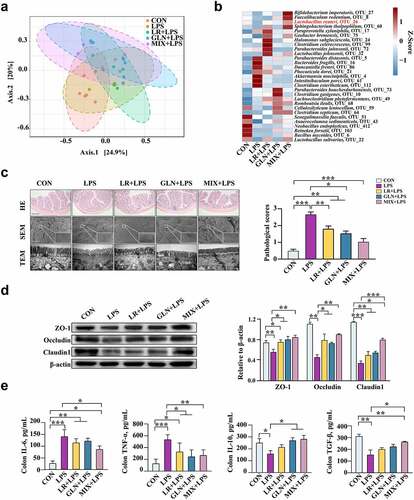
The optimal treatment concentrations of LR and GLN were investigated using Caco-2 and 3D4/2 cells in cell viability and cytotoxicity assays (Fig. S6c, d). LR and GLN treatment diminished the LPS-induced decrease in the survival rate of 3D4/2 cells (Fig. S6e, P < 0.05). Compared with the CON group, LPS treatment reduced the expression of Arg1 in 3D4/2 cells and increased the expression of iNOS. LR and GLN ameliorated this phenomenon (Fig. S6f, P < 0.05).
LR and GLN treatments alleviated the significant LPS-induced decrease in the relative TER values of Caco-2 cells, and the relative TER values of MIX and LPS treatment had the largest difference at 12 h (, P < 0.05). LR and GLN significantly inhibited the increase in FD4 content caused by LPS (, P < 0.05). Furthermore, LR and GLN alleviated the LPS-induced decrease in the viability of Caco-2 cells (Fig. S6g, P < 0.05). LR and GLN restored the LPS-induced decrease in ZO-1, Occludin, Claudin 1 and Bcl-2 expression and the increase in the expression of cleaved caspase 3 and Bax (, P < 0.05). Meanwhile, LR and GLN mitigated the LPS-induced increase in the phosphorylation of p38 MAPK and JNK proteins (, P < 0.05). Additionally, the activators of p38 (S2266), JNK (S7409), and ERK1/2 (S1013) were used to explore the effects of MIX on the LPS-induced expression of MAPK-related pathway proteins. The results showed that the three activators effectively altered cell viability and activated the respective proteins of the MAPK pathway (Fig. S6h, i, P < 0.05). Remarkably, p38 MAPK and JNK activators significantly reduced the suppressive effects of MIX on the LPS-induced decrease in the cell survival rate and tight junction protein expression and the increase in proapoptotic protein expression, while the ERK1/2 activator had no effect ( P < 0.05). The effects of MIX on alleviating LPS-induced inflammation were better than those of LR and GLN alone.
Figure 8. Validation of the effects of LR and GLN on LPS-induced inflammatory injury in cells (n = 3). (a) Changes in the relative TER values of Caco-2 cells for the completely differentiated monolayer; (b) FD4 content in lower compartments of Transwell plates. (c) Tight junction and apoptosis-related protein expression in Caco-2 cells. (d) p38, JNK and ERK1/2 phosphorylation in Caco-2 cells. (e) The tight junction and apoptosis-related protein expression when Caco-2 cells were treated with specific activators of p38, JNK and ERK1/2. ***: P < 0.001, **: 0.001 < P < 0.01, *: 0.01 < P < 0.05 indicate significance.
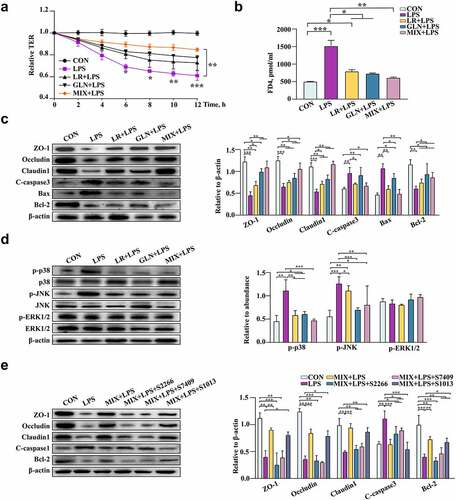
Discussion
The colonization of the gut by microbes in early life is essential for gut development and contributes to short- and long-term health outcomes.Citation28 Maternal nutritional states play fundamental roles in offspring gut health programming.Citation29 Fermented diets are functional foods that can positively regulate gut health.Citation30 However, there is little evidence to explain how the MFD manipulates gut development and health in offspring. Here, MFD-induced milk GLN and gut LR were found to contribute to early-life gut health by improving the establishment of early gut microbiota and attenuating LPS-induced gut inflammation by suppressing the phosphorylation of p38 MAPK and JNK and activation of Caspase-3.
Gut development in offspring is greatly affected by the maternal gut microbiota and breast milk.Citation31 There is little information on the effects of diet on the maternal gut microbiota, although it is important during pregnancy and lactation.Citation32 Roytio et al. reported that obese mothers who consumed sufficient amounts of fiber during pregnancy showed low-grade inflammation, a reduced abundance of gut Bacteroidetes, and a higher degree of gut microbial richness.Citation33 Mandal et al. found that low maternal intake of fat-soluble vitamins was related to increased gut microbial diversity and reduced abundance of Proteobacteria.Citation34 Only one study indicated that the gut microbial community in lactating women was related to macronutrient and micronutrient intake.Citation35 In the present study, the MFD reduced the abundance of maternal gut Enterobacteriaceae and Klebsiella. The low pH and probiotics in the MFD may have prevented pathogenic infection and enhanced gut health. The MFD significantly promoted the abundance of gut Lactobacillus and Succiniclasticum. Lactobacillus has been widely studied because of its ability to enhance gut barrier function, balance the gut microbiota, modulate the innate immune system, and prevent the colonization of pathogenic bacteria, thereby benefiting host health.Citation36 Succiniclasticum can utilize succinate and produce propionate, thus providing benefits to gut health.Citation37 The results suggest that MFD not only inhibits pathogens but also plays an important role in the modulation of gut commensal microbiota. The results of the analysis of the milk metabolome indicated that several amino acids and organic acids were significantly upregulated by MFD. Additionally, D-glutamine and D-glutamate metabolism in the milk metabolome was enhanced. Milk metabolites can promote microbial-dependent growth in infants.Citation38 Wu et al. reported that breast milk from pathological mothers could impair the growth of newborns.Citation32 Thus, the impact of the MFD on gut health and growth performance in offspring was attributed to the manipulation of maternal nutrition as a consequence of the comprehensive effects of the vertical transmission of microbes and milk metabolites. Notably, the correlation analysis revealed that small peptides and the pH of the MFD dramatically shaped the gut microbiota of sows.
Studies have indicated that maternal diets during pregnancy can affect gut health and the risk of pathogen infection in offspring in early and late life.Citation39–42 In this study, the effects of the MFD on the longitudinal and horizontal assembly of the gut microbiota in offspring during lactation were investigated. The MFD significantly improved the diversity and abundance of gut Firmicutes in offspring on day 28. The results revealed that the effects of the MFD on the development of the gut microbiota depended on time. Interestingly, the MFD accelerated the maturation of the gut microbiota in offspring. Additionally, the microbiota on day 14 had the highest number of correlations and level of abundance, indicating that they may have undergone remarkable changes and play an important role in the development of the gut microbiota in offspring.Citation41 Furthermore, the number of gut microbial interaction edges decreased as the offspring aged, demonstrating that the gut microbiota shifted from the initial significant changes to a relatively steady state during lactation. The structural and functional dynamics of the gut microbiome in offspring can be used as an indicator of growth and health.Citation43 A mature gut microbiome in suckling offspring is beneficial to host growth and development and prepares animals for weaning.Citation26 Therefore, the MFD may improve gut development and health in offspring by promoting the maturation of the gut microbiome. Horizontally, the MFD increased the abundance of gut Lactobacillus and Prevotella as well as amino acid–related enzyme function and glucose metabolism in piglets on day 28. One human study investigated the associations between maternal diet and gut microbiota in breastfeeding infants.Citation44 Babakobi et al. found no correlations between maternal diet and the gut microbiome in offspring due to the difficult specific assessment of maternal dietary consumption during lactation. Two studies reported correlations between maternal fiber consumption and the gut microbiome during pregnancy and lactation in pigs. A decreased abundance of gut Enterococcus and an increased abundance of Clostridiaceae were found in piglets from sows fed a fiber-rich diet.Citation45,Citation46 The different results may be attributed to the differences in diet type and intake volume, with proper supplementation volumes having the potential to enhance the effects of the maternal diets. In addition, the fermenting strains used to produce the MFD could have affected the results.Citation47 To take advantage of their combined probiotic properties, Bacillus subtilis and Enterococcus faecalis were used as inoculating microbes in the present study, which not only improved the nutritional value of the MFD but also promoted the development of the gut microbiota in offspring by maternal intervention.
Piglets were challenged with LPS to examine the effects of the MFD on the resistance of offspring to colonic inflammation. MFD significantly diminished LPS-induced morphological injury to colonic epithelial cells. The gut epithelial barrier consists of epithelial cells and intercellular junction proteins that play important roles in preventing inflammation and infection.Citation48 An increase in the abundance of beneficial microbes in the gut can improve the integrity of the intestinal barrier.Citation49 Cheng et al. Citation50 transplanted the fecal microbiota of healthy Jinhua pigs into K88-infected piglets, which were found to have improved intestinal morphology, reduced intestinal permeability and enhanced expression of mucin and mucosal tight junction proteins as a result of the transplant. The MFD improved the establishment of the gut microbiota in piglets and increased the abundance of Lactobacillus. These results demonstrated that the MFD benefited the gut microbiota in offspring to mitigate the LPS-induced impairment of colonic physical barriers. Cytokines are involved in the regulation of gut homeostasis and immune function.Citation51 In the present study, the MFD restored the cytokine disorder in offspring. Our previous study reported that the serum levels of anti-inflammatory cytokines in sows were enhanced by the MFD.Citation52 Maternal immune factors can be transmitted vertically to offspring.Citation28,Citation53 Liu et al.Citation54 demonstrated that the concentration of IL-10 in maternal serum and feces increased, those of IL-6 and TNF-α decreased, and that of IL-6 in serum of offspring also decreased by adding different dietary supplements to feed sows. A study has also shown that the suppression of colitis was mainly due to the decrease in inflammatory cytokine levels and the increase in anti-inflammatory cytokine levels.Citation55 Thus, MFD may ameliorate LPS-induced colonic cytokine disturbances in offspring by improving the immune status of sows. Macrophages comprise proinflammatory macrophages (M1 type) and anti-inflammatory macrophages (M2 type), which play vital roles in the inflammatory response.Citation56 MFD significantly alleviated LPS-induced proinflammatory colonic macrophages in offspring, which may be one of the anti-inflammatory mechanisms. Apoptosis is a type of programmed cell death that involves the activation of caspases, which is often accompanied by impairment of the gut barrier.Citation57 Therefore, the MFD can diminish apoptosis in the colon of LPS-challenged piglets and promote epithelial barrier function.
FMT is a critical technique for research into the functions of gut microbiota.Citation58 To further investigate whether the MFD affected early-life gut health by regulating the maternal gut microbiota, sow fecal microbiota was transplanted to broad-spectrum antibiotic-treated mouse dams. FMT from the MFD notably alleviated the LPS-induced decrease in gut Lactobacillus in both mouse dams and pups. Accordingly, FMT from the MFD significantly mitigated LPS-induced colonic inflammation in mouse dams and pups. Inconsistent with the results of the piglets, there was no significant difference in the body weight of pups and an increasing trend in weight gain (P = 0.067). The difference in the body weight of the offspring occurred during approximately 8 weeks of maternal prebiotic supplementation in mice.Citation59 Thus, the lack of an obvious body weight increase in pups in the present study might be due to insufficient maternal treatment time. Additionally, the different gene types and physiologies between mice and pigs may have caused inconsistencies in the results. Overall, these results are consistent with those of pigs, verifying that the MFD might improve the gut health of offspring by positively modulating the maternal gut microbiota.
Notably, the effects of the MFD were better than those of the PROB in both the maternal and offspring results. This might have been attributed to the probiotics in the MFD, which could positively shape the gut microbiota. At the same time, the MFD contains bioactive compounds, such as hydrolyzed small peptides, which could enhance physical barrier function, activate host immune cells, and improve gut functions. In addition, microbial metabolites in the MFD, such as organic acids, enzymes, and antimicrobial peptides, also exist and exert their properties.Citation12
To identify the main maternal components that affect gut health in offspring, correlation analysis and absolute quantification were conducted. The results of correlation analysis indicated that maternal gut LR and milk GLN promoted the abundance of offspring gut LR, thereby improving gut health and animal growth. In addition, the relative and absolute abundance of LR and milk GLN were significantly enriched in the MFD group, indicating that MFD might increase the early-life abundance of LR in the gut by improving maternal gut LR and milk GLN. Furthermore, the in vitro fermentation assay with maternal fecal microbiota revealed that the MFD promoted LR proliferation. Therefore, LR and GLN were two of the main maternal effectors that affected gut health in offspring. Additionally, the explanatory analysis indicated that the main contribution to the gut microbiota in offspring was the combined effects of the maternal gut microbiota and milk. Our previous study reported that the content of glutamate increased after diet fermentation.Citation60 Thus, the enriched glutamate in the MFD and enhanced milk glutamine metabolism might have contributed to the improved milk GLN. Additionally, the MFD could be easily digested, providing probiotics and their metabolites that might increase the amount of GLN in milk. The underlying mechanism of the increase in the abundance of the offspring gut LR might have been attributed to the transfer of the MFD-improved maternal gut LR through the entero-mammary axis.Citation7 LR and GLN alleviated LPS-induced gut inflammatory disorders by inhibiting p38 MAPK/JNK phosphorylation. The MAPK pathway plays crucial roles in cell physiology and the immune response.Citation61 MFD mitigated the LPS-induced increased phosphorylation of p38 MAPK and JNK in the colon during early life, which might have contributed to the reduced colonic inflammation. Ulcerative colitis can be ameliorated by inhibiting MAPK activation and mediating gut barrier integrity.Citation62 Therefore, it is speculated that MFD might have alleviated the LPS-induced increase in the phosphorylation of p38 MAPK and JNK, thus reducing colonic inflammation in offspring. LR can secrete reuterin and lactic acid to improve gut health and enhance immunity.Citation63 Although GLN is not generally considered an essential nutrient, it can improve gut mucosa and barrier functions and affect the expression of amino acid receptors and transporters as well as the immune function of the gut.Citation64 Therefore, the beneficial effects of gut LR and milk GLN on LPS-induced inflammation in vivo and in vitro were further revealed. Interestingly, LR and GLN together had the best effect, further implying that the combination of maternal milk and gut microbiota plays a major role in regulating the gut health of offspring.
Conclusions
The results revealed that an MFD benefited the performance of sows and piglets, the maternal gut microbiota, and the metabolite content of the milk from the sows. Meanwhile, the MFD accelerated the maturation of the neonatal gut microbiota, promoted the abundance of gut LR, and enhanced microbial metabolic functions. In addition, the MFD regulated the neonatal gut microbiota and epithelial homeostasis through the maternal gut microbiota. Milk GLN and gut LR were two MFD-caused maternal effectors that regulated gut microbiota development and alleviating LPS-induced colonic inflammation in offspring by suppressing the phosphorylation of p38 MAPK and JNK and activation of Caspase-3. These results expand our knowledge of preemptive strategies for neonatal intestinal inflammation that target both maternal factors and the early-life gut microbiota; furthermore, these results indicate that an MFD may be valuable in regulating gut health in offspring.
Abbreviations
MFD: Maternal fermented diet
LR: Lactobacillus reuteri
GLN: L-glutamine
LPS: Lipopolysaccharide
MAPK: Mitogen-activated protein kinase
JNK: c-Jun N-terminal kinases
ERK: Extracellular signal regulated kinase
FMT: Faecal microbiota transplantation
PROB: Probiotics
TUNEL: Terminal deoxynucleotidyl transferase-mediated dUTP nick end labeling
PCoA: Principal co-ordinates analysis
SEM: Scanning electron microscope
SPF: Specific pathogen-free
TEM: Transmission electron microscopy
PBS: Phosphate buffer saline
Authors’ contributions
CW and YZW designed the experiments. CW and SYW conducted the experiments. CW, SYW and BJL collected the samples and performed the analysis of samples. CW, SYW and FQW analyzed the data. CW and SYW wrote the manuscript. ZQL, MLJ and YZW help review the manuscript. All authors have read and approved the final manuscript.
Ethics approval and consent to participate
The Institutional Animal Care and Use Committee of Zhejiang University approved all experiments involving animals.
Data availability
The datasets supporting the conclusions of this article are available in the NCBI Sequence Read Archive (SRA) repository under accession number PRJNA552,228, 765,737, 765,800 and 765,829.
Supplemental Material
Download MS Word (5.7 MB)Acknowledgments
We thank the staff at the Electronic Microscopy Center in Zhejiang University for their assistance with the TEM and SEM.
Disclosure statement
The authors declare that they have no competing interests.
Supplementary material
Supplemental data for this article can be accessed on the publisher’s website
Correction Statement
This article has been corrected with minor changes. These changes do not impact the academic content of the article.
Additional information
Funding
References
- Lu Q, Lin Y, Chen T, Lv H, Diao FY, Liu C, Peng M, Ling X, Li H, Wang Y, et al. Alterations of gut microbiota composition in neonates conceived by assisted reproductive technology and its relation to infant growth. Gut Microbes. 2020;12(1):1794466. doi:10.1080/19490976.2020.1794466.
- Dai X, Guo ZX, Chen DF, Li L, Song XL, Liu TY, Jin G, Li Y, Liu Y, Ajiguli A, et al. Maternal sucralose intake alters gut microbiota of offspring and exacerbates hepatic steatosis in adulthood. Gut Microbes. 2020;11(4):1043–23. doi:10.1080/19490976.2020.1738187.
- Stiemsma LT, Michels KB. The role of the microbiome in the developmental origins of health and disease. Pediatrics. 2018;141(4):e20172437. doi:10.1542/peds.2017-2437.
- Stinson LF. Establishment of the early-life microbiome: a DOHaD perspective. J Dev Orig Health Dis. 2020;11(3):201–210. doi:10.1017/S2040174419000588.
- Laforest-Lapointe I, Becker AB, Mandhane PJ, Turvey SE, Moraes TJ, Sears MR, Subbarao P, Sycuro LK, Azad MB, Arrieta M-C, et al. Maternal consumption of artificially sweetened beverages during pregnancy is associated with infant gut microbiota and metabolic modifications and increased infant body mass index. Gut Microbes. 2021;13(1):1–15. doi:10.1080/19490976.2020.1857513.
- Fehr K, Moossavi S, Sbihi H, Boutin RCT, Bode L, Robertson B, Yonemitsu C, Field CJ, Becker AB, Mandhane PJ, et al. Breastmilk feeding practices are associated with the co-occurrence of bacteria in mothers’ milk and the infant gut: the child cohort study. Cell Host Microbe. 2020;28(2):285–97e4. doi:10.1016/j.chom.2020.06.009.
- Sindi AS, Geddes DT, Wlodek ME, Muhlhausler BS, Payne MS, Stinson LF. Can we modulate the breastfed infant gut microbiota through maternal diet? FEMS Microbiol Rev. 2021;45(5). doi:10.1093/femsre/fuab011.
- Rodriguez JM. The origin of human milk bacteria: is there a bacterial entero-mammary pathway during late pregnancy and lactation? Adv Nutr. 2014;5(6):779–784. doi:10.3945/an.114.007229.
- García-Mantrana I, Selma-Royo M, González S, Parra-Llorca A, Martínez-Costa C, Collado MC. Distinct maternal microbiota clusters are associated with diet during pregnancy: impact on neonatal microbiota and infant growth during the first 18 months of life. Gut Microbes. 2020;11(4):962–978. doi:10.1080/19490976.2020.1730294.
- Kimura I, Miyamoto J, Ohue-Kitano R, Watanabe K, Yamada T, Onuki M, Aoki R, Isobe Y, Kashihara D, Inoue D, et al. Maternal gut microbiota in pregnancy influences offspring metabolic phenotype in mice. Science. 2020;367(6481):eaaw8429. 28;. doi:10.1126/science.aaw8429.
- David LA, Maurice CF, Carmody RN, Gootenberg DB, Button JE, Wolfe BE, Ling AV, Devlin AS, Varma Y, Fischbach MA, et al. Diet rapidly and reproducibly alters the human gut microbiome. Nature. 2014;505(7484):559–563. doi:10.1038/nature12820.
- Tamang JP, Cotter PD, Endo A, Han NS, Kort R, Liu SQ, Mayo B, Westerik N, Hutkins R. Fermented foods in a global age: east meets West. Compr Rev Food Sci Food Saf. 2020;19(1):184–217. doi:10.1111/1541-4337.12520.
- Díaz-López A, Bulló M, Martínez-González MA, Corella D, Estruch R, Fitó M, Gómez-Gracia E, Fiol M, García de la Corte FJ, Ros E, et al. Dairy product consumption and risk of type 2 diabetes in an elderly Spanish Mediterranean population at high cardiovascular risk. Eur J Nutr. 2016;55(1):349–360. doi:10.1007/s00394-015-0855-8.
- Gille D, Schmid A, Walther B, Vergères G. Fermented food and non-communicable chronic diseases: a review. Nutrients. 2018;10(4):448. doi:10.3390/nu10040448.
- Takada T, Chinda D, Mikami T, Shimizu K, Oana K, Hayamizu S, Miyazawa K, Arai T, Katto M, Nagara Y, et al. Dynamic analysis of human small intestinal microbiota after an ingestion of fermented milk by small-intestinal fluid perfusion using an endoscopic retrograde bowel insertion technique. Gut Microbes. 2020;11(6):1662–1676. doi:10.1080/19490976.2020.1766942.
- Staudacher HM, Loughman A. Gut health: definitions and determinants. Lancet Gastroenterol Hepatol. 2021;6(4):269. doi:10.1016/S2468-1253(21)00071-6.
- Feldsine P, Abeyta C, Andrews WH, Committee AIM. AOAC International methods committee guidelines for validation of qualitative and quantitative food microbiological official methods of analysis. J AOAC Int. 2021;85(5):1187–1200. doi:10.1093/jaoac/85.5.1187.
- NRC. Nutrient requirements of Swine 11th revis. Washington (DC): National Academic Press; 2012.
- Geng SJ, Cheng SS, Li Y, Wen ZS, Ma X, Jiang XM, Wang YZ, Han XY. Faecal microbiota transplantation reduces susceptibility to epithelial injury and modulates tryptophan metabolism of the microbial community in a piglet model. J Crohns Colitis. 2018;12(11):1359–1374. doi:10.1093/ecco-jcc/jjy103.
- Martino C, Morton JT, Marotz CA, Thompson LR, Tripathi A, Knight R, Zengler K. A novel sparse compositional technique reveals microbial perturbations. mSystems. 2019;4(1):e00016–19. doi:10.1128/mSystems.00016-19.
- Bokulich NA, Kaehler BD, Rideout JR, Dillon M, Bolyen E, Knight R, Huttley GA, Gregory Caporaso J. Optimizing taxonomic classification of marker-gene amplicon sequences with QIIME 2 ‘ s q2-feature-classifier plugin. Microbiome. 2018;6(1):90. doi:10.1186/s40168-018-0470-z.
- DeSantis TZ, Hugenholtz P, Larsen N, Rojas M, Brodie EL, Keller K, Huber T, Dalevi D, Hu P, Andersen GL. Greengenes, a chimera-checked 16S rRNA gene database and workbench compatible with ARB. Appl Environ Microbiol. 2006;72(7):5069–5072. doi:10.1128/AEM.03006-05.
- Wang Q, Garrity GM, Tiedje JM, Cole JR. Naive Bayesian classifier for rapid assignment of rRNA sequences into the new bacterial taxonomy. Appl Environ Microb. 2007;73(16):5261–5267. doi:10.1128/AEM.00062-07.
- Segata N, Izard J, Waldron L, Gevers D, Miropolsky L, Garrett WS, Huttenhower C. Metagenomic biomarker discovery and explanation. Genome Biol. 2011;12(6):R60. doi:10.1186/gb-2011-12-6-r60.
- Douglas GM, Maffei VJ, Zaneveld JR, Yurgel SN, Brown JR, Taylor CM, Huttenhower C, Langille MGI. PICRUSt2 for prediction of metagenome functions. Nat Biotechnol. 2020;38(6):685–688. doi:10.1038/s41587-020-0548-6.
- Gao PF, Ma C, Sun Z, Wang LF, Huang S, Su XQ, Xu J, Zhang H. Feed-additive probiotics accelerate yet antibiotics delay intestinal microbiota maturation in broiler chicken. Microbiome. 2017;5(1):91. doi:10.1186/s40168-017-0315-1.
- Sobanbua S, Dolkittikul S, Nakphaichit M, Keawsompong S, Nitisinprasert S. Antimicrobial peptide presenting potential strain-specific real time polymerase chain reaction assay for detecting the probiotic Lactobacillus reuteri KUB-AC5 in chicken intestine. Poult Sci. 2020;99(1):526–535. doi:10.3382/ps/pez549.
- Guo JL, Ren CL, Han X, Huang WD, You YL, Zhan JC. Role of IgA in the early-life establishment of the gut microbiota and immunity: implications for constructing a healthy start. Gut Microbes. 2021;13:1–21.
- Chen CY, Chen CK, Chen YY, Fang A, Shaw GT, Hung CM, Wang D. Maternal gut microbes shape the early-life assembly of gut microbiota in passerine chicks via nests. Microbiome. 2020;8(1):129. doi:10.1186/s40168-020-00896-9.
- Sanlier N, Gokcen BB, Sezgin AC. Health benefits of fermented foods. Crit Rev Food Sci Nutr. 2019;59(3):506–527. doi:10.1080/10408398.2017.1383355.
- Yang B, Ding MF, Chen YQ, Han FZ, Yang CY, Zhao JX, Malard P, Stanton C, Ross RP, Zhang H, et al. Development of gut microbiota and bifidobacterial communities of neonates in the first 6 weeks and their inheritance from mother. Gut Microbes. 2021;13(1):1–13. doi:10.1080/19490976.2021.1908100.
- Wu Y, Yu JX, Liu XY, Wang WL, Chen Z, Qiao J, Liu X, Jin H, Li X, Wen L, et al. Gestational diabetes mellitus-associated changes in the breast milk metabolome alters the neonatal growth trajectory. Clin Nutr. 2021;40(6):4043–4054.
- Roytio H, Mokkala K, Vahlberg T, Laitinen K. Dietary intake of fat and fibre according to reference values relates to higher gut microbiota richness in overweight pregnant women. Brit J Nutr. 2018;120(5):599–600. doi:10.1017/S0007114518001940.
- Mandal S, Godfrey KM, Mcdonald D, Treuren WV, Bjornholt JV, Midtvedt T, Moen B, Rudi K, Knight R, Brantsæter AL, et al. Fat and vitamin intakes during pregnancy have stronger relations with a pro-inflammatory maternal microbiota than does carbohydrate intake. Microbiome. 2016;4(1):55. doi:10.1186/s40168-016-0200-3.
- Carrothers JM, York MA, Brooker SL, Lackey KA, Williams JE, Shafii B, Price WJ, Settles ML, McGuire MA, McGuire MK, et al. Fecal microbial community structure is stable over time and related to variation in macronutrient and micronutrient intakes in lactating Women. J Nutr. 2015;145(10):2379–2388. doi:10.3945/jn.115.211110.
- Ghosh TS, Arnoux J, O’Toole PW. Metagenomic analysis reveals distinct patterns of gut lactobacillus prevalence, abundance, and geographical variation in health and disease. Gut Microbes. 2020;12(1):1–19. doi:10.1080/19490976.2020.1822729.
- Kumbhare SV, Kumar H, Chowdhury SP, Dhotre DP, Endo A, Matto J, Ouwehand AC, Rautava S, Joshi R, Patil NP, et al. A cross-sectional comparative study of gut bacterial community of Indian and Finnish children. Sci Rep. 2017;7(1):10555. doi:10.1038/s41598-017-11215-y.
- Rubio-Del-Campo A, Gozalbo-Rovira R, Moya-Gonzálvez EM, Alberola J, Rodríguez-Díaz J, Yebra MJ. Infant gut microbiota modulation by human milk disaccharides in humanized microbiome mice. Gut Microbes. 2021;13(1):1–20. doi:10.1080/19490976.2021.1914377.
- Aaltonen J, Ojala T, Laitinen K, Poussa T, Ozanne S, Isolauri E. Impact of maternal diet during pregnancy and breastfeeding on infant metabolic programming: a prospective randomized controlled study. Eur J Clin Nutr. 2011;65:10–19.
- Ashino NG, Saito KN, Souza FD, Nakutz FS, Roman EA, Velloso LA, Torsoni AS, Torsoni MA. Maternal high-fat feeding through pregnancy and lactation predisposes mouse offspring to molecular insulin resistance and fatty liver. J Nutr Biochem. 2012;23(4):341–348. doi:10.1016/j.jnutbio.2010.12.011.
- Grech A, Collins CE, Holmes A, Lal R, Duncanson K, Taylor R, Gordon A. Maternal exposures and the infant gut microbiome: a systematic review with meta-analysis. Gut Microbes. 2021;13:1–30.
- Vogt MC, Paeger L, Hess S, Steculorum SM, Awazawa M, Hampel B, Neupert S, Nicholls H, Mauer J, Hausen A, et al. Neonatal insulin action impairs hypothalamic neurocircuit formation in response to maternal high-fat feeding. Cell. 2014;156(3):495–509. doi:10.1016/j.cell.2014.01.008.
- Alderete TL, Jones RB, Shaffer JP, Holzhausen EA, Patterson WB, Kazemian E, Chatzi L, Knight R, Plows JF, Berger PK, et al. Early life gut microbiota is associated with rapid infant growth in Hispanics from Southern California. Gut Microbes. 2021;13(1):1961203. doi:10.1080/19490976.2021.1961203.
- Babakobi MD, Reshef L, Gihaz S, Belgorodsky B, Fishman A, Bujanover Y, Gophna U. Effect of maternal diet and milk lipid composition on the infant gut and maternal milk microbiomes. Nutrients. 2020;12(9):2539. doi:10.3390/nu12092539.
- Leblois J, Massart S, Li B, Wavreille J, Bindelle J, Everaert N. Modulation of piglets’ microbiota: differential effects by a high wheat bran maternal diet during gestation and lactation. Sci Rep. 2017;7(1):7426. doi:10.1038/s41598-017-07228-2.
- Passlack N, Vahjen W, Zentek J. Dietary inulin affects the intestinal microbiota in sows and their suckling piglets. BMC Vet Res. 2015;11(1):51. doi:10.1186/s12917-015-0351-7.
- Marco ML, Sanders ME, Ganzle M, Arrieta MC, Cotter PD, De Vuyst L, Hill C, Holzapfel W, Lebeer S, Merenstein D, et al. The international scientific association for probiotics and prebiotics (ISAPP) consensus statement on fermented foods. Nat Rev Gastroenterol Hepatol. 2021;18(3):196–208. doi:10.1038/s41575-020-00390-5.
- Peng M, Tabashsum Z, Patel P, Bernhardt C, Biswas C, Meng J, Biswas D. Prevention of enteric bacterial infections and modulation of gut microbiota with conjugated linoleic acids producing Lactobacillus in mice. Gut Microbes. 2020;11(3):433–452. doi:10.1080/19490976.2019.1638724.
- Zhang JC, Zhao JS, Jin H, Lv R, Shi HW, De GZ, Yang B, Sun Z, Zhang H. Probiotics maintain the intestinal microbiome homeostasis of the sailors during a long sea voyage. Gut Microbes. 2020;11(4):930–943. doi:10.1080/19490976.2020.1722054.
- Cheng SS, Ma X, Geng SJ, Jiang XM, Li Y, Hu LS, Li J, Wang Y, Han X. Fecal microbiota transplantation beneficially regulates intestinal mucosal autophagy and alleviates gut barrier injury. mSystems. 2018;3(5):e00137–18. doi:10.1128/mSystems.00137-18.
- Rabe H, Lundell A-C, Sjöberg F, Ljung A, Strömbeck A, Gio-Batta M, Maglio C, Nordström I, Andersson K, Nookaew I, et al. Neonatal gut colonization by Bifidobacterium is associated with higher childhood cytokine responses. Gut Microbes. 2020;12(1):1–14. doi:10.1080/19490976.2020.1847628.
- Wang C, Wei SY, Xu BC, Hao LH, Su WF, Jin ML, Wang YZ. Bacillus subtilis and Enterococcus faecium co-fermented feed regulates lactating sow’s performance, immune status and gut microbiota. Microb Biotechnol. 2021;14(2):614–627. doi:10.1111/1751-7915.13672.
- Ramanan D, Sefik E, Galvan-Pena S, Wu M, Yang L, Yang Z, Kostic A, Golovkina TV, Kasper DL, Mathis D, et al. An immunologic mode of multigenerational transmission governs a gut Treg setpoint. Cell. 2020;181(6):1276–90 e13. doi:10.1016/j.cell.2020.04.030.
- Liu BS, Zhu XY, Cui YL, Wang WJ, Liu H, Li ZD, Guo Z, Ma S, Li D, Wang C, et al. Consumption of dietary fiber from different sources during pregnancy alters sow gut microbiota and improves performance and reduces inflammation in sows and piglets. mSystems. 2021;6(1):e00591–20. doi:10.1128/mSystems.00591-20.
- Neurath MF. Cytokines in inflammatory bowel disease. Nat Rev Immunol. 2014;14(5):329–342. doi:10.1038/nri3661.
- Liu C, Huang SM, Wu ZH, Li TT, Li N, Zhang B, Han D, Wang S, Zhao J, Wang J, et al. Cohousing-mediated microbiota transfer from milk bioactive components-dosed mice ameliorate colitis by remodeling colonic mucus barrier and lamina propria macrophages. Gut Microbes. 2021;13(1):1–23. doi:10.1080/19490976.2021.1903826.
- Rao Y, Kuang ZQ, Li C, Guo SY, Xu YH, Zhao DD, Hu Y, Song B, Jiang Z, Ge Z, et al. Gut Akkermansia muciniphila ameliorates metabolic dysfunction-associated fatty liver disease by regulating the metabolism of L-aspartate via gut-liver axis. Gut Microbes. 2021;13(1):1–19. doi:10.1080/19490976.2021.1927633.
- Singh P, Alm EJ, Kelley JM, Cheng V, Smith M, Kassam Z, Nee J, Iturrino J, Lembo A. Effect of antibiotic pretreatment on bacterial engraftment after Fecal Microbiota Transplant (FMT) in IBS-D. Gut Microbes. 2022;14(1):2020067. doi:10.1080/19490976.2021.2020067.
- Paul HA, Collins KH, Nicolucci AC, Urbanski SJ, Hart DA, Vogel HJ, Reimer RA. Maternal prebiotic supplementation reduces fatty liver development in offspring through altered microbial and metabolomic profiles in rats. FASEB J. 2019;33(4):5153–5167. doi:10.1096/fj.201801551R.
- Wang C, Shi CY, Su WF, Jin ML, Xu BC, Hao LH, Zhang Y, Lu Z, Wang F, Wang Y, et al. Dynamics of the physicochemical characteristics, microbiota, and metabolic functions of soybean meal and corn mixed substrates during two-stage solid-State fermentation. mSystems. 2020;5(1):00501–19. doi:10.1128/mSystems.00501-19.
- Holani R, Babbar A, Blyth GAD, Lopes F, Jijon H, McKay DM, Hollenberg MD, Cobo ER. Cathelicidin-mediated lipopolysaccharide signaling via intracellular TLR4 in colonic epithelial cells evokes CXCL8 production. Gut Microbes. 2020;12(1):1785802. doi:10.1080/19490976.2020.1785802.
- Deng XC, Wang YD, Tian L, Yang ML, He SY, Liu YP, Jianxin Cao JX, Cheng GG. Anneslea fragrans wall. ameliorates ulcerative colitis via inhibiting NF-kappaB and MAPK activation and mediating intestinal barrier integrity. J Ethnopharmacol. 2021;278:114304. doi:10.1016/j.jep.2021.114304.
- Wu HQ, Xie S, Miao JF, Li YC, Wang ZH, Wang MJ, Yu Q. Lactobacillus reuteri maintains intestinal epithelial regeneration and repairs damaged intestinal mucosa. Gut Microbes. 2020;11(4):997–1014. doi:10.1080/19490976.2020.1734423.
- Hemarajata P, Versalovic J. Effects of probiotics on gut microbiota: mechanisms of intestinal immunomodulation and neuromodulation. Therap Adv Gastroenterol. 2013;6(1):39–51. doi:10.1177/1756283X12459294.