Abstract
Hydrogels are hydrophilic polymers with high flexibility and elasticity, and are widely used in the field of bone tissue engineering. However, their low toughness and insufficient mechanical strength have limited their applications. Nanocomposite hydrogels are flexible and porous as well as possess excellent biocompatibility and mechanical strength, thus exhibiting a significant potential to be used in the field of bone tissue engineering. Nanocomposite hydrogels are synthesized by chemically or physically crosslinking nanomaterials and hydrogels, and their physical, chemical, and biological properties can be enhanced by changing the properties and compositions of the nanomaterials. Both nanomaterials and hydrogel polymers can be customized, and therefore, nanocomposite hydrogels can be developed for bone repair under different conditions. In this review, we first introduce the synthesis methods and properties of nanocomposite hydrogels, and then summarize the applications and progress of nanocomposite hydrogels in bone tissue engineering.
Graphical Abstract
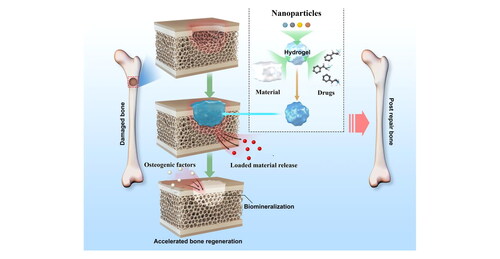
Nanocomposite hydrogels are used in bone tissue engineering. Adapted from Huang et al. (2019) [Citation121]
1. Introduction
Hydrogels, crosslinked three-dimensional polymers composed mainly of water, are widely used in bone tissue engineering [Citation1]. Hydrogels can mimic natural tissues, providing a suitable microenvironment for cells [Citation2–4]. They also possess good biocompatibility, and hence, they are excellent for bone tissue engineering applications [Citation5, Citation6]. However, the properties of conventional hydrogels are dependent on the polymer content and crosslink density, which significantly limit their mechanical strength and internal structure [Citation7–10]. To overcome these limitations, nanomaterials are used for the modification of hydrogels, and the resulting nanocomposite hydrogels exhibit improved mechanical strength and biological properties [Citation7, Citation11]. For example, Julien E. Gautrot et al. used electrospun PLA fibers and carboxymethyl cellulose (CMC) to prepare nanocomposite hydrogels, and its storage modulus increased by 3 times compared with pure hydrogels [Citation12]. Irmina Samba et al. prepared nanocomposite hydrogels containing PLGA nanoparticles with a more uniform pore structure than pure hydrogels [Citation13]. Nanomaterials and hydrogels can be crosslinked physically or chemically to form nanocomposite hydrogels, and different synthesis and combination methods (natural polymers/nanomaterials) can be used to alter the properties of the final composite hydrogels [Citation14]. In this review, we first introduce the synthesis methods for nanocomposite hydrogels and their properties, and then summarise the applications and progress of nanocomposite hydrogels in bone tissue engineering.
2. Synthesis of nanocomposite hydrogels and their properties
The main components of nanocomposite hydrogels are nanomaterials and hydrogels. In bone tissue engineering, inorganic nanomaterials such as nanometals (e.g. gold, copper, titanium), nanometallic oxides (e.g. TiO2, Fe3O4), and nano-nonmetals (e.g. BC, Se, Si) are used as nanomaterials, and hydrogels are usually formulated from natural polymers (e.g. gelatin, chitosan, cellulose, hyaluronic acid) or synthetic polymers (e.g. PEG, PEO, PNIPA) [Citation15–18].
The main synthesis methods for nanocomposite hydrogels are chemical (covalent bonding) and physical (non-covalent bonding) crosslinking [Citation19, Citation20]. In the chemical method, organic crosslinking agents are used to achieve irreversible covalent bonding between nanomaterials and polymers, whereas the physical method involves reversible bonding through hydrogen bonding and ionic interactions [Citation13, Citation21]. Nanocomposite hydrogels formed via physical crosslinking can be decomposed by changing physical conditions such as pH and temperature, whereas those formed via chemical crosslinking have better mechanical properties, can withstand greater mechanical stress, and are difficult to decompose [Citation22–25]. In addition, nanomaterials can be used as crosslinkers instead of organic crosslinkers to crosslink hydrogels as polymers, and the resulting products have greater mechanical toughness [Citation11, Citation26].
Nanocomposite hydrogels are generally synthesised from inorganic nanomaterials with a variety of properties and synthetic/natural polymers [Citation7]. The properties of the resulting nanocomposite hydrogels are dependent on the composition of the nanomaterials and polymers, the proportional concentration of nanomaterials and polymers, and the size and degree of dispersion of the nanomaterials in the hydrogel [Citation11]. Numerous studies have shown that as long as a small number of nanomaterials (2%∼10%) is added, the properties of the prepared nanocomposite hydrogels can be significantly improved [Citation27–30]. The combination of nanomaterials and hydrogel polymers directly affects the properties of nanocomposite hydrogels. Therefore, controlling the dispersion degree of nanomaterials in the hydrogel and its mutual combination with the molecular chains of the hydrogel is the key to the preparation of nanocomposite hydrogels. According to the dispersion method of nanomaterials in hydrogels, the current preparation methods include the blending method, grafting method, in situ precipitation method and freeze-thaw method [Citation14, Citation31].
2.1. The blending method
The blending method is the simplest method for preparing nanocomposite hydrogels, which directly mixes polymer monomers, crosslinking agents and nanomaterials, and initiates polymerization to form nanocomposite hydrogels [Citation32]. Filippi et al prepared magnetic responsive nanocomposite hydrogels from iron oxide nanoparticles (average particle size 15 nm) and polyethylene glycol (PEG) hydrogel polymers by co-blending, which have good biocompatibility and high elastic modulus, while it acts as a promoter of osteogenesis and angiogenesis in response to external magnetic field stimulation [Citation33]. The preparation process of the blending method is simple, and the nanocomposite hydrogel with uniform particle size can be obtained by controlling the stirring speed, material concentration and preparation period during mixing [Citation34]. However, when the nanocomposite hydrogel is prepared by the blending method, the nanoparticles are prone to agglomeration, which makes the structural enhancement effect of the prepared product not obvious. For example, Tong et al prepared nanocomposite hydrogels by blending carbon nanotubes and polyvinyl alcohol hydrogel polymers [Citation35]. The swelling properties of the composite hydrogels were significantly enhanced, but the mechanical properties were not significantly improved. The agglomeration phenomenon generated during the blending method weakens the direct interaction between the nanoparticles and the hydrogel polymer, resulting in the improvement of the mechanical properties of the prepared nanocomposite hydrogel is not obvious, which is a disadvantage that the blending method needs to overcome [Citation36, Citation37].
2.2. The Grafting-Onto method
The grafting method can effectively improve the agglomeration phenomenon of inorganic nanomaterials in hydrogel by modifying the surface of the nanomaterials to form a covalent bond between the inorganic nanomaterials and the hydrogel network [Citation21, Citation38]. At the same time, due to the enhanced interaction between nanoparticles and hydrogel polymers, the prepared nanocomposite hydrogel has stronger mechanical properties, and the surface of the nanomaterial is mainly modified by grafting method for polyacrylamide (PAM) hydrogels. Messing et al used methacrylic acid groups to modify the surface of magnetic nanoparticles, and then added the modified magnetic nanoparticles to the polyacrylamide hydrogel network to prepare nanocomposite hydrogels, which shows relatively high mechanical properties, including tensile strength and fracture toughness [Citation39]. Another similar study synthesized a nanocomposite hydrogel by grafting method, and its nano iron oxide particles were fused with 3-(trimethoxysilyl) propyl methacrylate and polydimethylsiloxane (PDMS) was used to modify the surface of the hydrogel [Citation40]. The mechanical properties of this nanocomposite hydrogel are greatly improved, and at the same time, it can maintain stability under fatigue load, which is very suitable for the application of bone tissue engineering [Citation41]. Therefore, the advantage of the grafting method is that after the surface of the nanomaterial is modified, the nanoparticles and the hydrogel polymer are combined in a covalent bond, which greatly improves the mechanical strength and stability of the nanocomposite hydrogel [Citation7, Citation42, Citation43].
2.3. In Situ precipitation
In situ precipitation is to uniformly disperse inorganic nanoparticles in hydrogel polymer monomers, and then polymerize the monomers under certain temperature conditions to form nanocomposite hydrogels [Citation44, Citation45]. This method can prepare nanocomposite hydrogels with good dispersion effect, can maintain the excellent properties of nanocomposite particles, and at the same time make the mutual bonding between nanoparticles and hydrogels more stable [Citation46, Citation47]. For example, Wang et al. synthesized magnetically responsive nanocomposite hydrogels by in situ precipitation. The main preparation process is to mix the magnetic nanoparticles and chitosan hydrogel, and then the mixture is polymerized in NaOH solution, in which chitosan and iron ions can chelate, this interaction makes the magnetic nanoparticles evenly dispersed in the hydrogel, and finally obtain a nanocomposite hydrogel with good magnetic response and mechanical properties [Citation28]. Meanwhile, the mechanical properties of nanocomposite hydrogels can be enhanced by increasing the concentration of magnetic nanoparticles (0 to 15 wt%). In addition, in situ precipitation can be triggered by appropriate heating, Yu et al. added an ultrasonically treated graphene aqueous suspension to an acrylamide monomer, and an in situ free radical polymerization reaction occurred under 25 °C water bath conditions, and a graphene nanocomposite hydrogel was formed after 48 h. In situ precipitation can ensure that the nanoparticles are evenly distributed in the hydrogel and maintain the characteristics of the nanoparticles, so the mechanical strength of the nanocomposite hydrogel can be prepared, the disadvantage is that the alkaline solution used may affect the ability of the hydrogel network cells to load, and the inorganic nanoparticles must be modified before the polymerization reaction [Citation36, Citation37, Citation48].
2.4. Freeze-thaw method
The freeze-thaw method is a method for preparing nanocomposite hydrogels by physical cross-linking. The method is mainly to repeatedly thaw the hydrogel mixed solution at low temperature and room temperature [Citation35, Citation49]. In this process, polymer aggregation and non-aggregation areas are formed internally when the hydrogel mixture solidifies, and when thawing, the polymer aggregation areas will undergo physical crosslinking to form a crosslinking network [Citation50]. Huang et al. prepared a magnetic nanocomposite hydrogel with good mechanical properties and biocompatibility by freeze-thaw method. During the preparation process, they mixed PVA, nano-hydroxyapatite (n-HA) and Fe2O3 in a certain proportion, mixed evenly at 60 °C, frozen at −20 °C for 16 h, thawed at room temperature for 4 h, and repeated freeze-thawed 7 times to finally obtain a nanocomposite hydrogel [Citation51]. Studies have reported that the number of freeze-thaws affects the mechanical strength and magnetization strength of magnetic nanocomposite hydrogels. The disadvantage of the freeze-thaw method is that the physical crosslinking is reversible, so the structure of the prepared hydrogel crosslinking network is not very strong, and it is easy to decompose quickly due to external influences.
3. Classification of nanocomposite hydrogels for bone tissue engineering applications
3.1. Clay-based nanocomposite hydrogel
Clay minerals are a class of inorganic layered nanomaterials consisting of two main units: tetrahedral (T) and octahedral (O) sheets [Citation42]. Nanoclay is a type of ultrathin (1 nm) polar nanomaterial [Citation52, Citation53] with excellent biocompatibility [Citation54], and its degradation products (e.g. magnesium and silicates) are non-toxic, resorbable, and capable of promoting cellular osteogenesis [Citation55]. The addition of nanoclay materials (dispersed phase) to the crosslinked network of the hydrogel (continuous phase) improves the mechanical properties and bioadhesion of the hydrogel, and the resulting nanocomposite hydrogel retains its previous inherent processing properties [Citation56]. These properties provide a theoretical basis for the application of clay-based nanocomposite hydrogels in bone tissue engineering () [Citation57].
Figure 1. Chemical structures of nanoclay platelets currently used for various biomedical engineering applications. Nanoclays are basically ultrathin (≈1 nm thick) platelets with a dual charge that makes it easy to dissolve and incorporate into almost any existing hydrogel matrix. Reproduced with permission [Citation56]. Copyright, John Wiley and Sons.
![Figure 1. Chemical structures of nanoclay platelets currently used for various biomedical engineering applications. Nanoclays are basically ultrathin (≈1 nm thick) platelets with a dual charge that makes it easy to dissolve and incorporate into almost any existing hydrogel matrix. Reproduced with permission [Citation56]. Copyright, John Wiley and Sons.](/cms/asset/23962121-5660-4277-bd31-412ffd257650/ynan_a_2076025_f0001_c.jpg)
According to the chemical composition and morphology of the nanoparticles, nanoclays can be divided into several categories, such as montmorillonite ((Al3.2Mg0.8) (Si8)O20(OH)4Na0.8), Laponite((Mg5.5Li0.3)Si8O20(OH)4Na0.7), hectorite((Mg5.2Li0.8)(Si8)O20(OH)4Na0.8), and halloysite (Si2Al2O5(OH)4·nH2O). The main nanoclay used in bone tissue engineering is Laponite, which consists of nanosheets with a high aspect ratio, width close to 25 nm, and thickness of 1–2 nm [Citation58]. Laponite has a negative surface charge due to the presence of surface hydroxyl groups. Owing to this, it is prone to electrostatic interactions with positively charged polymers. These interactions allow Laponite to be uniformly dispersed in the hydrogel, making the resulting nanocomposite hydrogel highly stable [Citation59, Citation60].
Several studies have reported that Laponite composite hydrogels can promote osteogenesis through multiple pathways. For example, its degradation product (magnesium ions) can promote osteogenesis by activating the hypoxia-inducible factor-1 (HIF-1α) and peroxisome proliferator-activated receptor-γ coactivator-1α (PGC-1α) signalling pathways [Citation61], and direct the differentiation of gel-encapsulated cells (e.g. endothelial cells and mesenchymal stem cells) [Citation55, Citation62]. In addition to the interaction with cells, some studies have suggested that nanoclay is internally ‘bioactive’ and can initiate biomineralisation to enhance calcium phosphate (CaP) deposition and thus promote osteogenesis [Citation63–66]. Owing to the size and shape of clay nanoparticles, theoretically, they can be endocytosed by cells (optimal particle size for endocytosis is 25–30 nm). The clay nanoparticles interact with cells after endocytosis to exert their osteogenesis-promoting effects, but the exact mechanism is not clear [Citation54, Citation67, Citation68].
In addition to the biological function of promoting osteogenesis, nanosilicates can also combine with various substances for bone repair, such as DNA Multifunctional DNA nanostructures have been created for drug delivery and therapy [Citation69]. The surface of a nanosilicate (Laponite XLS) has an anisotropic charge distribution and can interact with the phosphate groups on the DNA backbone through non-covalent bonds, which not only enhances the mechanical strength of the hydrogel but also loads the DNA for slow release. Based on this property, a research team has developed an injectable DNA-nSi nanocomposite hydrogel with a porous structure and shear-thinning property () [Citation70].(The shear-thinning nanocomposite hydrogels can be easily injected using a fine 22-gauge needle, and because of their ability to reassemble after shear stress is removed, gelation occurs almost immediately after injection. The injectable nature allows it to be used in minimally invasive procedures, reducing the risk of blood loss and infection, and it can be used for filling irregular bone defects.
Figure 2. Schematic representation of the design strategy for the development of multifunctional nanocomposite hydrogels. DNA—nSi injectable hydrogels are formed viaa two-step gelation method. The first step consists of an intermediate weak gel (pregel) formation by heating and subsequent cooling of double-stranded DNA. The denaturation of doublestranded DNA followed by rehybridization in a random fashion facilitates the development of interconnections between adjacent DNA strands (type A network points) via complementary base pairing. Introduction of nSi in the second step of the gelation process increases the number of network points (type B) viaelectrostatic interaction with the DNA backbone, resulting in a shear-thinning injectable hydrogel. Reprinted with permission from Basu et al. (2018) [Citation70].
![Figure 2. Schematic representation of the design strategy for the development of multifunctional nanocomposite hydrogels. DNA—nSi injectable hydrogels are formed viaa two-step gelation method. The first step consists of an intermediate weak gel (pregel) formation by heating and subsequent cooling of double-stranded DNA. The denaturation of doublestranded DNA followed by rehybridization in a random fashion facilitates the development of interconnections between adjacent DNA strands (type A network points) via complementary base pairing. Introduction of nSi in the second step of the gelation process increases the number of network points (type B) viaelectrostatic interaction with the DNA backbone, resulting in a shear-thinning injectable hydrogel. Reprinted with permission from Basu et al. (2018) [Citation70].](/cms/asset/2ea02925-0f7e-40a7-a9af-04ef91265502/ynan_a_2076025_f0002_c.jpg)
Nanoclay has the advantages of high surface reactivity and extensive interactions with polymers, cells, and minerals. The nanocomposite hydrogels made from nanoclay have significantly enhanced mechanical strength while maintaining the physical properties of the hydrogel (the nanoclay acts as a physical crosslinking agent) [Citation66, Citation71–73]. The results of Haraguchi K's research show that the elongation at break of nanoclay composite hydrogels is 10 times that of pure hydrogels [Citation74]. Another study reported that the tensile modulus, elongation, and tensile strength of PNIPAAM/Laponite XLG nanocomposite hydrogels were as high as 453 kPa, 1,000%, and 1.1 MPa, respectively. Its breaking energy is more than 1,000 times that of the classic PNIPAAM hydrogel [Citation75] Clay-based nanocomposite hydrogels have significant potential for bone tissue engineering; multiple studies have been conducted to test different combinations of formulations for improving the performance of these hydrogels, while the research on enhancing hydrogel’s bioactivity and osteogenesis on stem cells has also progressed.
3.2. Ceramic-based nanocomposite hydrogel
Ceramics are inorganic materials that are mixtures with the main components of silica and silicates (e.g. aluminium silicate, calcium silicate). Nanoceramic materials have grains and grain boundaries, and their bonding is at the nanometre level. This gives them higher strength, toughness, superplasticity, and electromagnetic properties than ordinary ceramic materials [Citation76]. The addition of nanoceramics can enhance the mechanical properties of hydrogels, thereby increasing the effectiveness of hydrogels for bone repair [Citation26, Citation77, Citation78]. The most widely used inorganic phosphate-based ceramics for bone tissue engineering are calcium phosphates, including hydroxyapatite (HA), β-tricalcium phosphate (β-TCP), and their mixtures—bidirectional calcium phosphate (BCP) [Citation79–81]. The inorganic component of bone is mainly composed of HA; hence, nanohydroxyapatite (nHAp) composite hydrogels have good biocompatibility with bone tissues. Meanwhile, nHAp composite hydrogels promote osteogenesis and vascularisation as well as possess high mechanical strength [Citation82–85]. C Giordano et al. found that the nHA composite hydrogel maintains excellent swelling properties, its maximum deformation in the swollen state increases (from about 0.7% to 19%) and the elastic modulus decreases (from 2027.51 MPa to 19.92 MPa), which also meets the mechanical properties of cancellous bone [Citation86]. In another study, nHA composite hydrogels exhibited excellent mechanical properties. Its tensile stress at break ranges from 0.21 to 0.86 MPa, the tensile strain at break can reach 30 mm/mm, and its compressive strength can reach 35.8 MPa [Citation87]. He et al. reported a polyacrylamide/nHAp hydrogel; with nHAp as a crosslinking agent that binds to the polymer through interactions such as hydrogen bonding, the nanocomposite hydrogel has better mechanical properties than ordinary hydrogels. This hydrogel also has interconnected homogeneous pores that allow better adhesion of mesenchymal stem cells to the scaffold, whereas nHAp promotes osteogenic differentiation of cells [Citation88]. Similarly, Gaharwar et al. proposed a photo-crosslinked elastic nanocomposite hydrogel composed of nHAp and gelatin methacrylate (GelMA) (), which exhibited better mechanical properties and cell adhesion, and promoted mesenchymal stem cells differentiation towards osteogenesis, the use of this nanocomposite hydrogel results in more cartilage tissue production, which promotes more collagen II production compared to pure hydrogels [Citation89, Citation90]. In another study, Jeong et al. also demonstrated the significant enhancing effect of nHAp on the mechanical strength of hydrogels while improving cell adhesion function and promoting osteogenic differentiation of mesenchymal stem cells. In the study, the osteogenesis-related indicators (alkaline phosphatase, bone morphogenetic protein 2, and osteocalcin) of mesenchymal stem cells loaded on the heat-responsive poly(ethylene glycol)-poly(l-alanine-co-l-phenylalanine) (PEG-PAF)/calcium phosphate hydrogels were highly expressed [Citation91]. In addition to directly promoting osteogenesis, nHAp can also regulate the bone immune microenvironment and enhance the activity of vascular endothelial cells, acting as an indirect promoter for osteogenesis [Citation92–94].
Figure 3. Engineering of an osteon-like, cell-laden hydrogel as discussed in Section 2.1.2 a) Schematic representation of the formation of hydroxyapatite nanoparticles in GelMA. b) Schematic representation of the UV-microfabrication procedure employed in the generation of the osteon-like microstructures. c) Bright-field and fluorescence imaging of the resulting biomimetic osteon construct. c) Reprinted with permission from Shi et al. (2016) [Citation90] Copyright 2015, American Chemical Society.
![Figure 3. Engineering of an osteon-like, cell-laden hydrogel as discussed in Section 2.1.2 a) Schematic representation of the formation of hydroxyapatite nanoparticles in GelMA. b) Schematic representation of the UV-microfabrication procedure employed in the generation of the osteon-like microstructures. c) Bright-field and fluorescence imaging of the resulting biomimetic osteon construct. c) Reprinted with permission from Shi et al. (2016) [Citation90] Copyright 2015, American Chemical Society.](/cms/asset/e117a6a1-218d-4c9b-ad6c-069400fdd7a0/ynan_a_2076025_f0003_c.jpg)
Both β-TCP and BCP have also been used to synthesise nanocomposite hydrogels, which are superior to hydrogels synthesised from nHAp in terms of certain properties. Nanocomposite hydrogels formed from natural hydrogels and BCP not only displayed improved mechanical strength and cell adhesion and proliferation but also overcame the drawback of slow degradation of nHAp, Song et al. found that Young's modulus of the BCP nanocomposite hydrogel is more than 2.5 times higher than that of the pure hydrogel, and it has a good interconnected porous structure with a pore size of 65.3 mm [Citation95]. In addition to natural hydrogels, another study reported poly(glycolylated poly(glycerol sebacate)) compounded with β-TCP nanoparticles as a hydrogel. The advantage of this composite hydrogel is that PEG and β-TCP synergistically enhanced osteoconduction and promoted mineralisation, its maximum tensile strength is about 9.58 MPa, which is 1.5 times that of the pure hydrogel group [Citation96].
In addition to the above-mentioned nanoceramic materials, other ceramic-based materials, such as alumina, bioactive glass, and titanium dioxide, with higher elasticity are used in bone tissue engineering. The hydrogels prepared from these ceramic-based materials and different polymers exhibit immunomodulatory, antibacterial, superabrasive, and self-healing properties [Citation16, Citation97–102]. Strontium, an alkaline earth metal, is a novel nanomaterial that promotes osteogenesis and has a significant potential for applications in the field of bone tissue engineering. Strontium-based nanocomposite hydrogels are expected to replace the current ceramic-based nanocomposite hydrogels; however, it has only been reported in limited studies so far.
3.3. Iron oxide-based nanocomposite hydrogel
Iron oxide nanoparticles are biocompatible and can adhere to cells, while the composite hydrogels based on iron oxide nanoparticles have magnetic and thermal responsive properties [Citation103–105]. Iron oxide nanocomposite hydrogel as a magnetic biomaterial has been proven to promote osteogenesis [Citation106]. The possible mechanism is that the contact between cells and magnetic materials generates nanoscale forces similar to mechanical forces, which stretches cell membranes and activates the associated channels and receptors [Citation107]. In addition to the effect of intrinsic magnetic field on cells, applied magnetic field interference influences cell behaviour (cell proliferation, migration direction, and differentiation) [Citation108–112]. Besides the effect of magnetic field, studies have found that iron oxide nanoparticles exhibit a peroxidase-like ability in a cytoplasmic environment to activate the p38 mitogen-activated protein kinase (MAPK) signalling pathway to accelerate cell cycle progression and promote cell proliferation [Citation113, Citation114]. So far, many iron oxide-based nanocomposite hydrogels have been developed for bone tissue engineering applications. These nanocomposite hydrogels have excellent mechanical strength and magnetic-responsive properties that can be tuned by the type, content, and size of the iron oxide particles. For example, in a chitosan hydrogel with iron oxide nanoparticles, the mechanical properties of the hydrogel improved with an increase in the content of the incorporated magnetic particles. The strength and elastic modulus of the iron oxide nanocomposite hydrogel were increased by 416% and 265% compared with the pure hydrogel group. Under the action of a low-frequency alternating magnetic field (LAMF), the drug in the nanocomposite hydrogel was switched from passive release to pulsed release, and the cumulative and partial drug release was increased by 67.2% and 31.9% compared with the pure hydrogel group [Citation28]. In another study, it was found that larger iron oxide nanoparticles could effectively avoid aggregation, better crosslink with the hydrogel, and improve the mechanical strength of the composite. The addition of iron nanoparticles increased the mechanical stiffness of the hydrogel by 10 times and the toughness by 20 times, while the mechanical stiffness of the composite hydrogel was tuned by changing the concentration and size of the iron nanoparticles (0.2KPa∼200KPa) [Citation107, Citation115]. The addition of iron oxide nanoparticles not only enhanced the mechanical strength of the composite hydrogels but also added more suitable cell adhesion sites. For example, in a mechanically rigid collagen/iron oxide (Fe3O4) NPs hydrogel, the iron oxide nanoparticles acted as the crosslinking agent and substantially enhanced the hydrogel strength and increased the number of cell adhesion sites () [Citation115]. Externally applied magnetic fields can also alter the properties of iron oxide nanocomposite hydrogels by causing vibrational and rotational displacements of magnetic particles. This results in microscopic-level changes in the shape of the composite and the appearance of increased micropores on the surface of the hydrogel that are favourable for cell growth. Based on this phenomenon, iron oxide nanoparticles were added into hydrogels containing arginine glycyl aspartate (RGD) to dynamically regulate cell-matrix interactions through an applied magnetic field (), promoting cell proliferation and differentiation, and ultimately realising tissue regeneration [Citation116]. The strength of the magnetic field also influences the proliferation and differentiation functions of the cells. It has been reported that an applied magnetic field of moderate strength (from 1 mT to 1 T) can effectively promote mesenchymal stem cell proliferation and osteogenic differentiation [Citation43, Citation107]. In addition to directly promoting osteogenesis, iron oxide nanocomposite hydrogels can indirectly promote bone regeneration by enhancing blood vessel formation. The composite hydrogel formed by adding iron oxide nanoparticles to the PEG hydrogel containing stromal vascular component cells of human adipose tissues can promote bone tissue formation as well as blood vessel angiogenesis in tissues. The mechanism behind this may be that the magnetic drive stimulates the function of progenitor cells, as evidenced by an increase in indicators such as vascular endothelial growth factor (VEGF) and enrichment of CD31-positive cells [Citation33].
Figure 4. Synthesis of MNP-reinforced nanocomposite hydrogels. (a) Nanocomposite hydrogels were synthesized by combining PEG-dopamine-MNPs with GelMA and exposing it to UV radiation in the presence of a photoinitiator to obtain covalently crosslinked network. (b) Crosslinking of the prepolymer solution was monitor using UV rheology. After the UV radiation was turned on, a significant increase in the modulus of the prepolymer solution was observed, indicating the formation of a crosslinked network. (c) The presence of MNPs within nanocomposite network was determined from XPS. (d) TEM images of nanocomposite show uniform distribution of MNPs within GelMA matrix. Inset show the electron diffraction pattern. (e) SEM micrographs of the nanocomposites indicate the formation of highly porous and interconnected networks. Reprinted with permission from Jaiswal et al. (2016) [Citation115].
![Figure 4. Synthesis of MNP-reinforced nanocomposite hydrogels. (a) Nanocomposite hydrogels were synthesized by combining PEG-dopamine-MNPs with GelMA and exposing it to UV radiation in the presence of a photoinitiator to obtain covalently crosslinked network. (b) Crosslinking of the prepolymer solution was monitor using UV rheology. After the UV radiation was turned on, a significant increase in the modulus of the prepolymer solution was observed, indicating the formation of a crosslinked network. (c) The presence of MNPs within nanocomposite network was determined from XPS. (d) TEM images of nanocomposite show uniform distribution of MNPs within GelMA matrix. Inset show the electron diffraction pattern. (e) SEM micrographs of the nanocomposites indicate the formation of highly porous and interconnected networks. Reprinted with permission from Jaiswal et al. (2016) [Citation115].](/cms/asset/128eada6-0905-40ff-9991-33c5fee54537/ynan_a_2076025_f0004_c.jpg)
Figure 5. Illustration of modulating cell − matrix interactions and mechanosensing of hMSCs by using a MNP-borne soft hydrogel substrate under a directional magneticfield. (A, B) Upward/downward magnetic attraction induces the EXPOSED/HIDDEN presentation of MNPs grafted with cell adhesive ligand on the hydrogel surface to trigger the activation/inactivation of cell mechanotransduction signaling, respectively. (C) Detailed chemical structures in a gray dashed square from (A). Reprinted with permission from Wong et al. (2020) [Citation116].
![Figure 5. Illustration of modulating cell − matrix interactions and mechanosensing of hMSCs by using a MNP-borne soft hydrogel substrate under a directional magneticfield. (A, B) Upward/downward magnetic attraction induces the EXPOSED/HIDDEN presentation of MNPs grafted with cell adhesive ligand on the hydrogel surface to trigger the activation/inactivation of cell mechanotransduction signaling, respectively. (C) Detailed chemical structures in a gray dashed square from (A). Reprinted with permission from Wong et al. (2020) [Citation116].](/cms/asset/508d0995-d08c-4e60-b823-d41e2e875146/ynan_a_2076025_f0005_c.jpg)
3.4. Black phosphorus (BP)-based nanocomposite hydrogel
Black phosphorus (BP) is a nanomaterial composed of a single element, phosphorus, with stable chemical properties and excellent biocompatibility [Citation117]. During bone remodelling, bone mineralisation induced by proper calcium and phosphorus metabolism is required in addition to the involvement of osteoblasts and osteoclasts [Citation118, Citation119]. BP is highly homologous to the inorganic components of bones [Citation120]. Its degradation products are nontoxic phosphates, which can promote calcium deposition in bone tissues and facilitate bone repair. The compressive modulus of this nanocomposite hydrogel is more than 2 times that of the pure hydrogel group, and it can also form more surface mineralization to promote bone formation [Citation121]. Based on this feature, researchers constructed a BP/PEA/GelMA composite hydrogel system for bone tissue engineering, which was prepared by photo-crosslinking GelMA, BP nanosheets (BPN), and cationic arginine-based unsaturated polyesteramide. BPNs were encapsulated inside the hydrogel, which not only improved the mechanical strength of the hydrogel but also released phosphorus ions upon degradation to promote bone defect repair. Its compressive modulus is 3-4 times higher than that of the pure hydrogel. In vitro and in vivo experiments have shown that it can form more mineralization than pure hydrogel, and the final bone repair effect is better [Citation121]. In another study, researchers constructed a BPN composite double network (DN) hydrogel, with the ductile network comprising PHEA, PDMA, or PAM and the brittle network comprising chitosan-methacrylate (ChiMA), alginate-methacrylate (AlgMA), or gelatin-methacrylate (GelMA). BPNs were compounded into various double network hydrogels in different combinations. Under weak alkaline conditions, the composite hydrogel could induce accelerated CaP nanocrystal formation via BPNs to mimic biomineralisation, thereby promoting bone formation [Citation122]. In addition, BPNs have excellent light absorption in the near-infrared (NIR) region, which enables stable and durable photo-controlled regulation of the release of loaded active substances as well as photothermal conversion and thermal therapy at the composite hydrogel sites [Citation123]. Local thermal therapy has been confirmed to enhance the expression of alkaline phosphatase (ALP) and heat shock protein (HSP), thereby accelerating bone regeneration [Citation124–126]. Based on this property, researchers constructed a heat-responsive BPN/PRP/chitosan hydrogel system to treat bone defects caused by rheumatoid arthritis (RA). The BPN in this composite hydrogel generates local heat in the NIR region while producing reactive oxygen species (ROS), which are delivered to diseased joints to treat abnormal synovial hyperplasia. The composite hydrogel can also precisely control the release of BP degradation products (phosphorus ions), providing sufficient raw material for bone regeneration and facilitating the repair of bone defects caused by RA [Citation127].
Owing to their high specific surface area, BPNs are excellent drug carriers(its drug loading efficiency can reach 950%), and drugs are adsorbed on BPNs through non-chemical bonding(this property preserves drug activity and purity); controlled drug release can be achieved under NIR light irradiation due to the photothermal effect of BPNs [Citation128]. Based on this property, researchers developed PLGA hydrogel microspheres combined with SrCl2 and BPNs for bone regeneration. The composite exhibited good light absorption and photothermal effects under NIR light, and its temperature increases by 24 °C after 10 min of NIR light irradiation. The BPN-induced photothermal conversion generated heat at the nanocomposite hydrogel sites to break the hydrogen bonds in the network. After turning off the light irradiation, the heat dissipated, the nanocomposite hydrogel cooled down gradually, and the broken hydrogen bonds reappeared in the nanocomposite hydrogel [Citation129]. Therefore, we conclude that the BP-based nanocomposite hydrogels possess multiple functions, including stronger mechanical properties, promotion of bone formation, stronger drug loading properties, and photothermal properties. It shows great potential in bone tissue engineering, especially in repairing bone defects caused by bone tumors
3.5. Carbon-based nanocomposite hydrogel
Owing to their excellent optical, mechanical, and electrical properties, carbon-based nanomaterials, such as graphene and carbon nanotubes (CNTs), have been used to prepare nanocomposite hydrogels with unique properties. After the addition of CNTs, the elastic modulus of GelMA hydrogel increased from 15KPa to 60KPa and the compressive modulus increased from 10KPa to 30KPa similar to the compressive modulus of bone matrix (25 − 40 kPa). At the same time, the nanocomposite hydrogel maintains a highly microporous structure. However, the elongation at break of the GelMA hydrogel (72%) is higher than that of the CNT-incorporated hydrogel (58%) () [Citation130, Citation131]. Compounding modified carbon-based nanomaterials (e.g. adding polar groups to improve their water solubility) with hydrogels, the mechanical strength of hydrogels can be substantially enhanced and the synthesised nanocomposite hydrogels can be equipped with other properties (e.g. electrical properties, optical properties, and elasticity) [Citation132, Citation133]. Although CNTs have many successful applications in biomedical engineering, some studies have reported that the use of carbon nanotubes may induce increased cytotoxicity [Citation134, Citation135].
Figure 6. (a) Schematic description of gelatin methacrylate (GelMA) coated onto a carbon nanotube (CNT). (b) High resolution TEM image of CNT-GelMA. (c) Stress-strain curves of CNTGelMA hydrogels with various concentrations of CNTs. (d) Tensile moduli of CNT-GelMA hydrogels. (e) 3T3 fibroblasts cultured on GelMA hydrogel (A) and CNT-GelMA hydrogel (B).(Scale bar: 100 μm) Reprinted with permission from Shin et al. (2012) [Citation131]. Copyright 2011 American Chemical Society.
![Figure 6. (a) Schematic description of gelatin methacrylate (GelMA) coated onto a carbon nanotube (CNT). (b) High resolution TEM image of CNT-GelMA. (c) Stress-strain curves of CNTGelMA hydrogels with various concentrations of CNTs. (d) Tensile moduli of CNT-GelMA hydrogels. (e) 3T3 fibroblasts cultured on GelMA hydrogel (A) and CNT-GelMA hydrogel (B).(Scale bar: 100 μm) Reprinted with permission from Shin et al. (2012) [Citation131]. Copyright 2011 American Chemical Society.](/cms/asset/2d9c2ddd-fd82-4c1e-81e9-cd159b560d1c/ynan_a_2076025_f0006_c.jpg)
Graphene is a novel material in which carbon atoms connected by sp2 hybridisation are tightly packed into a monolayer two-dimensional honeycomb lattice structure. Its derivatives include graphene oxide (GO), carboxyl graphene (CXYG), reduced graphene oxide (rGO), and graphene quantum dots (GQDs) [Citation136]. GO contains hydroxyl and carboxyl groups that make it hydrophilic and enable it to disperse uniformly in hydrogels. GO exhibits good biocompatibility and promotes cell proliferation and differentiation, in vitro studies confirmed that GO can promote the differentiation of hMSCs towards osteogenesis [Citation137]. It provides a microenvironment for cell proliferation while controlling cell differentiation; moreover, its broad surface can provide interaction sites for proteins and cytokines, enriching osteogenesis-related factors and minerals. In addition, GO also has excellent antibacterial properties; graphene family materials can act as electron acceptors and cause non-dependent oxidation of superoxide anions in bacteria to kill bacteria [Citation138–140]. Based on these properties of GO, its complexation with hydrogels has led to the synthesis of several nanocomposite hydrogels for bone tissue engineering applications. For example, scaffolds with dual functions of inducing bone regeneration and preventing bacterial infection were fabricated by depositing GO on a polydopamine (PDA)-modified titanium scaffold to form a GO/Ti porous scaffold and then assembling gelatin microspheres (GelMS) encapsulated with 2(bone morphogenetic protein) (BMP2) and vancomycin (Van) on the scaffold, the common stent released 76.9% of the loaded drug in 5 days, while the nanocomposite hydrogel stent released 63.4% of the loaded drug in 21 days [Citation141]. Moreover, an alginate/GO composite as a 3 D printing ink for repairing bone defects has been reported. This nanocomposite hydrogel improved cell printing performance and increased cell survival rate and differentiation capability. The GO-added hydrogels all exhibited shear-thinning properties, and the shear moduli of alginate/GO-0.5 and alginate/GO-1.0 at 1 Hz were 1.19 and 1.23 times higher than those of alginate/GO-0, respectively [Citation142]. Similarly, nanocomposite hydrogel scaffolds (GO/PDLLA) have been proposed to promote cartilage regeneration by releasing TGF-β3. Many studies have incorporated transforming growth factor-β (TGF-β) into scaffolds to promote cartilage repair, but TGF-β has limited utility due to its short functional half-life and/or rapid clearance. TGF-β loaded by GO/PDLLA hydrogel scaffolds can be released stably for 4 weeks, and the compressive modulus of GO/PDLLA hydrogel is about 1.32 to 1.45 times higher than that of control PDLLA hydrogel [Citation143]. GO can also enhance the mechanical strength of hydrogels. It has been reported that the addition of graphene to sericin methacryloyl (SerMA) hydrogels (SMH) can improve the compression modulus of the composite. Specifically, the compression modulus of the composite hydrogel increased by 17 kPa upon increasing the content of GO from 0.02% to 0.04% (the compressive modulus is 17 kPa for SMH, 34 kPa for SMH/GO-0.01%, and 43 kPa for SMH/GO-0.02%), and the SMH/GO hydrogel exhibited a superior therapeutic effect in a rat cranial defect model () [Citation144]. GO-reinforced regenerated cellulose/polyvinyl alcohol (GO-RCE/PVA) ternary hydrogels were prepared in another study, and it was confirmed that the elongation at break and tensile strength of the composite hydrogels significantly increased on increasing the GO content. With the addition of 1.0 wt% GO, the tensile strength increased from 0.52 MPa to 0.73 MPa (40.4% increase) and the elongation at break increased from 103% to 238%. With the addition of 0.8 wt% GO, the swelling ratio of the GO-RCE/PVA ternary hydrogel increased from 150% to 310% [Citation145].
Figure 7. (A). SMH/GO hydrogels as an artificial bone substitute for bone regeneration in a rat calvarial defect model via regulating BMSCs migration and osteogenesis differentiation.
Characterizations of SMH/GO hydrogels. (B) Preparation of SMH/GO hydrogels via UV light at 365 nm. (C) FTIR spectra of SMH/GO hydrogels. (D) Mechanical properties of SMH/GO hydrogels. (E) Degradation profiles of SMH/GO hydrogels in PBS (pH7.4, 37 oC). (F) Scanning electron micrographs of SMH (left), SMH/GO-1 (middle) and SMH/GO-2 (right). Scale Bars, 500μm (upper panel) and 10μm (lower panel). Red arrowheads indicate graphene oxide. Reprinted with permission from Qi et al. (2020) [Citation144].
![Figure 7. (A). SMH/GO hydrogels as an artificial bone substitute for bone regeneration in a rat calvarial defect model via regulating BMSCs migration and osteogenesis differentiation.Characterizations of SMH/GO hydrogels. (B) Preparation of SMH/GO hydrogels via UV light at 365 nm. (C) FTIR spectra of SMH/GO hydrogels. (D) Mechanical properties of SMH/GO hydrogels. (E) Degradation profiles of SMH/GO hydrogels in PBS (pH7.4, 37 oC). (F) Scanning electron micrographs of SMH (left), SMH/GO-1 (middle) and SMH/GO-2 (right). Scale Bars, 500μm (upper panel) and 10μm (lower panel). Red arrowheads indicate graphene oxide. Reprinted with permission from Qi et al. (2020) [Citation144].](/cms/asset/20bb8ea5-7930-4626-96d5-c2fbe85430a3/ynan_a_2076025_f0007_c.jpg)
4. Summary
Bone is a highly organized composite material consisting of 50-70% inorganic components (mainly hydroxyapatite (HAp), 20-40% organic components (mainly type I collagen), 5-10% Water, and 3% lipid. Macroscopically, bone can be divided into the outer hard cortical bone and inner spongy cancellous bone [Citation146]. Bone tissue engineering aims to develop new bone repair strategies to regenerate and repair damaged or diseased bone tissue, replacing more traditional bone grafting methods (such as autografts or allografts).
Materials used for bone tissue engineering should possess some basic properties. Such as biocompatibility: the materials used should allow cell adhesion, proliferation, and differentiation without cytotoxicity and excessive immune response during implantation [Citation147]. For example, osteoconductivity and osteoinductivity refer to the properties of materials that can promote the precipitation of bone on non-material surfaces and the differentiation of mesenchymal stem cells, respectively [Citation148]. The implanted biomaterial should have sufficient mechanical strength to support the bone tissue. For example, the elastic modulus of cortical bone is 14 ∼ 20 GPa, the compressive strength is 130 ∼ 225 MPa, and the elastic modulus of cancellous bone is 1 ∼ 2 GPa, the compressive strength is 7 ∼ 10 MPa, and the compressive modulus of cartilage is 1.9 ∼ 15 MPa [Citation149–151]. The interconnected porous structure of the material is also a key factor in the design of scaffolds for bone repair, which allows native cell migration and proliferation. In the structure of bone tissue, cancellous bone is highly porous (30-90% porosity) and cortical bone has only 5-30% porosity, so most metabolic activity occurs in cancellous bone. Typically, materials with pore sizes above 200 µm can promote new bone formation and vascularization [Citation55]. The geometry of the porous structure has a profound effect on both the mechanical and biological responses of bone tissue, and 3 D printing technology enables the production of customized bone tissue engineering scaffolds that can tune its porosity, mechanical properties, and structure. For 3 D printing strategies, printable bioinks are the key to success. Several aspects should be considered for printable bioinks, such as rheological behavior (viscosity and shear thinning), surface tension, swelling, gelling kinetics, and mechanical properties of the material. Another important aspect is the cross-linking (physical, chemical or enzymatic) of the bioink during processing and post-processing steps to maintain the biomechanical stability of the printed product structure [Citation152].
Nanocomposite hydrogels represent a new generation of advanced biomaterials that are widely used in bone tissue engineering. Bone tissue engineering aims to repair bone defects caused by various reasons and faces diverse challenges. Nanocomposite hydrogels have good biocompatibility and can mimic the cellular matrix environment; cells can grow and proliferate well in the scaffold; and various cytokines can flow in the porous three-dimensional structure. Moreover, nanocomposite hydrogels can gradually degrade and get absorbed in the process of bone repair, providing space for bone regeneration. Furthermore, its degradation products are non-toxic and can even promote osteogenesis (e.g. the degradation products are calcium and phosphorus ions). The scaffold material used for bone repair should also have a certain amount of mechanical strength. By adding hard inorganic nanomaterials, the mechanical strength of nanocomposite hydrogels can be enhanced and adjusted by changing the formula. Moreover, multi-layered bionic structures can also be constructed for different load-bearing stages. Vascular coupling is important in bone repair; the porous structure of nanocomposite hydrogels can facilitate oxygen exchange and vascular endothelial cell growth, and the addition of appropriate nanomaterials and encapsulation of pro-vascular factors can facilitate the angiogenesis of blood vessels. Bone repair is more difficult in infected and inflammatory environments. To address this problem, the addition of nanomaterials with antimicrobial and anti-inflammatory capabilities can equip the hydrogels with antimicrobial and anti-inflammatory effects in bone regeneration to promote osteogenesis. The incorporation of various nanomaterials can endow the composite hydrogels with stimulus-responsive properties, such as heat and magnetic responses, which can further enhance bone repair performance while providing a new strategy for controlled drug release. We summarize the properties of various types of nanocomposite hydrogels in .
Table 1. The properties of nanocomposite hydrogels.
In general, the nanocomposite hydrogels used for bone tissue engineering should be based on various factors of bone repair. By reasonable design and matching of nanomaterials and polymers, nanocomposite hydrogels have been developed with multiple adjustable functions. These functions include mechanical strength, elasticity, surface topography, controlled biodegradability, wettability, self-healing property, and stimulus responsiveness. Moreover, the cell loading capacity of nanocomposite hydrogels is an important factor. The effect of nanomaterials on the fate of mesenchymal stem cells should be explored, as it is an important factor for the construction of suitable bioactive materials.
Disclosure statement
No potential conflict of interest was reported by the author(s).
Additional information
Funding
Notes on contributors
Chengcheng Du
Chengcheng Du holds a bachelor's degree in clinical medicine from Nanchang University and a bachelor's degree in biomedicine from Queen Mary University of London and is currently studying for a master's degree in orthopaedics at Chongqing Medical University. His research focuses on bone defect repair with mesenchymal stem cells combined with nanocomposite hydrogels.
Wei Huang
Wei Huang is the director and professor of the First Affiliated Hospital of Chongqing Medical University and the head of the Orthopaedic Research Institute of Chongqing Medical University. His research focuses on bone defect repair and cartilage regeneration. Currently developed antibacterial bone repair materials, nano-bone filling materials and porous tantalum have achieved clinical transformation, and he is working on the development of biomaterials for cartilage repair.
References
- Qasim M, Chae DS, Lee NY. Advancements and frontiers in nano-based 3D and 4D scaffolds for bone and cartilage tissue engineering. Int J Nanomedicine. 2019;14:4333–4351.
- Annabi N, Tamayol A, Uquillas JA, et al. 25th anniversary article: Rational design and applications of hydrogels in regenerative medicine. Adv Mater. 2014;26(1):85–124.
- Abdollahiyan P, Oroojalian F, Mokhtarzadeh A, et al. Hydrogel-Based 3D bioprinting for bone and cartilage tissue engineering. Biotechnol J. 2020;15(12):e2000095.
- Niemczyk-Soczynska B, Zaszczyńska A, Zabielski K, et al. Hydrogel, electrospun and composite materials for bone/cartilage and neural tissue engineering. Mater Basel Switz. 2021;14(22):6899.
- Annabi N, Nichol JW, Zhong X, et al. Controlling the porosity and microarchitecture of hydrogels for tissue engineering. Tissue Eng Part B Rev. 2010;16(4):371–383.
- Yue K, Trujillo-de Santiago G, Alvarez MM, et al. Synthesis, properties, and biomedical applications of gelatin methacryloyl (GelMA) hydrogels. Biomaterials. 2015;73:254–271.
- Zhao H, Liu M, Zhang Y, et al. Nanocomposite hydrogels for tissue engineering applications. Nanoscale. 2020;12(28):14976–14995.
- Turnbull G, Clarke J, Picard F, et al. 3D bioactive composite scaffolds for bone tissue engineering. Bioact Mater. 2018;3(3):278–314.
- Jacob S, Nair AB, Shah J, et al. Emerging role of hydrogels in drug delivery systems, tissue engineering and wound management. Pharmaceutics. 2021;13(3):357.
- Chaudhari AA, Vig K, Baganizi DR, et al. Future prospects for scaffolding methods and biomaterials in skin tissue engineering: a review. IJMS. 2016;17(12):1974.
- Han IK, Chung T, Han J, et al. Nanocomposite hydrogel actuators hybridized with various dimensional nanomaterials for stimuli responsiveness enhancement. Nano Converg. 2019;6(1):18.
- Zhang X, Megone W, Peijs T, et al. Functionalization of electrospun PLA fibers using amphiphilic block copolymers for use in carboxy-methyl-cellulose hydrogel composites. Nanocomposites. 2020;6(3):85–98.
- Samba I, Hernandez R, Rescignano N, et al. Nanocomposite hydrogels based on embedded PLGA nanoparticles in gelatin. Nanocomposites. 2015;1(1):46–50.
- Chen T, Hou K, Ren Q, et al. Nanoparticle-Polymer synergies in nanocomposite hydrogels: from design to application. Macromol Rapid Commun. 2018;39(21):e1800337.
- Caseri WR. Nanocomposites of polymers and inorganic particles: preparation, structure and properties. Mater Sci Technol. 2006;22(7):807–817.
- Li B, Zhang L, Wang D, et al. Thermosensitive -hydrogel-coated titania nanotubes with controlled drug release and immunoregulatory characteristics for orthopedic applications. Mater Sci Eng C Mater Biol Appl. 2021;122:111878.
- Pelgrift RY, Friedman AJ. Nanotechnology as a therapeutic tool to combat microbial resistance. Adv Drug Deliv Rev. 2013;65(13–14):1803–1815.
- Wahid F, Zhong C, Wang H-S, et al. Recent advances in antimicrobial hydrogels containing metal ions and metals/metal oxide nanoparticles. Polymers. 2017;9(12):636.
- Mehrali M, Thakur A, Pennisi CP, et al. Nanoreinforced hydrogels for tissue engineering: Biomaterials that are compatible with Load-Bearing and electroactive tissues. Adv Mater. 2017;29(8):1603612.
- Huang Q, Zou Y, Arno MC, et al. Hydrogel scaffolds for differentiation of adipose-derived stem cells. Chem Soc Rev. 2017;46(20):6255–6275.
- Prasad AS, Wang Y, Li X, et al. Investigating the effect of surface modification on the dispersion process of polymer nanocomposites. Nanocomposites. 2020;6(3):111–124.
- Sadak O. One-pot scalable synthesis of rGO/AuNPs nanocomposite and its application in enzymatic glucose biosensor. Nanocomposites. 2021;7(1):44–52.
- Lu L, Yuan S, Wang J, et al. The formation mechanism of hydrogels. Curr Stem Cell Res Ther. 2018;13(7):490–496.
- Oryan A, Kamali A, Moshiri A, et al. Chemical crosslinking of biopolymeric scaffolds: Current knowledge and future directions of crosslinked engineered bone scaffolds. Int J Biol Macromol. 2018;107(Pt A):678–688.
- Burdick JA, Prestwich GD. Hyaluronic acid hydrogels for biomedical applications. Adv Mater. 2011;23(12):H41–56.
- Zhang Y, Chen Q, Dai Z, et al. Nanocomposite adhesive hydrogels: from design to application. J Mater Chem B. 2021;9(3):585–593.
- Gaharwar AK, Peppas NA, Khademhosseini A. Nanocomposite hydrogels for biomedical applications. Biotechnol Bioeng. 2014;111(3):441–453.
- Wang Y, Li B, Xu F, et al. Tough magnetic chitosan hydrogel nanocomposites for remotely stimulated drug release. Biomacromolecules. 2018;19(8):3351–3360.
- Haraguchi K, Ebato M, Takehisa T. Polymer–clay nanocomposites exhibiting abnormal necking phenomena accompanied by extremely large reversible elongations and excellent transparency. Adv Mater. 2006;18(17):2250–2254.
- Zhu M, Liu Y, Sun B, et al. A novel highly resilient nanocomposite hydrogel with low hysteresis and ultrahigh elongation. Macromol Rapid Commun. 2006;27(13):1023–1028.
- Adnan MM, Dalod ARM, Balci MH, et al. In situ synthesis of hybrid inorganic–polymer nanocomposites. Polymers. 2018;10(10):1129.
- Lü C, Yang B. High refractive index organic–inorganic nanocomposites: design, synthesis and application. J Mater Chem. 2009;19(19):2884–2901.
- Filippi M, Dasen B, Guerrero J, et al. Magnetic nanocomposite hydrogels and static magnetic field stimulate the osteoblastic and vasculogenic profile of adipose-derived cells. Biomaterials. 2019;223:119468.
- Liu Z, Liu J, Cui X, et al. Recent advances on magnetic sensitive hydrogels in tissue engineering. Front Chem. 2020;8:124.
- Tong X, Zheng J, Lu Y, et al. Swelling and mechanical behaviors of carbon nanotube/poly(vinyl alcohol) hybrid hydrogels. Mater Lett. 2007;61(8–9):1704–1706.
- Miwa Y, Zinchenko A, Lopatina LI, et al. Size control of gold nanoparticles synthesized in a DNA hydrogel. Polym Int. 2014;63(9):1566–1571.
- Ponnamma D, Cabibihan J-J, Rajan M, et al. Synthesis, optimization and applications of ZnO/polymer nanocomposites. Mater Sci Eng C Mater Biol Appl. 2019;98:1210–1240.
- Zhang Q, Li Q-L, Xiang S, et al. Covalent modification of graphene oxide with polynorbornene by surface-initiated ring-opening metathesis polymerization. Polymer. 2014;55(23):6044–6050.
- Messing R, Frickel N, Belkoura L, et al. Cobalt ferrite nanoparticles as multifunctional cross-linkers in PAAm ferrohydrogels. Macromolecules. 2011;44(8):2990–2999.
- Hu X, Nian G, Liang X, et al. Adhesive tough magnetic hydrogels with high Fe3O4 content. ACS Appl Mater Interfaces. 2019;11(10):10292–10300.
- Ju Y, Zhang Y, Zhao H. Fabrication of polymer-protein hybrids. Macromol Rapid Commun. 2018;39(7):e1700737.
- Chen P, Xie F, Tang F, et al. Structure and properties of thermomechanically processed silk peptide and nanoclay filled chitosan. Nanocomposites. 2020;6(3):125–136.
- Pardo A, Gómez-Florit M, Barbosa S, et al. Magnetic nanocomposite hydrogels for tissue engineering: Design concepts and remote actuation strategies to control cell fate. ACS Nano. 2021;15(1):175–209.
- Arias SL, Shetty A, Devorkin J, et al. Magnetic targeting of smooth muscle cells in vitro using a magnetic bacterial cellulose to improve cell retention in tissue-engineering vascular grafts. Acta Biomater. 2018;77:172–181.
- Liu K, Han L, Tang P, et al. An anisotropic hydrogel based on Mussel-Inspired conductive ferrofluid composed of electromagnetic nanohybrids. Nano Lett. 2019;19(12):8343–8356.
- Shen X, Tong H, Zhu Z, et al. A novel approach of homogenous inorganic/organic composites through in situ precipitation in poly-acrylic acid gel. Mater Lett. 2007;61(3):629–634.
- Caseri WR. In Situ synthesis of polymer-embedded nanostructures. Nanocomposites. John Wiley & Sons, Ltd; 2013. p. 45–72.
- Kangwansupamonkon W, Jitbunpot W, Kiatkamjornwong S. Photocatalytic efficiency of TiO2/poly[acrylamide-co-(acrylic acid)] composite for textile dye degradation. Polym Degrad Stab. 2010;95(9):1894–1902.
- Stoyneva V, Momekova D, Kostova B, et al. Stimuli sensitive super-macroporous cryogels based on photo-crosslinked 2-hydroxyethylcellulose and chitosan. Carbohydr Polym. 2014;99:825–830.
- Li X, Dong S, Yan H, et al. Fabrication and properties of PVA-TiO2 hydrogel composites. Procedia Eng. 2012;27:1488–1491.
- Huang J, Liang Y, Jia Z, et al. Development of magnetic nanocomposite hydrogel with potential cartilage tissue engineering. ACS Omega. 2018;3(6):6182–6189.
- Sposito G, Skipper NT, Sutton R, et al. Surface geochemistry of the clay minerals. Proc Natl Acad Sci U S A. 1999;96(7):3358–3364.
- Kotal M, Thakur AK, Bhowmick AK. Polyaniline-carbon nanofiber composite by a chemical grafting approach and its supercapacitor application. ACS Appl Mater Interfaces. 2013;5(17):8374–8386.
- Mihaila SM, Gaharwar AK, Reis RL, et al. The osteogenic differentiation of SSEA-4 Sub-population of human adipose derived stem cells using silicate nanoplatelets. Biomaterials. 2014;35(33):9087–9099.
- Xavier JR, Thakur T, Desai P, et al. Bioactive nanoengineered hydrogels for bone tissue engineering: a growth-factor-free approach. ACS Nano. 2015;9(3):3109–3118.
- Bitinis N, Hernandez M, Verdejo R, et al. Recent advances in clay/polymer nanocomposites. Adv Mater. 2011;23(44):5229–5236.
- Paul A, Manoharan V, Krafft D, et al. Nanoengineered biomimetic hydrogels for guiding human stem cell osteogenesis in three dimensional microenvironments. J Mater Chem B. 2016;4(20):3544–3554.
- Gaharwar AK, Mihaila SM, Swami A, et al. Bioactive silicate nanoplatelets for osteogenic differentiation of human mesenchymal stem cells. Adv Mater. 2013;25(24):3329–3336.
- Mohanty RP, Joshi YM. Chemical stability phase diagram of aqueous laponite dispersions. Appl Clay Sci. 2016;119:243–248.
- Jatav S, Joshi YM. Chemical stability of laponite in aqueous media. Appl Clay Sci. 2014;97–98:72–77.
- Yoshizawa S, Brown A, Barchowsky A, et al. Magnesium ion stimulation of bone marrow stromal cells enhances osteogenic activity, simulating the effect of magnesium alloy degradation. Acta Biomater. 2014;10(6):2834–2842.
- Dawson JI, Kanczler JM, Yang XB, et al. Clay gels for the delivery of regenerative microenvironments. Adv Mater. 2011;23(29):3304–3308.
- Gaharwar AK, Schexnailder PJ, Kline BP, et al. Assessment of using laponite cross-linked poly(ethylene oxide) for controlled cell adhesion and mineralization. Acta Biomater. 2011;7(2):568–577.
- Gaharwar AK, Mukundan S, Karaca E, et al. Nanoclay-enriched poly(ɛ-caprolactone) electrospun scaffolds for osteogenic differentiation of human mesenchymal stem cells. Tissue Eng Part A. 2014;20(15–16):2088–2101.
- Ambre AH, Katti DR, Katti KS. Nanoclays mediate stem cell differentiation and mineralized ECM formation on biopolymer scaffolds. J Biomed Mater Res A. 2013;101(9):2644–2660.
- Mousa M, Evans ND, Oreffo ROC, et al. Clay nanoparticles for regenerative medicine and biomaterial design: a review of clay bioactivity. Biomaterials. 2018;159:204–214.
- Vergaro V, Abdullayev E, Lvov YM, et al. Cytocompatibility and uptake of halloysite clay nanotubes. Biomacromolecules. 2010;11(3):820–826.
- Biddeci G, Spinelli G, Massaro M, et al. Study of uptake mechanisms of halloysite nanotubes in different cell lines. Int J Nanomedicine. 2021;16:4755–4768.
- Xu Y, Wu Q, Sun Y, et al. Three-dimensional self-assembly of graphene oxide and DNA into multifunctional hydrogels. ACS Nano. 2010;4(12):7358–7362.
- Basu S, Pacelli S, Feng Y, et al. Harnessing the noncovalent interactions of DNA backbone with 2D silicate nanodisks to fabricate injectable therapeutic hydrogels. ACS Nano. 2018;12(10):9866–9880.
- Su D, Jiang L, Chen X, et al. Enhancing the gelation and bioactivity of injectable silk fibroin hydrogel with laponite nanoplatelets. ACS Appl Mater Interfaces. 2016;8(15):9619–9628.
- Thakur A, Jaiswal MK, Peak CW, et al. Injectable shear-thinning nanoengineered hydrogels for stem cell delivery. Nanoscale. 2016;8(24):12362–12372.
- Waters R, Pacelli S, Maloney R, et al. Stem cell secretome-rich nanoclay hydrogel: a dual action therapy for cardiovascular regeneration. Nanoscale. 2016;8(14):7371–7376.
- Haraguchi K, Takehisa T. Nanocomposite hydrogels: a unique organic–inorganic network structure with extraordinary mechanical, optical, and swelling/De-swelling properties. Adv Mater. 2002;14(16):1120–1124.
- Haraguchi K, Li H-J. Mechanical properties and structure of polymer − clay nanocomposite gels with high clay content. Macromolecules. 2006;39(5):1898–1905.
- Bouville F, Maire E, Meille S, et al. Strong, tough and stiff bioinspired ceramics from brittle constituents. Nat Mater. 2014;13(5):508–514.
- Juhasz JA, Best SM, Bonfield W. Preparation of novel bioactive nano-calcium phosphate-hydrogel composites. Sci Technol Adv Mater. 2010;11(1):014103.
- Gibbs DMR, Black CRM, Dawson JI, et al. A review of hydrogel use in fracture healing and bone regeneration. J Tissue Eng Regen Med. 2016;10(3):187–198.
- Agarwal R, García AJ. Biomaterial strategies for engineering implants for enhanced osseointegration and bone repair. Adv Drug Deliv Rev. 2015;94:53–62.
- Ebrahimi M, Botelho M, Lu W, et al. Synthesis and characterization of biomimetic bioceramic nanoparticles with optimized physicochemical properties for bone tissue engineering. J Biomed Mater Res A. 2019;107(8):1654–1666.
- Liu F-H. Synthesis of bioceramic scaffolds for bone tissue engineering by rapid prototyping technique. J Sol-Gel Sci Technol. 2012;64: 704–710. doi:10.1007/s10971-012-2905-5
- Samavedi S, Whittington AR, Goldstein AS. Calcium phosphate ceramics in bone tissue engineering: a review of properties and their influence on cell behavior. Acta Biomater. 2013;9(9):8037–8045.
- Fox K, Tran PA, Tran N. Recent advances in research applications of nanophase hydroxyapatite. Chemphyschem. 2012;13(10):2495–2506.
- Swetha M, Sahithi K, Moorthi A, et al. Biocomposites containing natural polymers and hydroxyapatite for bone tissue engineering. Int J Biol Macromol. 2010;47(1):1–4.
- Pathi SP, Lin DDW, Dorvee JR, et al. Hydroxyapatite nanoparticle-containing scaffolds for the study of breast cancer bone metastasis. Biomaterials. 2011;32(22):5112–5122.
- Giordano C, Causa F, Silvio LD, et al. Chemical-physical and preliminary biological properties of poly (2-hydroxyethylmethacrylate)/poly-(epsilon-caprolactone)/hydroxyapa- tite composite. J Mater Sci Mater Med. 2007;18(4):653–660.
- Li Z, Mi W, Wang H, et al. Nano-hydroxyapatite/polyacrylamide composite hydrogels with high mechanical strengths and cell adhesion properties. Colloids Surf B Biointerfaces. 2014;123:959–964.
- Hu K, Zhou N, Li Y, et al. Sliced magnetic polyacrylamide hydrogel with Cell-Adhesive microarray interface: a novel multicellular spheroid culturing platform. ACS Appl Mater Interfaces. 2016;8(24):15113–15119.
- Thakur T, Xavier JR, Cross L, et al. Photocrosslinkable and elastomeric hydrogels for bone regeneration. J Biomed Mater Res A. 2016;104(4):879–888.
- Shi D, Xu X, Ye Y, et al. Photo-cross-linked scaffold with Kartogenin-encapsulated nanoparticles for cartilage regeneration. ACS Nano. 2016;10(1):1292–1299.
- Moon HJ, Patel M, Chung H, et al. Nanocomposite versus mesocomposite for osteogenic differentiation of Tonsil-Derived mesenchymal stem cells. Adv Healthc Mater. 2016;5(3):353–363.
- Mahon OR, Browe DC, Gonzalez-Fernandez T, et al. Nano-particle mediated M2 macrophage polarization enhances bone formation and MSC osteogenesis in an IL-10 dependent manner. Biomaterials. 2020;239:119833.
- Song Y, Wu H, Gao Y, et al. Zinc silicate/nano-hydroxyapatite/collagen scaffolds promote angiogenesis and bone regeneration via the p38 MAPK pathway in activated monocytes. ACS Appl Mater Interfaces. 2020;12(14):16058–16075.
- Yang C, Zhao C, Wang X, et al. Stimulation of osteogenesis and angiogenesis by micro/nano hierarchical hydroxyapatite via macrophage immunomodulation. Nanoscale. 2019;11(38):17699–17708.
- Chen Y, Kawazoe N, Chen G. Preparation of dexamethasone-loaded biphasic calcium phosphate nanoparticles/collagen porous composite scaffolds for bone tissue engineering. Acta Biomater. 2018;67:341–353.
- Yu S, Shi J, Liu Y, et al. A mechanically robust and flexible PEGylated poly(glycerol sebacate)/β-TCP nanoparticle composite membrane for guided bone regeneration. J Mater Chem B. 2019;7(20):3279–3290.
- Tohma Y, Tanaka Y, Ohgushi H, et al. Early bone in-growth ability of alumina ceramic implants loaded with tissue-engineered bone. J Orthop Res. 2006;24(4):595–603.
- Chen J, Li M, Yang C, et al. Macrophage phenotype switch by sequential action of immunomodulatory cytokines from hydrogel layers on titania nanotubes. Colloids Surf B Biointerfaces. 2018;163:336–345.
- Mota J, Yu N, Caridade SG, et al. Chitosan/bioactive glass nanoparticle composite membranes for periodontal regeneration. Acta Biomater. 2012;8(11):4173–4180.
- Luz GM, Mano JF. Chitosan/bioactive glass nanoparticles composites for biomedical applications. Biomed Mater. 2012;7(5):054104.
- El-Rashidy AA, Roether JA, Harhaus L, et al. Regenerating bone with bioactive glass scaffolds: a review of in vivo studies in bone defect models. Acta Biomater. 2017;62:1–28.
- Multanen V, Bhushan B. Bioinspired self-healing, superliquiphobic and self-cleaning hydrogel-coated surfaces with high durability. Philos Trans A Math Phys Eng Sci. 2019;377(2150):20190117.
- Chaudhari NS, Pandey AP, Patil PO, et al. Graphene oxide based magnetic nanocomposites for efficient treatment of breast cancer. Mater Sci Eng C Mater Biol Appl. 2014;37:278–285.
- Lamichhane N, Sharma S, null P, et al. Iron oxide-based magneto-optical nanocomposites for in vivo biomedical applications. Biomedicines. 2021;9:288.
- Pan W-Y, Huang C-C, Lin T-T, et al. Synergistic antibacterial effects of localized heat and oxidative stress caused by hydroxyl radicals mediated by graphene/iron oxide-based nanocomposites. Nanomedicine. 2016;12(2):431–438.
- Bock N, Riminucci A, Dionigi C, et al. A novel route in bone tissue engineering: magnetic biomimetic scaffolds. Acta Biomater. 2010;6(3):786–796.
- D'Amora U, Russo T, Gloria A, et al. 3D additive-manufactured nanocomposite magnetic scaffolds: Effect of the application mode of a time-dependent magnetic field on hMSCs behavior. Bioact Mater. 2017;2(3):138–145.
- Noriega-Luna B, Sabanero M, Sosa M, et al. Influence of pulsed magnetic fields on the morphology of bone cells in early stages of growth. Micron. 2011;42(6):600–607.
- Ba X, Hadjiargyrou M, DiMasi E, et al. The role of moderate static magnetic fields on biomineralization of osteoblasts on sulfonated polystyrene films. Biomaterials. 2011;32(31):7831–7838.
- Ross SM. Combined DC and ELF magnetic fields can alter cell proliferation. Bioelectromagnetics. 1990;11(1):27–36.
- Hashimoto Y, Kawasumi M, Saito M. Effect of static magnetic field on cell migration. Elect Eng Jpn. 2007;160(2):46–52.
- Kotani H, Kawaguchi H, Shimoaka T, et al. Strong static magnetic field stimulates bone formation to a definite orientation in vitro and in vivo. J Bone Miner Res. 2002;17(10):1814–1821.
- Chen Z, Yin J-J, Zhou Y-T, et al. Dual enzyme-like activities of iron oxide nanoparticles and their implication for diminishing cytotoxicity. ACS Nano. 2012;6(5):4001–4012.
- Huang D-M, Hsiao J-K, Chen Y-C, et al. The promotion of human mesenchymal stem cell proliferation by superparamagnetic iron oxide nanoparticles. Biomaterials. 2009;30(22):3645–3651.
- Jaiswal MK, Xavier JR, Carrow JK, et al. Mechanically stiff nanocomposite hydrogels at ultralow nanoparticle content. ACS Nano. 2016;10(1):246–256.
- Wong SHD, Wong WKR, Lai CHN, et al. Soft polymeric matrix as a macroscopic cage for magnetically modulating reversible nanoscale ligand presentation. Nano Lett. 2020;20(5):3207–3216.
- Choi JR, Yong KW, Choi JY, et al. Black phosphorus and its biomedical applications. Theranostics. 2018;8(4):1005–1026.
- Bala Y, Farlay D, Boivin G. Bone mineralization: from tissue to crystal in normal and pathological contexts. Osteoporos Int. 2013;24(8):2153–2166.
- Matsuoka K, Park K-A, Ito M, et al. Osteoclast-derived complement component 3a stimulates osteoblast differentiation. J Bone Miner Res. 2014;29(7):1522–1530.
- Gaharwar AK, Cross LM, Peak CW, et al. 2D nanoclay for biomedical applications: Regenerative medicine, therapeutic delivery, and additive manufacturing. Adv Mater. 2019;31(23):e1900332.
- Huang K, Wu J, Gu Z. Black phosphorus hydrogel scaffolds enhance bone regeneration via a sustained supply of Calcium-Free phosphorus. ACS Appl Mater Interfaces. 2019;11(3):2908–2916.
- Gao D, Guo X, Zhang X, et al. Multifunctional phototheranostic nanomedicine for cancer imaging and treatment. Mater Today Bio. 2020;5:100035.
- Chen W, Ouyang J, Liu H, et al. Black phosphorus nanosheet-based drug delivery system for synergistic photodynamic/photothermal/chemotherapy of cancer. Adv Mater Deerfield Beach Fla. 2017;29.
- Sun Z, Xie H, Tang S, et al. Ultrasmall black phosphorus quantum dots: Synthesis and use as photothermal agents. Angew Chem Int Ed Engl. 2015;54(39):11526–11530.
- Shui C, Scutt A. Mild heat shock induces proliferation, alkaline phosphatase activity, and mineralization in human bone marrow stromal cells and Mg-63 cells in vitro. J Bone Miner Res. 2001;16(4):731–741.
- Nørgaard R, Kassem M, Rattan SIS. Heat shock-induced enhancement of osteoblastic differentiation of hTERT-immortalized mesenchymal stem cells. Ann N Y Acad Sci. 2006;1067:443–447.
- Pan W, Dai C, Li Y, et al. PRP-chitosan thermoresponsive hydrogel combined with black phosphorus nanosheets as injectable biomaterial for biotherapy and phototherapy treatment of rheumatoid arthritis. Biomaterials. 2020;239:119851.
- Singh MN, Hemant KSY, Ram M, et al. Microencapsulation: a promising technique for controlled drug delivery. Res Pharm Sci. 2010;5(2):65–77.
- Wang X, Shao J, Abd El Raouf M, et al. Near-infrared light-triggered drug delivery system based on black phosphorus for in vivo bone regeneration. Biomaterials. 2018;179:164–174.
- Cha C, Shin SR, Annabi N, et al. Carbon-Based nanomaterials: multifunctional materials for biomedical engineering . ACS Nano. 2013;7(4):2891–2897.
- Shin SR, Bae H, Cha JM, et al. Carbon nanotube reinforced hybrid microgels as scaffold materials for cell encapsulation. ACS Nano. 2012;6(1):362–372.
- Motealleh A, Kehr NS. Nanocomposite hydrogels and their applications in tissue engineering. Adv Healthcare Mater. 2017;6(1):1600938.
- Samuel ELG, Duong MT, Bitner BR, et al. Hydrophilic carbon clusters as therapeutic, high-capacity antioxidants. Trends Biotechnol. 2014;32(10):501–505.
- Yang S-T, Luo J, Zhou Q, et al. Pharmacokinetics, metabolism and toxicity of carbon nanotubes for biomedical purposes. Theranostics. 2012;2(3):271–282.
- Firme CP, Bandaru PR. Toxicity issues in the application of carbon nanotubes to biological systems. Nanomedicine. 2010;6(2):245–256.
- Cheng X, Wan Q, Pei X. Graphene family materials in bone tissue regeneration: Perspectives and challenges. Nanoscale Res Lett. 2018;13(1):289.
- Nayak TR, Andersen H, Makam VS, et al. Graphene for controlled and accelerated osteogenic differentiation of human mesenchymal stem cells. ACS Nano. 2011;5(6):4670–4678.
- Dong R, Zhao X, Guo B, et al. Self-Healing conductive injectable hydrogels with antibacterial activity as cell delivery carrier for cardiac cell therapy. ACS Appl Mater Interfaces. 2016;8(27):17138–17150.
- Akhavan O, Ghaderi E. Toxicity of graphene and graphene oxide nanowalls against bacteria. ACS Nano. 2010;4(10):5731–5736.
- Li J, Wang G, Zhu H, et al. Antibacterial activity of large-area monolayer graphene film manipulated by charge transfer. Sci Rep. 2014;4:4359.
- Han L, Sun H, Tang P, et al. Mussel-inspired graphene oxide nanosheet-enwrapped Ti scaffolds with drug-encapsulated gelatin microspheres for bone regeneration. Biomater Sci. 2018;6(3):538–549.
- Choe G, Oh S, Seok JM, et al. Graphene oxide/alginate composites as novel bioinks for three-dimensional mesenchymal stem cell printing and bone regeneration applications. Nanoscale. 2019;11(48):23275–23285.
- Shen H, Lin H, Sun AX, et al. Acceleration of chondrogenic differentiation of human mesenchymal stem cells by sustained growth factor release in 3D graphene oxide incorporated hydrogels. Acta Biomater. 2020;105:44–55.
- Qi C, Deng Y, Xu L, et al. A sericin/ graphene oxide composite scaffold as a biomimetic extracellular matrix for structural and functional repair of calvarial bone. Theranostics. 2020;10(2):741–756.
- Rui-Hong X, Peng-Gang R, Jian H, et al. Preparation and properties of graphene oxide-regenerated cellulose/polyvinyl alcohol hydrogel with pH-sensitive behavior. Carbohydr Polym. 2016;138:222–228.
- Christy PN, Basha SK, Kumari VS, et al. Biopolymeric nanocomposite scaffolds for bone tissue engineering applications – a review. J Drug Deliv Sci Technol. 2020;55:101452.
- Jodati H, Yılmaz B, Evis Z. A review of bioceramic porous scaffolds for hard tissue applications: Effects of structural features. Ceram Int. 2020;46(10):15725–15739.
- Barradas AMC, Yuan H, van Blitterswijk CA, et al. Osteoinductive biomaterials: current knowledge of properties, experimental models and biological mechanisms. Eur Cell Mater. 2011;21:407–429; discussion 429.
- Wubneh A, Tsekoura EK, Ayranci C, et al. Current state of fabrication technologies and materials for bone tissue engineering. Acta Biomater. 2018;80:1–30.
- Schinagl RM, Gurskis D, Chen AC, et al. Depth-dependent confined compression modulus of full-thickness bovine articular cartilage. J Orthop Res. 1997;15(4):499–506.
- Yang J, Gao H, Zhang D, et al. Static compressive behavior and material failure mechanism of trabecular tantalum scaffolds fabricated by laser powder bed fusion-based additive manufacturing. Int J Bioprint. 2022;8(1):438.
- Chimene D, Lennox KK, Kaunas RR, et al. Advanced bioinks for 3D printing: a materials science perspective. Ann Biomed Eng. 2016;44(6):2090–2102.
- Jin Y, Liu C, Chai W, et al. Self-Supporting nanoclay as internal scaffold material for direct printing of soft hydrogel composite structures in air. ACS Appl Mater Interfaces. 2017;9(20):17456–17465.
- Ahlfeld T, Cidonio G, Kilian D, et al. Development of a clay based bioink for 3D cell printing for skeletal application. Biofabrication. 2017;9(3):034103.
- Byambaa B, Annabi N, Yue K, et al. Bioprinted osteogenic and vasculogenic patterns for engineering 3D bone tissue. Adv Healthcare Mater. 2017;6(16):1700015.
- Gao F, Xu Z, Liang Q, et al. Direct 3D printing of high strength biohybrid gradient hydrogel scaffolds for efficient repair of osteochondral defect. Adv Funct Mater. 2018;28(13):1706644.
- Barros J, Ferraz MP, Azeredo J, et al. Alginate-nanohydroxyapatite hydrogel system: Optimizing the formulation for enhanced bone regeneration. Mater Sci Eng C Mater Biol Appl. 2019;105:109985.
- Roohaniesfahani I, Wang J, No YJ, et al. Modulatory effect of simultaneously released magnesium, strontium, and silicon ions on injectable silk hydrogels for bone regeneration. Mater Sci Eng C Mater Biol Appl. 2019;94:976–987.
- Kwon S, Lee SS, Sivashanmugam A, et al. Bioglass-Incorporated methacrylated gelatin cryogel for regeneration of bone defects. Polymers. 2018;10(8):914.
- Mahdavinia GR, Etemadi H, Soleymani F. Magnetic/pH-responsive beads based on caboxymethyl chitosan and κ-carrageenan and controlled drug release. Carbohydr Polym. 2015;128:112–121.
- Madani SZM, Reisch A, Roxbury D, et al. A magnetically responsive hydrogel system for controlling the timing of bone progenitor recruitment and differentiation factor deliveries. ACS Biomater Sci Eng. 2020;6(3):1522–1534.
- Huang J, Jia Z, Liang Y, et al. Pulse electromagnetic fields enhance the repair of rabbit articular cartilage defects with magnetic nano-hydrogel. RSC Adv. 2019;10(1):541–550.
- Yang G, Wan X, Gu Z, et al. Near infrared photothermal-responsive poly(vinyl alcohol)/black phosphorus composite hydrogels with excellent on-demand drug release capacity. J Mater Chem B. 2018;6(11):1622–1632.