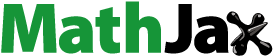
Abstract
In this investigation, we employed chemical oxidative copolymerization techniques to produce cross-linked polyaniline/chitosan-graphene oxide-oxidized single-wall carbon nanotubes (Cross PANI/Chito-GO-OXS NCs) as a network to increase the surface area, as well as the active centers to enhance the efficiency of the adsorption of anionic and cationic dyes. This novel material was utilized as an adsorbent for decontaminating water by eliminating acid red dye (AR1) and brilliant green dye (BG). Several analytical techniques were employed to assess the composition and structure of the synthesized adsorbent, including scanning electron microscopy (SEM), high-resolution transmission electron microscopy (HR-TEM), X-ray powder diffraction (XRD), infrared spectroscopy (FT-IR), thermogravimetric analysis (TGA), and Raman spectroscopy. To assess the efficacy of Cross PANI/Chito-GO-OXS NCs in dye removal, batch experiments were carried out under diverse conditions, such as varying pH, adsorbent quantity, agitation duration, ionic strength, and temperature. The results indicated that the optimum conditions for AR1 removal were achieved at pH 2, using 5 mg of Cross PANI/Chito-GO-OXS NCs at a concentration of 20 mg/L for 120 min. Conversely, for BG removal, the most favorable outcomes were observed at pH 6, with the use of 5 mg of Cross PANI/Chito-GO-OXS NCs at a concentration of 5 mg/L for 120 min. Under these operating conditions, the maximum removal efficiency for actual wastewater was determined to be 96.73% for AR1 dye and 95.55% for BG dye. Additionally, we analyzed the adsorption isotherm data utilizing various models. The adsorption kinetics were elucidated through a pseudo-second-order model, supported by regression data, which yielded R2 values of 0.9839 for AR1 and 0.9928 for BG, respectively, describing the adsorption of both dyes onto Cross PANI/Chito-GO-OXS NCs. Furthermore, the thermodynamic analysis revealed that the removal of AR1 dye is an endothermic process, while BG dye removal is exothermic, and both removal of AR1 and BG dyes are spontaneous and chemisorption processes.
1. Introduction
Water pollution is a pressing global concern, primarily driven by a wide range of industrial effluents, including those originating from industries such as paint, food, printing, pharmaceuticals, and textiles. These industrial waste streams contain hazardous pollutants that have previously caused numerous health and environmental problems. The reason for the widespread presence of these inorganic pollutants in groundwater is their propensity to accumulate in living organisms [Citation1]. Textile industry activities alone contribute to 10–15% of toxic dye contamination [Citation2]. Verities of dyes can be divided into groups such as azo dyes, acidic, basic, reactive, dispersed, and metal complex dyes. Dyes, especially present in industrial effluents, pose a considerable threat because of their hazardous nature. Their effluents can have adverse effects on human health, plant species, aquatic life, and overall environmental equilibrium [Citation3–5]. In this research, model dyes AR1 and BG were chosen for investigation. AR1 dyes are complex chemical compounds containing azo linkages and aromatic rings commonly used for coloring wool, silk, and leather. AR1 dye is toxic to humans and aquatic organisms, with bactericidal and mutagenic properties [Citation6,Citation7]. On the other hand, BG dye belongs to a group of compounds used in various applications, such as biological stains, dermatological agents, veterinary medications, and mold and intestinal issue inhibitors. When the BG dye contacts skin, it will cause dermatitis with pain and redness. It may be also cause gastrointestinal tract irritation [Citation7,Citation8]. To address wastewater contamination, several methods are available, including coagulation, chlorination, electrocoagulation, flotation, ozonation, filtration, chemical oxidation, adsorption, membrane separation, and ultrafiltration [Citation9,Citation10]. In adsorption, new adsorbents with high properties, such as high surface area, high capacity of adsorption, and low cost are the focus areas of many researchers. Numerous adsorbents, such as zeolite, clay, fly ash, etc., have been used successfully for environmental treatment at low costs. On the other hand, the removal of dyes by some of these adsorbents is effective, but most of these adsorbents have low surface area with a limited number of active sides and destitute kinetic adsorption. Recently, many nano adsorbents have been worth development in the field of adsorption [Citation11,Citation12].
Polyaniline (PANI), a conductive polymer, has gained attention as a potential adsorbent for removing water pollutants. Its unique electrical properties, ease of synthesis, cost-effective monomers, environmental stability, and controllable reversible characteristics make it a promising choice. PANI also possesses functional groups like NH-, further enhancing its suitability as an adsorbent. PANI-based nanocomposites, known for their high surface areas, excellent dispersibility, and synergistic effects, have been widely studied for pollutant adsorption. Because of these considerations, the applications of PANI have focused on solving many environmental problems [Citation13,Citation14].
Chitosan (Cs) is another material with remarkable properties, making it an excellent option for water purification and extraction [Citation15]. Cs possess several advantageous characteristics: biocompatibility, biodegradability, non-toxicity, abundance, bioactivity, and good adsorption capacities are only a few benefits. It is a D-glucosamine and N-acetyl-D-glucosamine copolymer connected by −1-4-glycosidic links [Citation16]. The hydrophilicity, biocompatibility, biodegradability, antibacterial activity, and low cytotoxicity are among the many attributes that make Cs highly valuable [Citation17]. Because of the amino and hydroxyl groups, Cs can be used as an adsorbent to effectively remove cationic and/or anionic dyes, providing active sites for adsorption [Citation18,Citation19].
Recent research has focused on hybrid materials that include graphene oxide (GO) and/or oxidized carbon nanotubes (OXS) because of their high theoretical specific surface areas and demonstrated effectiveness in removing dyes and organic contaminants [Citation20,Citation21]. The oxygen functional groups and aromatic sp2 regions in GO and/or OXS facilitate strong bonding with other materials [Citation22–26].
This study aimed to prepare crosslinked (PANI/Chito-GO-OXS) NCs through oxidative polymerization and evaluate their efficiency in removing anionic dye AR1 and cationic dye BG from both aqueous solutions as well as real samples of water collected from different locations. The synthesis of NCs resulted in a network structure that increased the surface area and active sites within the NCs, enhancing their efficacy in dye removal. Various analytical techniques, including XRD, FTIR, Raman spectroscopy, SEM, TEM, and thermal analyses, were used to characterize the NCs. The adsorption process was investigated through thermodynamic and kinetic experiments. Finally, AR1 and BG dyes were used to assess their removal from real water samples.
2. Materials and procedures
2.1. Materials
Aniline (ANI) at a purity of 99.50% was sourced from Shanghai Chemical Reagent Co. in China. Ammonium persulfate (APS) was provided by Across Organics in Belgium. p-Phenylenediamine (PPDA) with a purity of 98.00% was obtained from PDH in the UK without the need for additional purification. Triphenylamine (TPA), with a purity of 95.00%, was supplied by Janssen Chimica in Belgium. Graphene and single-walled carbon nanotubes (SWCNTs) were procured from XFNANOA Advanced Materials Supplier Inc. in China. Low molecular weight chitosan was sourced from Sigma Aldrich in the USA, along with sulfuric acid (H2SO4) at a purity of 99.99%, brilliant green, and acid red dyes. During the experiments, ultrapure, distilled, and deionized water (DI water) were used. Additionally, tap water from the chemical laboratory, sewage water from Umm-Alqura University in Makkah, Saudi Arabia, and Red Sea waters in Jeddah, Saudi Arabia, were employed for specific purposes. All additional compounds included in the study were of analytical grade and were not subjected to subsequent purification processes. Stock solutions of both dye BG and AR1 were prepared at a concentration of 500 mg/L, and the solutions were diluted to generate standard solutions with concentrations that varied between 5 and 50 mg/L. Distilled water was used for the purpose of dilution. A range of Britton-Robinson (BR) buffers with pH values spanning from 2 to 10, the extraction medium HCl (0.1 mol/L) used in this study served for the solid-phase adsorption of dyes.
2.2. Instrumentation
X-ray diffraction (XRD) on a Bruker D8 instrument was used to assess the crystallinity of the samples. Scanning electron microscopy (SEM) on a Thermo Fisher Quanta 250 FEG system and high-resolution transmission electron microscopy (HR-TEM) on a JEOL JEM-2100 apparatus were used to examine the morphologies of the materials created. The thermogravimetric analysis (TGA) and differential thermal gravimetry (DTG) were used to assess the thermal stability of the NC materials. The study was carried out using a TGA-50 system with a heating rate of 10 °C/min in the air to standardize their degradation temperature. To detect functional groups in the samples, Fourier-transform infrared spectroscopy (FTIR) with JASCO equipment was employed. Raman spectroscopy was performed with a Lab RAM-HR (Evolution Horiba Co.) The spectrophotometer (UV–visible) model Lambda 25 from Perkin-Elmer, which has a quartz cell with a width of 10 mm, was used to take the measurements. For pH measurements and solution testing, an Orion Co. model EA 940 pH meter was used. A micropipette from Volac Co. was utilized to make standard dye solutions, and a digital intelligent balance with four decimal digits from Citizen Scales Co., USA, was used for precise weighing. Finally, for various applications, a mechanical shaker from Precision Scientific (USA) with a vibration rate ranging from 10 to 250 rpm was used.
2.3. Preparation of the Chito-GO-OXS NCs
A modified Hummers’ approach [Citation27] was used to prepare GO. OXS was synthesized using a previously disclosed method. To make GO-OXS, a 1:1 mass ratio of OXS powder was mixed with a 25 mL deionized water GO dispersion. The mixture was then sonicated for 2 h in an ultrasonic instrument. The resulting dispersion had been dried for 24 h at 100 °C. To make the Chito-GO-OXS NCs composite [Citation26] and to create a homogenous solution, 0.5 g of Chitosan (Chito) was dissolved in 15 mL of a 1% acetic acid aqueous solution at 30 °C with stirring. The synthesized GO-OXS was then dispersed in deionized water (100 mL) in a bath with ultrasound for 1 h at room temperature. The solution of Chito was included in the GO-OXS mixture and sonicated for 2 h with the addition of 0.1 mL triethylamine. Finally, the Chito-GO-OXS composite was dried at 80 °C for 12 h.
2.4. Preparation of crosslinked (Cross PANI/Chito-GO-OXS) NCs
In the beginning, a three-neck flask with a volume of 250 mL was filled with 5% Chito-GO-OXS and 0.5 M H2SO4 (120 mL). Ultrasonication was used on the mixture at the ambient temperature for a period of 2 h. The flask was then filled with 1.8626 mL of twice distilled ANI, 0.043256 gm of P-phenylene diamine (PPDA), and 0.049064 gm of triphenylamine (TPA). For another 30 min, the mixture was ultrasonically treated. The solution was then refrigerated in an ice bath and constantly stirred at 0–4 °C. A solution containing 5 g of ammonium persulfate (APS) that had been precooled was dissolved in 40 mL of 0.5 M H2SO4. This solution was then added slowly and incrementally to the aforementioned solution over a duration of 30 min. At 0–4 °C, the process of adding occurred in a nitrogen (N2) environment with constant stirring. The polymerization technique was conducted in an N2 atmosphere at 0–4 °C with continual stirring for 24 h. The precipitate that developed was collected and rinsed with deionized water repeatedly until it turned colorless. Ultimately, the fine powder generated with a black color was dried for 24 h at 60 °C. PANI nanoparticles that have been crosslinked are known as “Cross PANI/Chito-GO-OXS NCs.” The same method was used to create Cross PANI/GO-OXS nanoparticles as well as pure Cross PANI nanoparticles but without the presence of Chito and Chito-GO-OXS, respectively [Citation28].
2.5. Batch removal step
The objective of this research was to investigate the impact of the Cross PANI/GO-OXS NCs adsorbent on the removal of hazardous organic dyes, specifically, AR 1 and BGA specific quantity of Cross PANI/Chito-GO-OXS NCs (0.005 ± 0.0003 g) was introduced into a conical flask containing 25 mL of an aqueous solution including AR1 dye (20 mg/L) and 0.1 M of HCl. At pH = 6, cross PANI/Chito-GO-OXS NCs (0.005 ± 0.0003 g) were accurately added to an aqueous solution (25 mL) containing dye of BG (5 mg/L). The solutions were then shaken for 120 min. After reaching equilibrium, the aqueous solution was subjected to separation, and the quantity of remaining dyes was assessed using a UV–Vis spectrophotometer by measuring dye absorbance before and subsequent to adsorption. Finally, Equationequation (1)(1)
(1) expresses the fraction of separation efficiency (%E). EquationEquation (2)
(2)
(2) is then used to express the amount of dye adsorbed (qt) on Cross PANI/Chito-GO-OXS NCs.
(1)
(1)
(2)
(2)
Where Co denotes the dye concentration, Ct denotes the solution dye concentration following shaking.
3. Rustle and dissociation
3.1. Surface morphology
A thorough analysis was conducted on the surface morphology of the synthesized Cross-linked PANI/Chito-GO-OXS NCs to get a comprehensive understanding of the interaction between the Chito-GO-OXS hybrid and the chemical and physical characteristics of the NCs. shows SEM images of pure Cross PANI and its NCs. The SEM image of pure Cross PANI ( displays a nanorod-like structure. In , the SEM image of Cross PANI/GO-OXS NCs reveals that GO-OXS serves as a framework during the in-situ polymerization procedure, leading to the uniform growth of Cross PANI nanorods on the surface of GO-OXS. This phenomenon results from the interaction between Cross PANI and GO-OXS, involving electrostatic forces, electron interactions, and hydrogen bonding; the outcome of this process is the formation of a nanorod core-shell structure that exhibits excellent alignment [Citation26]. However, SEM images of the Cross are depicted in . PANI/Chito-GO-OXS NCs demonstrate that the incorporation of the Chito-GO-OXS hybrid into Cross PANI significantly affects its morphology compared to the incorporation of GO-OXS alone without Chito. The previously observed well-aligned nanorod core-shell structure of Cross PANI/GO-OXS NCs has now transformed into a uniform planar morphology resembling plate-like grains. Similar to the behavior of GO-OXS, the Chito-GO-OXS hybrid serves as a templates during the preparation process, impacting the overall morphology of the Cross PANI, leading to the observed changes in the structure.
Figure 1. SEM images of Cross PANI (a), Cross PANI/GO-OXS NCs (b), Cross PANI/Chito-GO OXS NCs (c) NCs, and EDX of Cross PANI/Chito-GO OXS NCs (d).
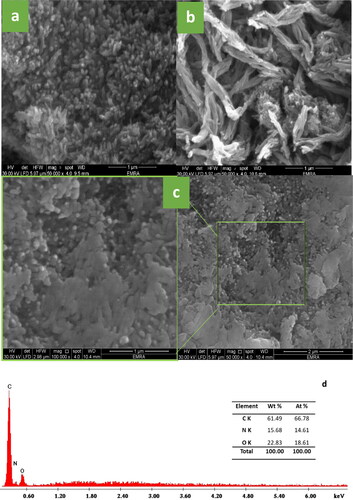
The EDX analysis ( of the Cross PANI/Chito-GO-OXS NCs provides evidence for the existence of peaks corresponding to C, N, and O. This validates the presence of PANI and Chito-GO-OXS inside the system.
The addition of the Chito-GO-OXS hybrid to Cross PANI introduces a distinct interconnected structure with larger network surfaces. When used as electrode materials, these materials can significantly enhance electrical conductivity. Chito plays a crucial role in forming a complex Cross PANI matrix. Cross PANI/Chito-GO-OXS NCs exhibit a plate-like shape, contributing to their improved electrical conductivity and electrochemical properties [Citation26,Citation29]. Further investigation of the microstructure of Cross PANI and its NCs experimentation with TEM. displays images of TEM of Cross PANI and Cross PANI/GO-OXS NCs, showing a regular nanorod morphology. However, the inclusion of Chito in the Chito-GO-OXS hybrid transforms the typical nanorod shape into a plate-like structure with a distinct core-shell architecture, as seen in . This image clearly reveals a discernible Chito-GO-OXS core (with darker contrast) surrounded by Cross PANI shells (with lighter contrast), demonstrating the successful coating of a thin polymer layer on the surface of Chito-GO-OXS. Additionally, no graphene oxide (GO) was detected in these NCs, indicating that the GO was entirely covered by Chito-GO-OXS, establishing the core-shell structure. The fabrication process of the Cross PANI/Chito-GO-OXS NCs is illustrated in Scheme 1. In summary, the incorporation of a Chito-GO-OXS hybrid into Cross PANI results in a plate-like morphology with a distinct core-shell structure.
3.2. Chemical structure
The crystalline phase of the produced materials was analyzed using XRD. The XRD diffraction patterns of Cross PANI and its NCs are presented in . For pure Cross PANI, two distinct peaks are observed at approximately 2θ = 20° and 25°, corresponding to the characteristic XRD peaks of Cross PANI, in line with prior studies that have reported these two diffraction peaks for Cross PANI [Citation29,Citation30]. When GO-OXS and Chito-GO-OXS are incorporated into the matrix of Cross PANI, the XRD spectra of the NCs exhibit diffraction peaks that are indistinguishable from those seen in pure Cross PANI, as well as peaks corresponding to GO-OXS and Chito-GO-OXS. The conspicuous crystalline peak at around 26° in both NCs, overlapping with Cross PANI, results from the presence of both GO-OXS and indicates increased long-range conjugation and interchain stacking [Citation31]. In both NCs, this peak signifies the establishment of the core-shell structure [Citation32]. Additional peaks appear at 21° and 24° in the case of Cross PANI/Chito-GO-OXS NCs, indicating the presence of Chito.
Figure 3. XRD patterns (a) and FTIR spectra (b) of Cross PANI, Cross PANI/GO-OXS, and Cross PANI/Chito-GO-OXS NCs; RAMAN spectra (c) and (d) isotherm curve of Cross PANI/Chito-GO-OXS NCs.
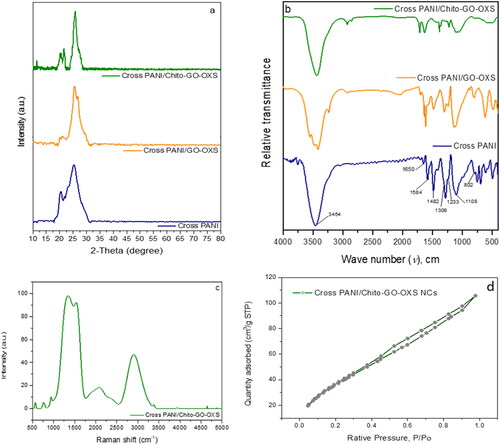
In , the FTIR spectra of Cross PANI and its NCs are depicted. The C = C stretching mode in rings of quinonoid and benzenoid units is responsible for the peaks in Cross PANI at 1582 and 1481 cm−1, respectively. The C-N stretching mode in units of aromatic amine gives rise to the peaks at 1307 and 1235 cm−1. The prominent signal at nearly 1108 cm−1 represents the doped structure. An absorbance peak at around 801 cm−1 is associated with the bending vibration out-of-plane C-H of 1,4-disubstituted rings. The clear peak that appears at 1652 cm−1 is induced by an increase in benzenoid content resulting from the copolymerization of TPA and PPDA and reflects the aromatic ring stretching vibration. The intense absorbance peak at 3456 cm−1 corresponds to the stretching mode of N-H in Cross PANI, and it becomes more pronounced with the addition of PPDA, indicating an increased amine concentration [Citation29]. In the FTIR spectra of both [Citation29] Cross PANI/GO-OXS NCs and Cross PANI/Chito-GO-OXS NCs, the intensities of the peaks are reduced compared to pure Cross PANI, suggesting interactions between Cross PANI chains and the surfaces of GO-OXS and Chito-GO-OXS, respectively. With the addition of GO-OXS, the peak at 1107 cm−1, which is characteristic of Cross PANI’s electrical conductivity, increases in intensity and slightly shifts, indicating an interaction between the conjugated structure of Cross PANI and the bonded structure of GO-OXS. In the case of Cross PANI/Chito-GO-OXS NCs, the strength of the peak at 1107 cm−1 decreases, signifying an interaction between Chito-GO-OXS and Cross PANI chains. The presence of Chito, which affects the electrical conductivity of the NCs, could explain the decrease in intensity. Based on the FTIR measurements, it can be concluded that both NCs were successfully synthesized.
In , Raman spectroscopy is utilized to demonstrate the presence of Chito-GO-OXS alongside Cross PANI. The GO-OXS Raman spectra show discrete peaks at 1345 and 1540 cm−1 [Citation33]. These findings indicate the presence of GO-OXS in the Cross PANI/Chito-GO-OXS NCs as prepared [Citation28,Citation29].
The N2 adsorption/desorption isotherms observed for Cross PANI/Chito-GO-OXS NCs were categorized as type IV isotherms in . Additionally, the specific surface area (BET) was determined to be 148.158 m2/g ().
Table 1. The SBET, total pore volumes, and pore size of Cross PANI/Chito-GO-OXS NCs.
3.3. Thermal analysis
illustrates the thermogravimetric analysis (TGA-DTG) of Cross PANI) and its NCs. As per previous research [Citation26], the TGA curve of pure Cross PANI, shown in , reveals three distinct weight loss phases. The first minor weight loss step (14.1 wt%) occurring between 50 and 150 °C is attributed to moisture evaporation. The second weight loss stage (5.4 wt%) between 150 and 250 °C is responsible for the elimination of doping anions and the rapid breakdown of PPDA throughout copolymerization crosslinking [Citation33]. The third weight loss stage (250–580 °C) is mostly caused by polymer chain breakup, which results in the total decomposition of Cross PANI at 583 °C.
Figure 4. TGA (a) and DTG (b) curves of Cross PANI, Cross PANI/GO-OXS, and Cross PANI/Chito-GO-OXS NCs, and (c) TGA, DTG and DTA curves of Cross PANI/Chito-GO-OXS NCs.
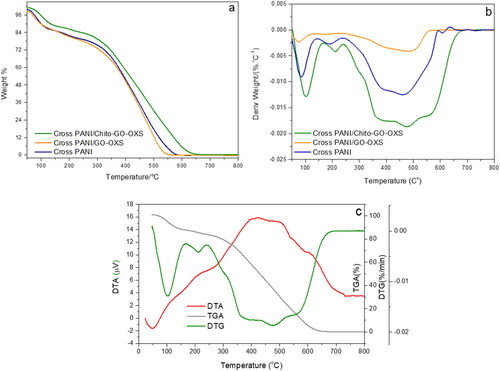
In , the NCs of Cross PANI, Cross PANI/GO-OXS, and Cross PANI/Chito-GO-OXS exhibit similar degradation behavior to pure Cross PANI. However, in the case of Cross PANI/GO-OXS NCs, the temperature of thermal degradation is slightly lower than that of pure Cross PANI, indicating reduced thermal stability after the incorporation of GO-OXS. Conversely, when Chito is hybridized with GO-OXS in Cross PANI/Chito-GO-OXS NCs, the temperature of thermal degradation is enhanced, leading to improved thermal stability, as evidenced by the shift to higher temperatures. provides temperatures (T10, T25, and T50) that correspond to 10%, 25%, and 50% weight losses during thermal degradation, respectively. Cross PANI/Chito-GO-OXS NCs exhibit higher T10, T25, and T50 values than other samples, indicating that Chito-GO-OXS has a significant impact on limiting the degradation of the Cross PANI matrix and enhancing its thermal stability. This increased stability can be attributed to the interactions between Cross PANI and Chito-GO-OXS, signifying that the presence of Chito in Chito-GO-OXS plays a role in improving thermal stability. Furthermore, Chito-GO-OXS is well coated by Cross PANI chains, further contributing to the enhanced thermal properties. The maximum polymer degradation temperature (PDTmax) [Citation34,Citation35] was determined using the DTG curves in , and it is somewhat higher in Cross PANI/GO-OXS NCs than in Cross PANI/Chito-GO-OXS NCs. Additionally, the composite degradation temperature (CDTfinal) [Citation27,Citation36] represents the final temperature at which decomposition is complete. The CDTfinal value of Cross PANI/Chito-GO-OXS NCs is greater compared to the values observed in other samples, indicating that the interaction between Cross PANI and Chito-GO-OXS increases thermal stability. In the DTA data, Cross PANI/Chito-GO-OXS NCs exhibit an endothermic peak at 160 °C (4.5 V) and two exothermic peaks at 216 °C (6.9 V) and 421 °C (16 V), as shown in . The largest change in mass corresponds to the peak at 421 °C. The exothermic maxima at 216 and 421 °C correspond to the first and second breakdown stages (150–240 °C and 240–585 °C, respectively). In summary, the TGA-DTG analyses demonstrate that the incorporation of Chito-GO-OXS enhances the thermal stability of Cross PANI/Chito-GO-OXS NCs, and the interactions between Cross PANI and Chito-GO-OXS significantly contribute to the enhancement of the composite material’s thermal characteristics.
Table 2. Thermal behavior of Cross PANI, Cross PANI/GO-OXS, and Cross PANI/Chito-GO-OXS NCs.
3.4. Adsorption studies
presents a comparative analysis of the effectiveness of two NCs, Cross PANI/GO-OXS and Cross PANI/Chito-GO-OXS; AR1 and BG dyes were removed from an aqueous phase. The capacity of both NCs to remove BG dye from the aqueous solution was evaluated. It was observed that Cross PANI/Chito-GO-OXS NCs exhibited superior performance in dye removal compared to Cross PANI/GO-OXS NCs. As a result, Cross PANI/Chito-GO-OXS NCs were chosen as the best NCs for all future experiments. In the aqueous solution phase, the electronic spectrum of AR1 and BG dyes indicated absorption maxima at 530 nm for AR1 and 624 nm for BG. However, after interaction with Cross PANI/Chito-GO-OXS NCs, these absorption peaks significantly decreased, as depicted in . This behavior confirms the high efficiency of Cross PANI/Chito-GO-OXS NCs in removing dyes from aqueous solutions. The significant reduction in the absorption peaks indicates that the dyes are effectively adsorbed by the NCs, leading to their removal from the aqueous phase. Overall, the comparative research shows that Cross PANI/Chito-GO-OXS NCs are very efficient in the elimination of dyes from aqueous solutions and are hence the best candidate for future dye removal investigations and applications.
Figure 5. Efficiency comparison of (a) Cross PANI/GO-OXS NCs and (b) Cross PANI/Chito-GO-OS NCs in removing AR1 and BG dyes from an aqueous phase.
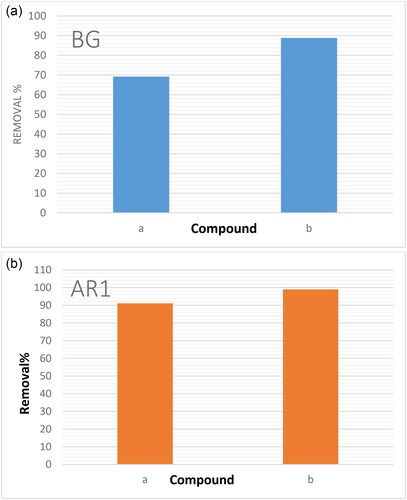
Figure 6. The electronic spectra of (A) Acid Red 1 dye (20 ppm) in the sample solution without Cross PANI/Chito-GO-OXS NCs, (B) after shaking in solution with Cross PANI/Chito-GO-OXS NCs (5 mg) (I), and (A) Brilliant Green dye (5 ppm) in the sample solution without Cross PANI/Chito-GO-OXS NCs (5 mg) and (B) after shaking in solution with Cross PANI/Chito-GO-OXS NCs (5 mg) (II).
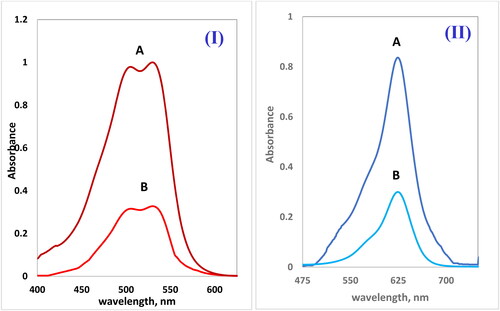
3.5. AR 1 and BG dyes retention profiles from aqueous solutions onto Cross PANI/Chito-GO-OXS NCs
3.5.1. The pH effect
The ionization state of both the adsorbent molecules and the adsorbate, as well as the surface properties of the adsorbent, are influenced by the pH of a solution during the adsorption process [Citation5,Citation37]. In this investigation, Cross PANI/Chito-GO-OXS NCs were used to remove AR1 and BG dyes at pH 2–10. The experimental conditions remained constant, with a contact time of 120 min, adsorbent doses of 5 mg for both AR1 and BG dyes, and initial dye concentrations of 20 mg/L for AR1 and 5 mg/L for BG. illustrates the effects of pH changes on dye removal. The highest percentage of AR1 dye adsorption was observed at severely acidic pH (pH = 2), and the removal efficiency decreased as the pH increased. In contrast, for BG dye, the removal efficiency increased with an increase in pH until reaching pH 6, after which it began to decrease. The optimal pH for the removal of AR1 dye using Cross PANI/Chito-GO-OXS NCs was found to be pH 2, while for BG dye, the optimal pH was pH 6. This result emphasizes the significance of pH in dye adsorption onto Cross PANI/Chito-GO-OXS NCs and provides valuable insights for optimizing the adsorption process. The findings indicate that adsorption behavior is highly dependent on solution pH, and altering the pH can have a significant impact on the efficiency of dye removal using these NCs. As a result of the low pH (pH 2), the surface of Cross PANI/Chito-GO-OXS NCs becomes highly protonated, which accelerates the rate at which anionic dye molecules are adsorbed. The efficient removal of dyes is facilitated by the strong electrostatic interaction between negatively charged dye molecules and the positively charged Cross PANI/Chito-GO-OXS NCs [Citation5]. Conversely, in a highly alkaline dye solution with a pH of 10, the surface of Cross PANI/Chito-GO-OXS NCs attracts hydroxyl ions because of the high concentration of OH- ions. This neutralizes the surface charges and hinders the adsorption of dye molecules, resulting in a very low rate of dye removal [Citation5]. On the other hand, the adsorption efficiency of cationic dye by Cross PANI/Chito-GO-OXS NCs decreases at low pH and increases as the pH value rises [Citation38].
3.5.2. Adsorbent dosage effect
The quantity of adsorbent used plays a crucial role in determining its capacity to adsorbate a given amount under specific operating conditions. This research examined dye adsorption at different Cross PANI/Chito-GO-OXS NC concentrations from 2.5 to 12.5 mg (as shown in ). The study maintained the following fixed conditions: a 120-min contact time, initial dye concentrations of 20 mg/L for AR1 and 5 mg/L for BG dyes, and pH values of 2 and 6 for AR1 and BG dyes, respectively. The results demonstrated that increasing the adsorbent dosage from 2.5 to 12.5 mg had a positive effect, leading to an increase in the amount of adsorbed AR1 dye from 60.5% to 99.7%. Similarly, for BG dye, increasing the adsorbent dosage from 2.5 to 12.5 mg resulted in an increase in the adsorbed amount from 52.0 to 98.4%. It is important to note that a solid-phase mass of 5 mg was selected for the removal of both AR1 and BG dyes, resulting in removal efficiencies of 65.7% and 75.7% for Cross PANI/Chito-GO-OXS NCs, respectively. This choice allowed us to investigate how different parameters affected the adsorption process.
3.5.3. Contact time effect
Determining the optimal contact duration represents a pivotal aspect in adsorption investigations as it elucidates the duration needed for the system to attain equilibrium. In this search, the adsorption of AR1 and BG dyes onto Cross PANI/Chito-GO-OXS NCs necessitated approximately 2 h to reach a state of full equilibrium, as illustrated in .The percentage and quantity of AR1 and BG dyes adhering to the surface of Cross PANI/Chito-GO-OXS NCs increased progressively over time, as indicated by the outcomes obtained within the prescribed conditions (contact time range: 5–120 min, initial concentrations of dye were 20 mg/L and 5 mg/L, and pH values of 2 and 6). The adsorption process exhibited two discernible phases: the initial phase (rapid adsorption) occurred during the initial 75 min, with AR1 dye exhibiting an adsorption percentage of 62.2% and BG dye at 50%. Subsequently, from 90 to 120 min, the process transitioned into the second phase of slower adsorption, with minimal alterations in the concentration of AR1 and BG dyes, ultimately resulting in an adsorption percentage of 75.0% for AR1 dye and 66% for BG dye.
3.5.4. Temperature effect
The effect of temperature on AR1 and BG dye adsorption was investigated using Cross PANI/Chito-GO-OXS at a range of temperatures from 285 K to 318 K. The experiment was conducted under controlled conditions, with a fixed contact time of 120 min, initial dye concentrations of 20 mg/L and 5 mg/L, and pH values of 2 and 6 for AR1 and BG dyes, respectively. illustrates the findings. The percentage of AR1 dye adsorbed onto Cross PANI/Chito-GO-OXS NCs increased with rising temperature, as depicted in . This phenomenon demonstrates that the adsorption process is endothermic, signifying that higher temperatures promote it. Conversely, as displayed in , elevating the solution temperature decreased the removal efficiency of BG dye from Cross PANI/Chito-GO-OXS NCs. This observation reveals that the adsorption process for BG dye is exothermic, implying that lower temperatures are more favorable for adsorption. To summarize, temperature exerts a substantial influence on AR1 and BG dye adsorption on Cross PANI/Chito-GO-OXS NCs, with AR1 dye exhibiting enhanced adsorption at higher temperatures (an endothermic process) and BG dye demonstrating reduced removal efficiency at higher temperatures (an exothermic process).
3.5.5. The influence of ionic strength
In this system, electrostatic interactions between the adsorbent surface and the adsorbate ions play a crucial role, and an increase in ion strength is expected to lead to a reduction in adsorption capacity. If electrostatic attraction were repulsive, higher ionic strength would enhance adsorption [Citation39]. The experimental data, conducted under constant conditions with a contact time of 120 min, varying KNO3 concentrations (0, 0.025, 0.05, 0.057, and 0.1 M), adsorbent dose of 5 mg, and initial dye concentrations of 20 mg/L and 5 mg/L for AR1 and BG dyes, as well as pH values of 2 and 6, revealed a gradual decline in the adsorption of AR1 and BG dye molecules on Cross PANI/Chito-GO-OXS NCs with increasing KNO3 concentrations, as illustrated in . The percentage of AR1 and BG dye removal using Cross PANI/Chito-GO-OXS NCs decreased progressively as the KNO3 concentration increased, indicating a reduction in binding effectiveness in the presence of KNO3. The presence of a large number of KNO3 ions (K+ and NO3) generates a shielding effect, leading to a decrease in the electrostatic attraction across molecules of AR1 and BG and the external surface of Cross PANI/Chito-GO-OXS NCs. Furthermore, the presence of cations such as K+ near the adsorption surface impedes the connection of dye species with the adsorbed solid phase surface [Citation40]. As the KNO3 concentration increases, it leads to diminished adsorption of AR1 and BG dyes.
3.5.6. Comparison with other adsorbents
compares the solution pH, equilibrium time, and qe of Cross PANI/Chito-GO-OXS NCs with other various adsorbents used in this investigation. When compared to other adsorbents, the current adsorbent has a high adsorption capacity for AR1 and BG dyes, as shown in The use of NCs offers a network structure that facilitates the presence of active centers, which are very conducive to enhancing the adsorption of dyes. Consequently, the NCs serve as a framework that enables the effective removal of dye materials. This shows that the Cross PANI/Chito-GO-OXS NCs have a lot of practical application potential for enhanced AR1 dye and BG dye elimination from industrial wastewater and aqueous solutions.
Table 3. Comparision of Cross PANI/Chito-GO-OXS NCs based on solution pH, equilibrium time, and qe with various other adsorbents for the removal of AR1 and BG dyes from aqueous medium.
3.6. Kinetic study
The kinetics of pollutant removal from aqueous solutions utilizing solid-phase sorbents, such as dyes, is critical because it sheds light on adsorption mechanisms and chemical pathways. The total rate of transport, film diffusion, and intraparticle diffusion are all factors that impact on the amount of dyes retained on the surface of Cross PANI/Chito-GO-OXS NCs and the quickest of these steps. The overall rate of movement is controlled, which determines the amount of dyes adsorbed on the surface. The kinetics of AR1 and BG dye adsorption on Cross PANI/Chito-GO-OXS NCs were examined in this study at different contact periods ranging from 5 to 120 min while maintaining the following conditions constant: AR1 and BG dyes have initial dye concentrations of 20 mg/L and 5 mg/L, pH 2 and 6, adsorbent dosage 5 mg and 5 mg, and 22 °C temperature, respectively [Citation46,Citation47].
Adsorption of solutes from aqueous solutions onto solid Cross PANI/Chito-GO-OXS NCs is modeled here by using the Lagergren pseudo-first-order kinetic model [Citation48,Citation49]:
(3)
(3)
Where qe is the amount of adsorbed dyes per unit weight of Cross PANI/Chito-GO-OXS NCs at equilibrium, qt is the amount of adsorbed dyes per unit mass of Cross PANI/Chito-GO-OXS NCs at any time t, and Klag is the first-order rate constant. A linear relationship is obtained by plotting log(qe – qt) against time, as shown in shows the KLag and qe values acquired from this linear plot for AR1 and BG dye removal on Cross PANI/Chito-GO-OXS NCs. The results obtained, however, do not fully support the first-order kinetics hypothesis for AR1 dye species adsorption on Cross PANI/Chito-GO-OXS NCs [Citation50]. This shows that a different kinetic model may be better suited to describe the procedure of AR1 dye adsorption on Cross PANI/Chito-GO-OXS NCs; further investigation is necessary to determine the most suitable model that effectively characterizes the adsorption properties of AR1 dye on Cross PANI/Chito-GO-OXS NCs.
Figure 12. Lagergren curve for AR1 BG dyes uptake on Cross PANI/Chito-GO-OXS NCs vs. time. The experimental conditions are specified in the batch extraction step.
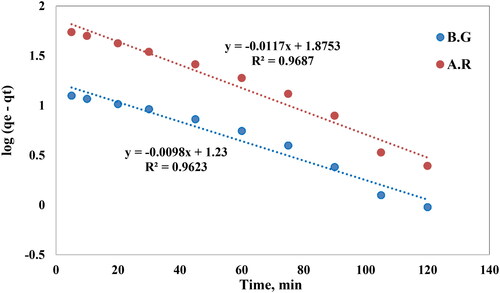
The researchers used the pseudo-second-order kinetic model to elucidate the adsorption behavior of AR1 and BG dyes on Cross PANI/Chito-GO-OXS NCs. The linearized expression of the PSO rate equation may be represented as follows [Citation51]:
(4)
(4)
Where qe is the dyes adsorbed per unit weight of Cross PANI/Chito-GO-OXS NCs at equilibrium, qt is the dyes adsorbed per unit weight of Cross PANI/Chito-GO-OXS NCs at any time t, and k2 is the pseudo-second-order model coefficient. The curves of t/qt against t exhibited a linear relationship when the experimental data was analyzed under the assumptions that (i) the total number of binding sites is determined by the quantity of adsorbate at equilibrium and (ii) the concentration of adsorbate remains constant over time, as shown in . The slope and intercept of these curves for AR1and BG dyes were used to calculate the pseudo-second-order model coefficient (k2) and the equilibrium capacity (qe) of the dye species. The results of the data collection are shown in . The results demonstrate that the pseudo-second-order kinetic model is appropriate for characterizing the adsorption process of AR1 dye using Cross PANI/Chito-GO-OXS NCs. Contact time, solution pH, dye concentration, and temperature are among the experimental variables that influence k2 values [Citation52]. This underscores the need to consider these factors while investigating adsorption kinetics to get a deeper understanding and improve the adsorption process.
Figure 13. Pseudo-second order curves for AR1 and BG dye uptake onto Cross PANI/Chito-GO-OXS NCs vs. time. The experimental conditions are specified in the batch extraction step.
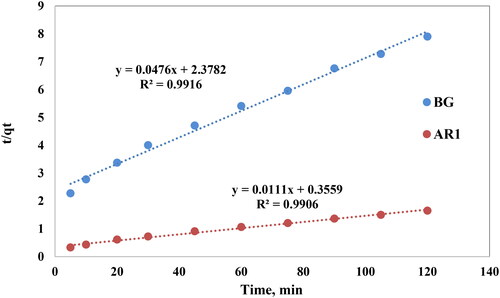
The Elovich equation is an additional kinetic model used to elucidate the process of adsorption of adsorbates in an aqueous environment onto a solid adsorbent [Citation40]. This model is especially useful for chemisorption and is frequently applied to systems with heterogeneous adsorbing surfaces. The following equation represents the Elovich model:
(5)
(5)
Where and stand for the rate of initial adsorption and desorption coefficient, respectively. When qt was plotted against ln(t), the curves produced were linear, as seen in .
Figure 14. Curves of the Elovich model for dye uptake by Cross PANI/Chito-GO-OXS NCs vs. time. The experimental conditions are specified in the batch extraction step.
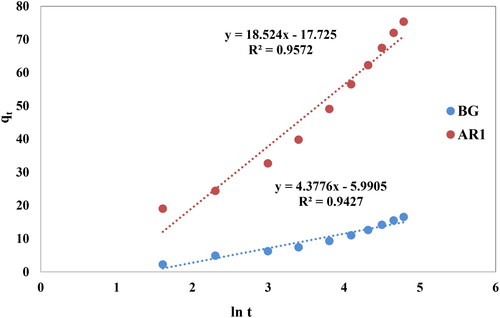
The slopes and intercepts of the dyes’ linear plots were used to calculate the values of and coefficients of the Elovich model. summarizes the numerical data obtained. The adsorption process of AR1 and BG dyes using Cross PANI/Chito-GO-OXS NCs is well described by the Elovich kinetic model, as shown by the data. The Elovich model sheds light on the chemisorption behavior and heterogeneity of the adsorbing surface in this system. The measured coefficients provide insight into the initial adsorption rate and desorption process, assisting in enhancing comprehension of the adsorption process.
Table 4. The parameters for several kinetic models for the removal of BG dye and AR1 dye from Cross PANI/Chito-GO-OXS NCs were determined at 22 °C.
Intra-particle diffusion model were used to study the adsorption of AR1 and BG dyes onto Cross PANI/Chito-GO-OXS NCs surface by following the equation [39]:
(6)
(6)
where kid is the intra-particle diffusion rate constant (mg/g min1/2) and C (mg/g) is a constant proportional to the thickness of the boundary layer. Applying the intra-particle diffusion model, all of the experimental data from adsorption in . The intra-particle diffusion model is appropriate for all of the experimental data since it converged well with zero intercepts and had straight lines that passed through the origin and the figure. The value of Kid was equal to 1.47 and 6.76 for AR1 BG, respectively, with correlation coefficient (R2) 0.992 and 0.996 for BG A and AR1, respectively, as illustrated in .
Figure 15. Intra-particle diffusion model for the AR1 and BG dyes removed from an aqueous solution by Cross PANI/Chito-GO-OXS NCs.
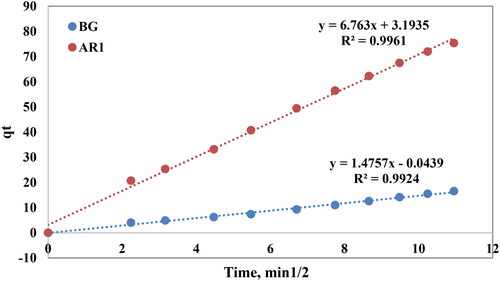
Based on the correlation coefficient values presented in and an analysis of the experimental data obtained from various kinetic models (Lagergren pseudo-first-order, pseudo-second-order, Elovich, and intra-particle diffusion models), it is evident that the pseudo-second-order kinetic model is the most suitable for describing the adsorption process of dyes AR1 and BG onto Cross PANI/Chito-GO-OXS NCs. Among the kinetic models examined, the pseudo-second-order model exhibited the highest correlation coefficient, indicating a superior agreement between the experimental data and the model’s predictions. This finding strongly suggests that the adsorption process follows pseudo-second-order kinetics, implying that chemisorption is the most likely mechanism governing the adsorption of dyes on Cross PANI/Chito-GO-OXS NCs. The selection of the model that fits best, the pseudo-second-order model, offers valuable insights into the adsorption behavior and rate-limiting processes of this process. It can play a pivotal role in optimizing adsorption conditions and enhancing the adsorption methods efficiency for dye removal from aqueous solutions using Cross PANI/Chito-GO-OXS NCs as the adsorbent [Citation53].
3.7. Thermodynamics of AR1 and BG dyes absorption by Cross PANI/Chito-GO-OXS NCs
To determine dye retention on the adsorbent, the sorption of AR1 and BG dyes by Cross PANI/Chito-GO-OXS NCs was investigated over a temperature range of 285–318 K. Using adsorption data at various temperatures, the Van’t Hoff equation was used to calculate entropy of adsorption (ΔS) and the apparent enthalpy of adsorption (ΔH) [Citation54,Citation55]:
(7)
(7)
Following that, calculate the free energy of adsorption (ΔG) by using the equation below [Citation54,Citation55]:
(8)
(8)
Where T stands for Kelvin temperature, R stands for gas constant, and KC is for equilibrium constant.
The equilibrium constant (KC) for the adsorption of AR1 and BG dyes was calculated using the equation:
(9)
(9)
In the provided system, Ce represents the equilibrium concentration of AR1 and BG dyes in the aqueous solution (mg/L), and Ca represents the concentration of AR1and BG dyes adsorbed onto the solid surface per liter at equilibrium (mg/L). The plots of ln KC against 1000/T for the retention of AR1and BG dyes on Cross PANI/Chito-GO-OXS NCs across a temperature range from 285 to 318 K are depicted in . With an increase in temperature, the equilibrium constant for BG dye decreases. This indicates that the retention of BG dye on Cross PANI/Chito-GO-OXS NCs is an exothermic process. The adsorption process is considered exothermic when the enthalpy change (ΔH) is negative, signifying a reduction in bond energy between the solid surface and the dyes. A negative (ΔS) value suggests that the reorientation step governs entropy at the activation state, resulting in the formation of a non-electrostatic bond between the solid phase and adsorbent material. Since the Gibbs free energy (ΔG) is negative at 295 K for BG dye, the adsorption process is both spontaneous and physical. In contrast, the equilibrium constant for AR1 dye increases with rising temperature, indicating an endothermic process. A positive (ΔH) value implies that heat is absorbed during the adsorption of AR1 dye. The positive ΔS value for Cross PANI/Chito-GO-OXS NCs suggests an increase in degrees of freedom at the solid–liquid interface, likely due to the release of water molecules from the hydration sphere during adsorption. Because (ΔG) is negative at 295 K, the adsorption of AR1 dye on Cross PANI/Chito-GO-OXS NCs is also spontaneous and physical. In summary, the thermodynamic study provides insights into the nature of adsorption process and the interactions between the dyes and Cross PANI/Chito-GO-OXS NCs at different temperatures. summarizes the thermodynamic characteristics for the adsorption of AR1 and BG dyes using Cross PANI/Chito-GO-OXS NCs.
Figure 16. Curves showing ln Kc against 1000/T for BG dye and AR1 dye uptake from aquatic solution by Cross PANI/Chito-GO-OXS NCs.
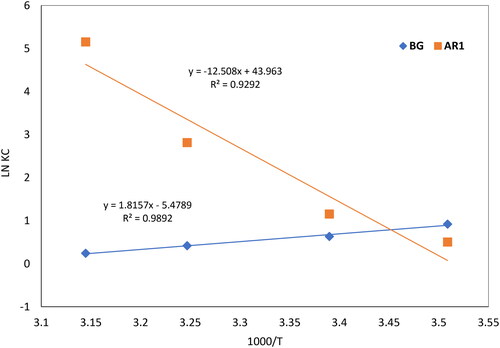
Table 5. Thermodynamic characteristics for AR1 and BG dye adsorption using Cross PANI/Chito-GO-OXS NCs.
3.8. Application in the environment
The capability of Cross PANI/Chito-GO-OXS NCs to adsorb and remove dyes from actual water samples was evaluated. Three distinct water samples were collected from various sources, namely Red Sea water, wastewater from a water treatment facility, and laboratory tap water. Initially, it was determined that the concentrations of AR1 and BG dyes in these samples were below the detection limit of UV–vis spectroscopy.
To assess the adsorption capacity of Cross PANI/Chito-GO-OXS NCs in real water samples, three water samples were intentionally spiked with AR1 dye at a concentration of 20 mg/L and BG dye at a concentration of 5 mg/L. The solutions were agitated for 120 min at a temperature of 295 K, with the pH adjusted to 2 for AR1 dye and 6 for BG dye.
The results revealed that Cross PANI/Chito-GO-OXS NCs efficiently removed dyes from real water samples, with removal percentages of 91.67%, 93.04%, and 95.55% for BG dye in ocean water, wastewater, and tap water, respectively. Similarly, the removal percentages for AR1 dye in seawater, wastewater, and tap water were found to be 93.17%, 94.52%, and 96.73%, respectively, as depicted in .
Figure 17. The efficiency of AR1 and BG dyes removed by Cross PANI/Chito-GO-OXS NCs from various real three samples, (experimental conditions: 25 mL solution, contact time = 120 min, pH solution = 6, temperature = 295 K, 12.5 mg of Cross PANI/Chito-GO-OXS NCs and 5 mg/L concentration for BG dye, and for the AR1 dye 25 mL solution, contact time = 120 min, pH solution = 2, temperature = 295 K, 12.5 mg of Cross PANI/Chito-GO-OXS NCs and 20 mg/L concentration).
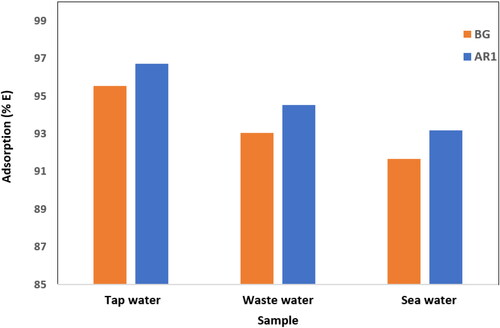
3.9. Reusability
After the adsorption process, Cross PANI/Chito-GO-OXS NCs were collected, washed with acetone to eliminate the adsorbed dyes, and then dried. To assess the adsorbent’s recyclability, four adsorption-harvesting cycles were conducted. The results indicated that even after four cycles, the adsorption capacity of Cross PANI/Chito-GO-OXS NCs for AR1 and BG dyes remained nearly unchanged, with only a minor decrease. This underscores the ability of Cross PANI/Chito-GO-OXS NCs to be reused and treated multiple times without a significant loss in adsorption efficiency, as displayed in . This research underscores the potential of Cross PANI/Chito-GO-OXS NCs as a practical adsorbent for the removal of dyes from real water samples. Such a capability is essential for pollution control and environmental preservation. The recyclability of the adsorbent and its consistent adsorption performance make it an attractive choice for real-world applications in water treatment processes.
4. Conclusions
In this study, PANI/Chito-GO-OXS NCs were synthesized, purified, and functionalized, resulting in the creation of Cross PANI/Chito-GO-OXS NCs. These novel compounds were found to be highly effective adsorbents for the removal of AR1 dye and BG dye from aqueous solutions and wastewater. A comprehensive characterization of Cross PANI/Chito-GO-OXS NCs was conducted using various analytical techniques. The batch technique was employed to investigate key parameters influencing the efficiency of AR1 dye and BG dye removal, including adsorbent dose, agitation time, pH, and temperature. The results demonstrated a remarkable removal efficiency, with a 96.73% removal of AR1 dye and a 95.55% removal of BG dye in real wastewater samples. The pseudo-second-order model was identified as the most appropriate, with R2 values of 0.9839 for AR1 and 0.9928 for BG, respectively, indicating a strong fit between the model predictions and the experimental data. The study also delved into the thermodynamic parameters (ΔG, ΔH, and ΔS) of the adsorption process. An endothermic adsorption process was observed for AR1 dye, suggesting heat absorption during the process. In contrast, the elimination of BG dye was exothermic, spontaneous, and characterized by chemisorption, signifying robust chemical interactions between the adsorbent and dye molecules. Overall, this work underscores the potential of Cross PANI/Chito-GO-OXS NCs as highly efficient and versatile adsorbents for dye removal. The exploration of various factors influencing the adsorption process and the determination of thermodynamic parameters contribute to a deeper understanding of the adsorption mechanism and can aid in optimizing the adsorption process for practical applications in water treatment and pollution control.
Acknowledgments
The authors extend their appreciation to the Deputyship for Research & Innovation, Ministry of Education in Saudi Arabia, for funding this research work through project number: IFP22UQU4350651DSR167
Disclosure Statement
The authors declare that they have no known financial or interpersonal conflicts that would have seemed to have an impact on the research presented in this study.
Additional information
Funding
Notes on contributors
Hanan K. Alzahrani
Hanan K. Alzahrani, received her Ph.D. from King Abdulaziz University, and she is an Assistant Professor of physical Chemistry at Umm Al-Qura University. Her research interest covers catalysis, synthesis of catalyst, natural products, materials chemistry, zeolite, environmental, treatment, and removal applications…etc.
Dina F. Katowah
Dina F. Katowah, received her Ph.D. from King Abdulaziz University, and she is an Assistant Professor of Organic Chemistry at Umm Al-Qura University. Her research interest covers polymer chemistry, synthesis of novel polymers and polymer nanocomposite, natural products, materials chemistry, environmental, medical, and sensor applications…etc.
References
- Soliman NK, Mohamed HS, Ahmed SA, et al. Cd2+ and Cu2+ removal by the waste of the marine brown macroalga hydroclathrus clathratus. Env Technol Innov. 2019;15:100365. doi: 10.1016/j.eti.2019.100365.
- Hidayat E, Harada H, Mitoma Y, et al. Rapid removal of acid red 88 by zeolite/chitosan hydrogel in aqueous solution. Polymers. 2022;14(5):893. doi: 10.3390/polym14050893.
- Srivastava A, Rani RM, Patle DS, et al. Emerging bioremediation technologies for the treatment of textile wastewater containing synthetic dyes: a comprehensive review. J Chem Tech Biotech. 2022;97(1):26–41. doi: 10.1002/jctb.6891.
- Bhowmik M, Debnath A, Saha B. Fabrication of mixed phase CaFe2O4 and MnFe2O4 magnetic nanocomposite for enhanced and rapid adsorption of methyl orange dye: statistical modeling by neural network and response surface methodology. J Dispersion Sci Technol. 2020;41(13):1937–1948. doi: 10.1080/01932691.2019.1642209.
- Katowah DF, Abdel-Fadeel MA. Ultrahigh adsorption capacity of a new metal sieve-like structure nanocomposite-based chitosan-graphene oxide nanosheet coated with poly-o-toluidine for the removal of acid red dye from the aquatic environment. Nanocomposites. 2023;9(1):80–99. doi: 10.1080/20550324.2023.2251677.
- Mani S, Chowdhary P, Bharagava RN. Textile wastewater dyes: toxicity profile and treatment approaches. Emerging and Eco-Friendly Approaches for Waste Management. 2019;1:219–244.
- Liu W, Kong F, Zhang J, et al. Modification of haematococcus pluvialis algal residue by ionic liquid for improved extraction of astaxanthin followed by removal of acid red dye in water. Algal Res. 2022;64:102656. doi: 10.1016/j.algal.2022.102656.
- Rehman R, Muhammad SJ, Arshad M. Brilliant green and acid orange 74 dyes removal from water by pinus roxburghii leaves in naturally benign way: an application of green chemistry. J Chem. 2019;2019:1–10. doi: 10.1155/2019/3573704.
- Alemu A, Kerie E. Removal of acid yellow 17 dye from aqueous solutions using activated water hyacinth (eichhornia crassipes). Water Pract Technol. 2022;17(6):1294–1304. doi: 10.2166/wpt.2022.063.
- Chatterjee S, Guha N, Krishnan S, et al. Selective and recyclable Congo red dye adsorption by spherical Fe3O4 nanoparticles functionalized with 1, 2, 4, 5-benzenetetracarboxylic acid. Sci Rep. 2020;10(1):111. doi: 10.1038/s41598-019-57017-2.
- Deb A, Kanmani M, Debnath A, et al. Ultrasonic assisted enhanced adsorption of methyl orange dye onto polyaniline impregnated zinc oxide nanoparticles: kinetic, isotherm and optimization of process parameters. Ultrason Sonochem. 2019;54:290–301. doi: 10.1016/j.ultsonch.2019.01.028.
- Deb A, Debnath A, Saha B. Ultrasound‐aided rapid and enhanced adsorption of anionic dyes from binary dye matrix onto novel hematite/polyaniline nanocomposite: response surface methodology optimization. Appl Organom Chem. 2020;34(2):e5353. doi: 10.1002/aoc.5353.
- Samadi A, Xie M, Li J, et al. Polyaniline-based adsorbents for aqueous pollutants removal: a review. Chem Eng J. 2021;418:129425. doi: 10.1016/j.cej.2021.129425.
- Deb A, Das S, Debnath A. Fabrication and characterization of organometallic nanocomposite for efficient abatement of dye laden wastewater: CCD optimization, adsorption mechanism, co-existing ions, and cost analysis. Chem Phys Lett. 2023;830:140820. doi: 10.1016/j.cplett.2023.140820.
- Lefatle MC, Madikizela LM, Pakade VE, et al. Magnetic chitosan-zeolite composite as an adsorbent in ultrasound-assisted magnetic solid phase extraction of tetracyclines in water samples. J Anal Sci Technol. 2023;14(1):28. doi: 10.1186/s40543-023-00394-1.
- Abo Elsoud MM, El Kady E. Current trends in fungal biosynthesis of chitin and chitosan. Bull Natl Res Cent. 2019;43(1):1–12. doi: 10.1186/s42269-019-0105-y.
- Kumar S, Bera R, Das N, et al. Chitosan-based zeolite-Y and ZSM-5 porous biocomposites for H2 and CO2 storage. Carbohydr Polym. 2020;232:115808. doi: 10.1016/j.carbpol.2019.115808.
- Wu M, Chen W, Mao Q, et al. Facile synthesis of chitosan/gelatin filled with graphene bead adsorbent for orange II removal. Chem Eng Res Des. 2019;144:35–46. doi: 10.1016/j.cherd.2019.01.027.
- Noreen S, Tahira M, Ghamkhar M, et al. Treatment of textile wastewater containing acid dye using novel polymeric graphene oxide nanocomposites (GO/PAN, GO/PPy, GO/PSty). J Mater Res Technol. 2021;14:25–35. doi: 10.1016/j.jmrt.2021.06.007.
- Yang J, Shojaei S, Shojaei S. Removal of drug and dye from aqueous solutions by graphene oxide: adsorption studies and chemometrics methods. Npj Clean Water. 2022;5(1):5. doi: 10.1038/s41545-022-00148-3.
- Mao B, Sidhureddy B, Thiruppathi AR, et al. Efficient dye removal and separation based on graphene oxide nanomaterials. New J Chem. 2020;44(11):4519–4528. doi: 10.1039/C9NJ05895H.
- Minisy I, Salahuddin N, Ayad M. Chitosan/polyaniline hybrid for the removal of cationic and anionic dyes from aqueous solutions. J Appl Polymer Sci. 2019;136(6):47056. doi: 10.1002/app.47056.
- Bryan MYK, Chai PV, Law JY, et al. Graphene oxide-chitosan composite material as adsorbent in removing methylene blue dye from synthetic wastewater. Mater Today: Proc. 2022;64:1587–1596. doi: 10.1016/j.matpr.2022.03.092.
- Wang Y, Pan C, Chu W, et al. Environmental remediation applications of carbon nanotubes and graphene oxide: adsorption and catalysis. Nanomaterials. 2019;9(3):439. doi: 10.3390/nano9030439.
- Mashkoor F, Nasar A. I. Carbon nanotube-based adsorbents for the removal of dyes from waters: a review. Environ Chem Lett. 2020;18:605–629. doi: 10.1007/s10311-020-00970-6.
- Katowah DF, Saleh SM, Mohammed GI, et al. Ultra-efficient hybrid material-based cross-linked PANI@ Cs-GO-OXS/CuO for the photocatalytic degradation of Rhodamine-B. J Phys Chem Solids. 2021;157:110208. doi: 10.1016/j.jpcs.2021.110208.
- Katowah DF, Hussein MA, Alam MM, et al. Poly (pyrrole-co-o-toluidine) wrapped CoFe 2 O 4/R (GO–OXSWCNTs) ternary composite material for Ga 3+ sensing ability. RSC Adv. 2019;9(57):33052–33070. doi: 10.1039/C9RA03593A.
- Katowah DF, Saleh SM, Alqarni SA, et al. Network structure-based decorated CPA@ CuO hybrid nanocomposite for methyl orange environmental remediation. Sci Rep. 2021;11(1):5056. doi: 10.1038/s41598-021-99791-y.
- Katowah DF, Mohammed GI, Al‐Eryani DA, et al. Fabrication of conductive cross‐linked polyaniline/G‐MWCNTS core‐shell nanocomposite: a selective sensor for trace determination of chlorophenol in water samples. Polym Adv Tech. 2020;31(11):2615–2631. doi: 10.1002/pat.4988.
- Katowah DF, Mohammed GI, Adeosun WA, et al. Impact of CuO nanoparticles on the performance of ternary conductive C-PANI/(OXSWCNTs-GO-CS)/CuO network as a selective chlorophenol sensor. Polym Plast Technol Mater. 2021;60(13):1–17. doi: 10.1080/25740881.2021.1904986.
- Gorduk O, Gencten M, Gorduk S, et al. Electrochemical fabrication and supercapacitor performances of metallo phthalocyanine/functionalized-multiwalled carbon nanotube/polyaniline modified hybrid electrode materials. J Storage Mater. 2021;33:102049. doi: 10.1016/j.est.2020.102049.
- Ibrahim NI, Wasfi AS. A comparative study of polyaniline/MWCNT with polyaniline/SWCNT nanocomposite films synthesized by microwave plasma polymerization. Synth Met. 2019;250:49–54. doi: 10.1016/j.synthmet.2019.02.007.
- Katowah DF, Mohammed GI, Al-Eryani DA, et al. Rapid and sensitive electrochemical sensor of cross-linked polyaniline/oxidized carbon nanomaterials core-shell nanocomposites for determination of 2, 4-dichlorophenol. PLoS ONE. 2020;15(6):e0234815. doi: 10.1371/journal.pone.0234815.
- Katowah DF, Alam MM, Hussein MA, et al. Core–shell-shell structured P (ani-co-Py)/NiF-grafted-(go-PPD)-PPy nanocomposites prepared via two steps polymerization of conducting polymer for sensitive Tl3+ detection. Surf Interfaces. 2023;42:103374. doi: 10.1016/j.surfin.2023.103374.
- Katowah DF, Rahman MM, Hussein MA, et al. Ternary nanocomposite based poly (pyrrole-co-O-toluidine), cobalt ferrite and decorated chitosan as a selective Co2+ cationic sensor. Composites Part B: Eng. 2019;175:107175. doi: 10.1016/j.compositesb.2019.107175.
- Katowah DF, Asiri AM, Rahman MM. Development of novel nanocomposites based on SrSnO3-conjugated coconut-shell activated carbon with conducting polymers towards 4-nitrophenol detection by electrochemical approach. Surf Interfaces. 2023;41:103241. doi: 10.1016/j.surfin.2023.103241.
- Manjunath S, Baghel RS, Kumar M. Performance evaluation of cement–carbon composite for adsorptive removal of acidic and basic dyes from single and multi-component systems. Env Technol Innov. 2019;16:100478. doi: 10.1016/j.eti.2019.100478.
- Nizam NUM, Hanafiah MM, Mahmoudi E, et al. The removal of anionic and cationic dyes from an aqueous solution using biomass-based activated carbon. Sci Rep. 2021;11(1):8623. doi: 10.1038/s41598-021-88084-z.
- Katowah DF, Al-Zahrani HK. A new ternary nanocomposites-based cellulose derivatives-CuFe2O4-Zeolite with ultra-high adsorption capacity for brilliant green dye treatment and removal from the aquatic environment. J Saudi Chem Soc. 2023;27(6):101764. doi: 10.1016/j.jscs.2023.101764.
- Abdel-Fadeel MA, Aljohani NS, Al-Mhyawi SR, et al. A simple method for removal of toxic dyes such as brilliant green and acid red from the aquatic environment using halloysite nanoclay. J Saudi Chem Soc. 2022;26(3):101475. doi: 10.1016/j.jscs.2022.101475.
- Munilakshmi N, Srimurali M, Karthikeyan J. Adsorptive removal of acid red 1, from aqueous solutions by preformed flocs. Int J Curr Eng Technol. 2013;3(4):1456–1462.
- Alizadeh S, Seyyedi K. Removal of CI acid red 1 (AR1) dye pollutant from contaminated waters by adsorption method using sunflower seed shells and pine cone as agro waste materials. J Appl Chem Res. 2019;13(4):93–105.
- Ahmad R, Ansari K. Chemically treated lawsonia inermis seeds powder (CTLISP): an eco-friendly adsorbent for the removal of brilliant green dye from aqueous solution. Groundwater Sustainable Dev. 2020;11:100417. doi: 10.1016/j.gsd.2020.100417.
- Baidya KS, Kumar U. Adsorption of brilliant green dye from aqueous solution onto chemically modified areca nut husk. S Afr J Chem Eng. 2021;35:33–43. doi: 10.1016/j.sajce.2020.11.001.
- Vyavahare G, Gurav R, Patil R, et al. Sorption of brilliant green dye using soybean straw-derived biochar: characterization, kinetics, thermodynamics and toxicity studies. Env Geochem Health. 2021;43(8):2913–2926. doi: 10.1007/s10653-020-00804-y.
- Gabal MA, Al-Juaid AA, El-Rashed S, et al. Structural, thermal, magnetic and electrical properties of polyaniline/CoFe2O4 nano-composites with special reference to the dye removal capability. J Inorg Organomet Polym. 2019;29(6):2197–2213. doi: 10.1007/s10904-019-01179-z.
- Gabal MA, Al-Harthy EA, Al Angari YM, et al. Synthesis, characterization and dye removal capability of conducting polypyrrole/Mn0.8Zn0.2Fe2O4/graphite oxide ternary composites. Catalysts. 2022;12(12):1624. doi: 10.3390/catal12121624.
- Al-Saidi HM, Abdel-Fadeel MA, Alharthi SS. Preconcentration and ultrasensitive spectrophotometric estimation of tungsten in soils using polyurethane foam in the presence of rhodamine B: kinetic and thermodynamic studies, and designing a simple automated preconcentration system. J Saudi Chem Soc. 2021;25(8):101301. doi: 10.1016/j.jscs.2021.101301.
- El‐Attar HG, Salem MA, Bakr EA. Facile synthesis of recoverable superparamagnetic AgFeO2@ polypyrrole/SiO2 nanocomposite as an excellent catalyst for reduction and oxidation of different dyes in wastewater. Appl Organom Chem. 2021;35(10):e6357. doi: 10.1002/aoc.6357.
- Hameed SA, Abdel-Fadeel MA, Al-Saidi HM, et al. Simultaneous removal of the toxic tungsten ions and rhodamine B dye by graphene nanosheets from model and real water. DWT. 2020;188:266–276. doi: 10.5004/dwt.2020.25348.
- Salam MA, Lateefa A, Abdel-Fadeel MA. Removal of toxic ammonium ions from water using nanographene sheets. DWT. 2018;129:168–176. doi: 10.5004/dwt.2018.23085.
- Braun T, Navratil JD, Farag A. Polyurethane foam sorbents in separation science. Boca Raton: CRC press; 2018.
- Parimelazhagan V, Yashwath P, Arukkani Pushparajan D, et al. Rapid removal of toxic remazol brilliant blue-R dye from aqueous solutions using juglans nigra shell biomass activated carbon as potential adsorbent: optimization, isotherm, kinetic, and thermodynamic investigation. Int J Mol Sci. 2022;23(20):12484. doi: 10.3390/ijms232012484.
- Althomali RH, Alamry KA, Hussein MA, et al. Modification of alginic acid for the removal of dyes from aqueous solutions by solid-phase extraction. Int J Env Anal Chem. 2022;102(16):3673–3693. doi: 10.1080/03067319.2020.1772772.
- Alqarni SA. Deliberated system of ternary core–shell polythiophene/ZnO/MWCNTs and polythiophene/ZnO/ox-MWCNTs nanocomposites for brilliant green dye removal from aqueous solutions. Nanocomposites. 2022;8(1):47–63. doi: 10.1080/20550324.2022.2054209.