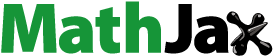
Abstract
Nanocomposites represent one of the most useful strategies for resolving the pharmaceutical challenge about the dissolution and delivery of poorly water-soluble drugs. Besides the compatibility between the drug and polymeric carriers, the physical shapes of materials also play their important roles on the release behaviors of a guest poorly water-soluble drug from the host polymeric matrices. In this study, three kinds of nanocomposites in the forms of homogeneous nanofibers (E1), spindles-on-a-string (E2), and beads-on-a-string (E3) were prepared using electrospinning with ketoprofen (KET) as the model drug and a mixture of ethylcellulose (EC) and polyvinylpyrrolidone (PVP) as the polymeric matrices. Controllable preparation mechanisms of these morphologies are disclosed based on the results of SEM, TEM, and processes observations. XRD and FTIR data demonstrated that KET was compatible with PVP and EC. In vitro dissolution tests verified that all the three nanocomposites were able to provide the typical bi-stage fast-sustained release profiles of KET. Whereas, the beads-on-a-string E3 had a better functional performance than the spindles-on-a-string E2, and the homogeneous nanofibers E1 in terms of the KET sustained release profiles in the second stage. The protocols reported here pioneered a new way for developing novel functional nanocomposites based on the process-shape-performance relationship.
1. Introduction
During the past three decades of nano surging, a wide variety of nano techniques have tried their possibilities in resolving the key and long existing challenges about the dissolution and delivery of poorly water-soluble drugs [Citation1,Citation2]. In most cases, the medicated nanocomposites are the intermediate media between the nano techniques and a certain poorly water-soluble drug, in which the drug molecules are often distributed all over the drug carriers in an amorphous state [Citation3,Citation4]. For a successful material conversion from the raw materials to the composite nano products, two keys are there. One is the reasonable selection of the drug carriers, which should have a fine compatibility with the loaded drugs [Citation5,Citation6]. These drug carriers can be polymers [Citation7,Citation8], proteins [Citation9,Citation10], lipids, and even inorganic materials [Citation11,Citation12]. Among them, ‘polymers’ are the mainstream in modern pharmaceutical industry, which is regarded as the backbone for supporting the developments of pharmaceutics during the past half century [Citation13,Citation14].
The other is the facility and practicability of the nano techniques. In general, the nano techniques have two approaches, one is the bottom-up method, such as self-assembly and chemical syntheses [Citation15]. The other is the top-down methods, such as electrospinning [Citation16,Citation17] and electrospraying [Citation18,Citation19], which also belong to electrohydrodynamic atomization (EHDA) methods because electrostatic energy is exploited for converting the working fluids into solid products [Citation20–22]. It is thought that the popularity of electrospinning is resulted from its facile and straightforward process for nano fabrication and the well-known unique properties of electrospun nanofibers (such as small diameter, huge porosity, and large surface area) [Citation23,Citation24]. However, the powerful capability of creating polymer-based composites through electrospinning in the form of nanofibers should be a strong contribution to its popularity [Citation25,Citation26]. Although only about 200 polymers are reported to be able to electrospun into nanofibers [Citation27,Citation28], the number of guest active ingredients that can be distributed within the filament-forming polymers is numerous. These active ingredients mostly co-present with the polymers in the nanofibers in a molecular dispersion manner, suggesting excellent nanocomposites other than hybrids [Citation29,Citation30].
Since the reviving of electrospinning in 1990s, electrospinning is moving forward from the single-fluid blending process [Citation31] to bi-fluid coaxial [Citation32,Citation33] and side-by-side process [Citation34], and to tri-fluid side-by-side [Citation35], tri-axial [Citation36–38], and other complicated processes [Citation39]. These processes are powerful in creating all types of inner multi-chamber nanostructures, such as core-shell [Citation40], Janus [Citation41], tri-layer core-shell [Citation42], tri-layer Janus [Citation43], and the combinations of core-shell and Janus, such as the so-called JCS structure (Janus structure with one side composed of core-shell structure) [Citation39]. In turn, these multi-chamber structure can provide a strong platform for designing novel functional nanocomposites based on the process-structure-performance relationship [Citation44]. However, the outer shape of the electrospun nanofibers is overlooked to a certain extent, although nanofibers in the shape of ribbons or cylinder or dented surface have been noticed in literature [Citation45–47]. Particularly, the formed spindles-on-a-string or beads-on-a-string hybrids are frequently regarded as abnormal products and were abandoned [Citation48]. This case is similar to electrospraying, in which particles are needed and spindles-on-a-string or beads-on-a-string hybrids are thought to be unnecessary products [Citation49].
Both electrospinning and electrospraying takes the advantages of the easy interaction between electrostatic energy with working fluids [Citation50–52]. Based on this central idea, on one hand, the electrospinning system is clearly composed of four parts (): a power supply to provide the high voltage, one or more syringes, and their pumps to drive the working fluids in a quantitative manner, a spinneret to guide the working fluids to the electric field, and a collector for the resultant nanofibers to deposit [Citation53,Citation54]. On the other hand, the properties of the working fluids comprise the most important parameters for both the working processes and also the final EHDA products. Particularly, the concentrations of the filament-forming polymer in the working fluids are key elements to determine the electrospinnability or solidifiability of the fluids. The diagram at the bottom section of shows the relationships between the working fluids’ processability and the formats of final polymeric composite products.
When the polymer concentration in a working fluid is enough to support the abundant physical entanglements, i.e. the elasticity and viscosity are large enough to balance an electrical drawing in the electrostatic field, the working fluid is regarded as an electrospinnable fluid, and correspondingly the resultant EHDA products are linear nanofibers. Often, each filament-forming polymer has its electrospinnable window, i.e. a concentration range for creating solid linear nanofibers. As the concentration of polymer in the fluid reduces below the electrospinnable window, the EHDA products would be spindles-on-a-string or beads-on-a-string hybrids [Citation47–49]. Further reductions of polymer concentration would still be solidifiable, but the electrospinning process would degrade into an electrospraying process, and the products would be solid micro-/nano particle. The extremely dilute solution would be converted into wet membrane, i.e. the applied voltage can’t exhaust all the solvent from the working fluid during an EHDA process. Thus, the most important parameter for determining the morphologies of an EHDA product is the concentration of the filament-forming polymer, or vice visa, the polymer concentration can be exploited as a useful tool to manipulate the morphologies of the final polymer-based composites.
Ketoprofen (KET) is frequently exploited for treating fever and ache in clinic. A fast release initially for removing the symptoms and a later sustained release of less time administration for patients’ compliance are highly desired [Citation55]. Ethyl cellulose (EC) is an inert and insoluble polymer. It is frequently utilized for providing drug sustained release profiles [Citation56]. Polyvinylpyrrolidone (PVP), a soluble polymeric carrier, is reported to be able to enhance the solubility of around 200 poorly water-soluble drugs [Citation57]. A blending of PVP and EC as both the filament-forming matrices and also the drug carriers for producing the polymeric medicated nanocomposites is expected to provide the desired bi-stage release of KET.
In view of the above-mentioned situations, this study aimed to disclose how to controllably manipulate the creations of various EHDA morphologies (including linear composite nanofibers, spindles-on-a-string, and beads-on-a-string composite products), the related process-morphology relationships and the nano fabrication mechanism. These EHDA products would be experienced systematic characterizations about their shapes, inner structures, physical state of components and their compatibility, and the in vitro KET controlled release profiles. The structure-performance relationships based on various EHDA products will be disclosed.
2. Materials and methods
2.1. Materials
Polyvinylpyrrolidone K60 (PVP, Mw = 360,000), ethyl cellulose (EC), and ketoprofen (KET) were purchased from Shanghai Fine Chemical Co., Ltd. (Shanghai, China). The solvents dichloromethane (DCM) and anhydrous ethanol were obtained from Shanghai Chemical Regents Co., Ltd. (Shanghai, China). All other chemicals are analytical reagents. Water was doubly distilled just before usage.
2.2. EHDA fabrication processes
The EHDA system was homemade [Citation10,Citation23,Citation31,Citation37]. The spinneret was prepared from G20 needle, which had an inner and outer diameters of 0.60 and 0.91 mm, respectively. A KDS100 (Cole-Parmer®, USA) syringe pump was exploited to quantitatively drive the working fluids to the inlets of spinneret. A high voltage generator (60 kV/2 mA, 2000ZGF) was bought from Wuhan Huatian High Power Co., Ltd. (Wuhan, China). The product collector was simply prepared using a cardboard to be wrapped by a sheet of aluminum foil. The power supply and the collector must be grounded due to prevention of possible electric shock. The ambient conditions included an environmental temperature of 21 ± 4 °C and a relatively humidity of 52 ± 5%. The parameters for creating EHDA products E1, E2, E3, and E4 are listed in .
Table 1. Parameters for preparing the EHDA products with various morphologies.Table Footnotea
2.3. Characterizations
2.3.1. Morphology and inner structure
The morphologies of the three electrohydrodynamic atomization (EHDA) products were evaluated using a scanning electron microscope (SEM, Quanta FEG450, FEI Corporation). Before evaluations, the EHDA samples were placed on the conductive tapes and coated with Au for 60 s under a nitrogen atmosphere. The diameters of EHDA products were estimated using ImageJ software. The inner structure of the EHDA products was assessed through a transmission electron microscope (TEM, JEM2200F, JEOL, Japan). The applied electron voltage for observation was 300 kV.
2.3.2. Physical state and compatibility
Both the raw components (EC, PVP, and KET powders) and their composite EHDA products were experienced the X-ray diffraction measurements (XRD, Bruker-AXS, Karlsruhe, Germany) for determining their physical state. Attenuated Total Reflectance-Fourier Transform Infrared (ATR-FTIR, PerkinElmer, Billerica, MA, USA) was utilized to investigate the compatibility between the guest KET and host polymeric composites of EC and PVP.
2.4. Drug encapsulation and in vitro bi-stage controlled release
The drug encapsulation efficiency (EE%) was assessed. The procedures are listed as follows: a fixed amount of 0.1 g EHDA products were accurately weighed; they were dissolved into 100 mL 20% ethanol aqueous solution to free all the loaded KET; one milliliter was fetched and was diluted in 200 mL phosphate buffered solution (PBS, pH = 7.0, 0.1 M). The absorbance at λmax = 260 nm was measured using a UV-vis Spectrophotometer (UV-2102PC, Unico Instrument Co. Ltd., Shanghai, China); and the drug concentration could be calculated based the predetermined calibration equation; Finally, the value of EE% could be gotten through the following EquationEquation (1)(1)
(1) :
(1)
(1)
where EE is the entrapment efficiency, Wm is KET measured in the EHDA products, and Wp represents the KET added in the working fluids. All measurements were carried out three times.
In vitro dissolution tests were carried out using a paddle method according to the Chinese Pharmacopoeia (Ed. 2020). An RCZ-8A dissolution apparatus (Tianjin University Radio Factory, Tianjin, China). An amount of 0.1 g EHDA products were placed into the six dissolution vessels, in which 600 mL PBS was kept at a temperature of 37.0 ± 1.0 °C. The paddle rotation rate was 50 rpm. At predetermined time points, 5.0 mL samples were fetched for measurements and 5.0 mL blank PBS in the seventh vessel was compensated for keeping a constant volume of dissolution medium. The quantitative measurements of KET concentrations were conducted as above-mentioned procedures. All the measurements were repeated six times.
3. Results and discussion
3.1. The process-morphology relationship
The working processes for preparing EHDA products E1 to E3 were recorded in . From , the total polymer contents (i.e. the concentrations of both EC and PVP in w/v%, represented by P) in the working fluids reduced from 0.24, to 0.192, and to 0.144, respectively. Further reduction of the P value to 0.096 resulted in an electrospraying process. From these typical working process images, the following phenomena are noticed: (1) the Taylor cone’s height (H) gradually reduced, as the reduction of total polymer contents (P) in the working fluids, a linear relationship can be regressed as H = 0.16 + 1.25 P (R = 0.9954), which is exhibited in (The exploited spinneret was a capillary of G20 needle, which had an inner and outer diameters of 0.60 and 0.91 mm, respectively, and can be exploited as a reference for measuring the height of Taylor cone and the length of the straight fluid jet); (2) The length of straight fluid jet (L) also reduced as the reduction of total polymer contents (P) in the working fluids, a linear relationship is similarly regressed as L = 0.13 + 7.39 P (R = 0.9954), which is exhibited in . These quantitatively related data, reflecting the process-property (fluid) relationships, would be very useful for manipulating the fibrous products’ quality in future large-scale productions of commercial fibrous products; (3) Right after the straight fluid jets in the instable regions, there were always bright dots within the fluid jet in . However, as the bending and whipping processes moved forwards in the instable regions, the density of bright dots reduced to disappear from the fluid jets in for the electrospinnable working fluids. Whereas, the bright dots in are still in a dense manner, and the dot case in is between the situations shown in . The lower polymer concentrations would keep a robust situation that liquid bright dots are accompanied always with the fluid jets.
Figure 2. The observations of the three working progresses for preparing the EHDA products: (a) a typical process for creating the homogeneous nanofibers E1; (b) a typical process for producing the composite spindles-on-a-string E2; (c) a typical digital image for preparing the beads-on-a-string E3; (d) the quantitative relationship between the total polymer (EC and PVP) contents in the working fluids and the heights of their Taylor cone; and (e) the quantitative relationship between the total polymer (EC and PVP) contents in the working fluids and the lengths of their straight fluid jet.
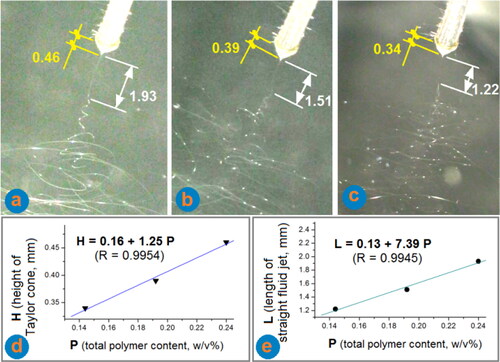
The SEM results of the prepared EHDA products from the working fluids with various total polymer concentrations are shown in . As the total polymer concentrations reduced from 0.24 to 0.192, to 0.144 and to 0.096, the linear nanofibers E1 () changed to the typical spindles-on-a-string hybrids E2 (), to the typical beads-on-a-string hybrids E3 (), and to aggregated particles E4 (), respectively. The formation of aggregated particles E4 in suggested the incomplete exhaustion of solvent during the EHDA processes and thus the re-bound on the collector, whereas, other EHDA products in were directly resulted from the solidifiable working fluids.
Figure 3. SEM images of the prepared EHDA products (a–d) and the relationships between the total polymer (EC and PVP) contents in the working fluids and the sizes of resultant EHDA products: (a–d) SEM images of EHDA products E1, E2, E4, and E4, respectively; (e) the relationships between the diameters of strings and polymeric contents; and (f) the relationships between the diameters of beads and polymeric contents.
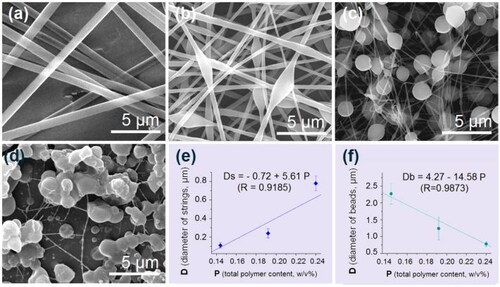
From , two trends are obvious. One is that the diameters of strings gradually reduced until disappeared in . The other is that the sizes of spindles (or beads) gradually increased. The linear nanofibers in were suggested to have a size of both strings and ‘beads’, then the change trends can be quantitatively built. Shown in is the gradual increase of the diameters of strings (Ds) with the increase of total polymer contents (P) in the working fluids, Ds = −0.72 + 5.61 P (R = 0.9185). The quantitative relationship indicating the gradual decrease of beads’ size (Db) with the increase of total polymer contents (P) in the working fluids is regressed as Db = 4.27 − 14.58 P (R = 0.9873) in . Although these phenomena are frequently reported for a wide variety of polymeric solutions in many publications, almost all of them provided only qualitative narrations because that researchers always cared only about the resultant linear nanofibers. Here, the disclosed quantitative process-morphology relationships should be useful for the intentional manipulation of the final EHDA products’ shapes and in turn their functional performances.
The inner structure properties of the three resultant EHDA products were achieved through TEM observations, which are exhibited in . In , the gray levels gradually reduced from the central axis to the boundaries of nanofibers, which was a direct result of the different thickness at different places of the nanofibers. No separated particles or no uneven regions could be discerned, suggesting that the nanofibers E1 were homogeneous composites, which often called solid dispersion in pharmaceutics [Citation58]. In , both the string and the spindle had their owns’ uniform gray levels, suggesting that they are homogeneous composites but had different thickness. Similarly, the EHDA beads-on-a-string products E3 are hybrids of strings and beads, and all the components were homogeneously mixed together, as suggested by their uniform gray levels. No matter how complicated the morphologies were, all the EHDA products were generated in a single step and in a straightforward manner from drug/polymer blended solutions. Today, inorganic nanoparticles and their hybrids with organic ingredients are popular for developing functional nanomaterials [Citation59–61]. The situations would be more complicated provided the inorganic nanoparticles were explored to be further treated via EHDA techniques, and SEM/TEM mapping methods would be useful tools for disclosing their distributions [Citation62,Citation63].
Figure 4. TEM images of the prepared EHDA products: (a) straight nanofibers E1; (b) spindles-on-a-string E2; and (c) beads-on-a-string E3.
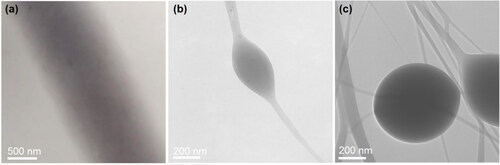
Based on the observations of working processes and the evaluations of the resultant EHDA products, the formation mechanisms of the composites with different morphologies can be deduced in . Shown in , the instable regions can be further divided into three stages for differentiating their different bending and weeping processes. Generally, it is regarded that the elasticity of the working fluids (having a close relationship with their viscosity and surface tensions) determines the final EHDA products. For working fluids having none elasticity (such as water) to lower elasticity, and to adequate elasticity, the working fluids would correspondingly break into round droplets, form spindles-on-a-string hybrids, form beads-on-a-string hybrids, and solid nanofibers, as shown in .
Figure 5. Mechanisms for controllable fabrications of EHDA products with various morphologies: (a) the divisions of three stages of electrospinning during the instable bending and weeping processes; (b) a general trend of morphologies change with the treated working fluids’ elasticity; (c) the balance between the elasticity/surface tension and the repelling forces for drawing the working fluids; (d) the gradual change procedures for EHDA products E1, E2, and E3.
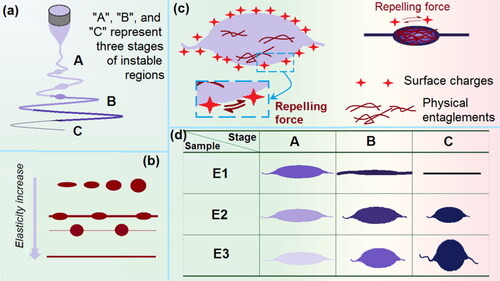
For the fluid jet just following the straight fluid jet, the elongated ellipses are frequently formed due to the interactions of several different forces. To simply the explanations, some forces aren’t taken into consideration, such as the gravity, the repelling forces between two adjacent bending circles, and the attraction between positive and negative poles. The first force is the drawing forces exerted on the fluid jest. As indicated in , the repelling forces due to the surface distribution of charges were responsive for the electric drawing on the fluid jets. However, the elasticity of fluid (and also the surface tension) would retard the drawing to keep the previous unspread state of the fluid. Whereas the elasticity has a positive relationship with the physical entanglements of polymeric molecules within the working fluid. A larger molecular weight and a higher concentration would result in more entanglements, and in turn a bigger viscosity or elasticity apparently.
Thus, the states of different stages of the working fluids for preparing E1 to E3 can be diagrammed in . For the highest polymeric concentration for fabricating E1, there are adequate elasticity to resist the strong electric drawing or repelling forces in stage A. The balance would result in the spreading of the elongated ellipses fluid jets into ‘linear’ format fluid jets. In stage B, some solvent is evaporated and some surface charges escape to the environment. Thus, on one hand, the repelling force would decrease. On the other hand, the elasticity is further increased to keep the previous ‘linear’ format state. In stage C, the electric drawing is stopped, and the solid nanofibers are deposited on the collector.
As for the fluids for preparing E2, the lower polymer concentration would make the presence of elongated ellipse fluid jets sustain a longer time period to go into the stages B and C. Meanwhile, the reduction of drawing forces due to the escape of electric charges on the surface of fluid jet would be unable to spread these ellipse jets into linear formats. However, the ‘linear’ section fluid jets can experience more electrical drawing, and thus, the strings in E2 are smaller than the nanofibers in E1. When the polymer concentration is further reduced for preparing E3, the further reduction of elasticity would project the role of surface tension, which always draw the fluid into a ‘round’ droplet. Thus, when the fluid jets go into the stages B and C, the reduced electric repelling forces and the formations of semisolid substances on the surface of ellipse fluid jets would round the beads. However, the linear fluid jets would be subjected even longer time period drawing. Thus, an even smaller string and rounded particles present in the EHDA products E3.
3.2. The physical state of the components in the fibrous nanocomposites
The raw crystalline state of KET powders determines that KET is poorly water-soluble. In pharmaceutics, many poorly water-soluble drugs are experienced a phase transition for developing novel dosage forms. Electrospinning is one of the most useful methods for transferring the crystalline powders of poorly water-soluble drugs into an amorphous state [Citation58].
In this study, XRD patterns of the raw KET, EC, and PVP powders and also their EHDA products E1 to E3 were achieved, which are included in . Just as anticipated, the raw KET powders had many sharp Bragg peaks in its patterns, suggesting a crystalline state. PVP and EC are amorphous polymers, as indicated by the blunt halos in their XRD patterns. In the XRD patterns of EHDA products E1 to E3, all the sharp peaks of KET totally disappeared, giving a hint that the drug KET presented in the EHDA products in an amorphous state. The extremely fast drying process of electrospinning have propagated the homogeneous co-present state of KET and the two polymers in the working fluids into the solid products, i.e. a molecular scale distribution of KET within the EC and PVP matrices. Later, the polymeric chains of PVP and EC would effectively retard the encountering and re-crystallization of KET molecules, and in turn the possible phase separations and formations of nano crystals within the EHDA products.
Figure 6. XRD patterns of the starting raw components (i.e. EC, PVP, and KET) and their different kinds of EHDA products E1, E2, and E3.
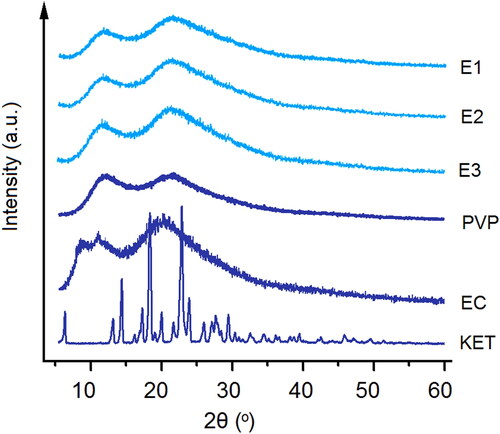
In the medicated nanocomposites, the fine compatibility between the active ingredient and the polymeric matrices is very important for their stability, and in turn their storage and shipping for further developments of commercial products. FTIR spectra are frequently exploited to disclose the functional groups in an organic molecule and also the secondary interactions between different molecules [Citation64–69]. In this study, FTIR tests were carried out to achieve the spectra of the raw components and resultant EHDA composites. These data are included in . KET has two characteristic peaks for its C = O groups. From its molecular formula, it is clear that KET molecules are easy to form dimers due to the hydrogen bonding. The hydrogen bonding would result in the red shift of the peaks at 1697 to 1655 cm−1. The characteristic peaks for EC and PVP are 1067 and 1662 cm−1. These characteristic peaks are also obvious in the spectra of E1, E2, and E3, suggesting the co-existence of PVP and EC in the three EHDA products. However, the characteristic peaks for KET, both 1697 and 1655 cm−1, disappeared from the spectra of E1 to E3. Meanwhile, other peaks at finger region also reduced to almost disappearance. These phenomena should be attributed to the favorite secondary interactions between KET molecules and the polymeric carriers. These interactions include the hydrogen bindings, hydrophobic interactions (between the long carbon chains of EC and PVP and the benzene rings in KET molecules), and also the Van der Waals forces and electrostatic interactions. It is just also these interactions that the KET is highly compatible with the polymeric matrices and the stability of three kinds of EHDA composites can be ensured.
3.3. The functional performance of the EHDA products with various morphologies
As anticipated, the measured encapsulation efficiency (EE%) values for the three kinds of EHDA products E1, E2, and E3 were 99.7 ± 4.2, 100.3 ± 3.4, and 99.7 ± 2.7%, respectively. The electrospinning processes, regardless of the polymeric concentrations in the working fluids, were finished in several decades of milliseconds, leaving almost no time for the drug KET molecules to escape from the electrospun solidification procedures. Thus, these physical drying processes always have an EE% value around 100%, an advantage over many other nano pharmaceutical methods that the EE% values are often smaller than 50%, such as molecular self-assembly.
The KET release profiles from the linear fibrous E1, the spindles-on-a-string E2, and the beads-on-a-string E3 are included in (showing the accumulative release percentage vs. sampling time point) and (showing the time needed by reaching a certain percentage drug release). In , it is clear that all the three EHDA products were able to release over 30% of the loaded KET molecules. In pharmaceutics, this initial burst release is viewed as an abnormal phenomenon. It is undesired for drug sustained release dosage form for the possibility of resulting in a high and toxic drug blood concentration. However, for bi-stage release, it is fine for eliminating the patients’ symptoms and achieving a fast therapeutic action. The key thing is to controllably manipulate the drug release rate below a certain value. Shown in , the time needed for reached a 30% release was 0.88, 0.82, and 0.93 h for EHDA products E1, E2, and E3, respectively. In other words, all the EHDA products were able to release over 30% of the loaded KET. This should be mainly attributed to the surface distribution of KET on the EHDA products but also had a relationship with the additions of water-soluble PVP in the EC matrix and the amorphous state of KET within the EHDA products.
Figure 8. In vitro dissolution tests: (a) the accumulative release vs. release time periods; and (b) the time needed for reached a certain percentage release (30, 50, and 95%).
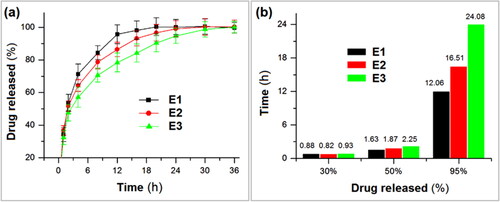
For a half release of the loaded KET in E1, E2, and E3, 1.63, 1.87, and 2.25 h was needed, respectively (). However, for reaching a 95% drug release, 12.06, 16.51, and 24.08 h was needed, respectively. From a standpoint of drug sustained release, the performance has an order of the linear fibrous E1 < the spindles-on-a-string E2 < the beads-on-a-string E3. The final (100–95%) release is often thought be useless for therapy due to the small drug release through a relatively longer time period can’t ensure an effective therapeutic blood drug concentration.
Shown in are diagrams about the drug controlled release mechanisms for EHDA products E1 to E3. These linear relationships were regressed according to the Peppas equation [Citation70], i.e. Q = ktn, where Q, k, t, and n represent the accumulative drug release percentage, a constant, sampling time point, and an index. It is well-known that a value larger than 0.89, between 0.45 and 0.89, and smaller than 0.45 indicates that erosion, a combination of erosion and Fickian diffusion, and a Fickian diffusion drug controlled release mechanism, respectively. The regressed equations for the linear nanofibers E1, the spindles-on-a-string E2 and the beads-on-a-string E3 are LogQ1 = 1.5851 + 0.3684 Logt (R = 0.9761) (), LogQ2 = 1.6014 + 0.3038Logt (R = 0.9895) (), and LogQ3 = 1.5522 + 0.3117Logt (R = 0.9912) (), respectively. All the n values (i.e. 0.3684 for E1, 0.3038 for E2, and 0.3117 for E3) are smaller than the critical value of 0.45, indicating that the drug KET molecules were released from the linear nanofibers, the spindles-on-a-string E2 and the beads-on-a-string E3 similarly through a Fickian diffusion mechanism. This can be anticipated because that EC, as the main matrix in the EHDA products, is insoluble in water. The free encapsulated KET molecules must be experiences the following procedure: the water molecules penetrate into the EHDA products, the PVP molecules are dissolved and some KET molecules are also dissolved, the dissolved PVP and KET molecules diffuse from the swollen EHDA products to the bulk solution, the residue KET molecules in the swollen EHDA products diffuse to the outer bulk solution through the routed by the previously dissolved KET and PVP molecules. These procedures have nothing to do with the inner structure of the EHDA products, but have a close relationship with the shortest lengths for water molecules penetration and KET molecules diffusion, i.e. the size of the EHDA products and their morphologies [Citation47].
Figure 9. Drug release mechanisms from the different types of EHDA composites based on the Peppas equation: (a) fibrous composites E1; (b) spindles-on-a-string composites E2; and (c) beads-on-a-string composites E3.
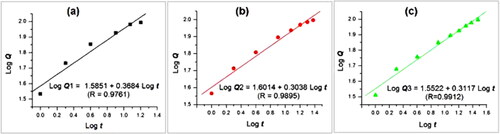
Electrospinning, as a useful nano technique for synthesizing and fabricating polymeric nanocomposites, is expanding its biomedical applications in almost all the scientific branches associated with human health [Citation71–73]. Based on the polymers’ properties, many drug controlled release profiles, such as immediate/pulsatile release, sustained release, delayed release, colon-targeted release, and multiple-phase release can be achieved through manipulating the inner structures of nanofibers and also the assembly formats [Citation74,Citation75]. Different from previous studies about drug release from EHDA products, this manuscript demonstrates for the first time that the shapes and morphologies of EHDA products are able to play their important roles in manipulating the drug release behaviors. Although the drug KET and the polymeric matrices PVP and EC are common in pharmaceutics, and the drug release mechanisms were verified to be similar Fickian diffusion mechanism, the composites in the form of linear nanofibers (as recommended by numerous publications) showed a worse performance of providing bi-stage drug controlled release profiles than those nonlinear products, i.e. spindles-on-a-string and beads-on-a-string hybrids. Polymeric nanofibers can be exploited as templates for producing ceramics and metal nanofibers [Citation76]. Based on the similar strategies, ceramics and metals spindles-on-a-string and beads-on-a-string hybrids can be developed and functionalized for a wide variety of functional applications in the near future. By the way, the present investigation is from an angle of functional nanomaterials’ shapes and morphologies. The popular elements, such as nanofibers [Citation77,Citation78], new kinds of polymers [Citation79,Citation80], multi-chamber structures (particularly core-shell [Citation81] and Janus structures [Citation82]), and their integrated combinations would make the functional nanocomposites more prosperous.
4. Conclusions
In this study, polymers concentrations in the working fluids were explored as a dominant factor to manipulate the morphologies of resultant EHDA products from linear nanofibers to spindles-on-a-string, and beads-on-a-string hybrids. SEM and TEM results indicated that all the EHDA products are homogeneous composites without any inner discerned solid phase separation. Meanwhile, it is disclosed that the diameters of strings gradually decreased whereas the diameters of particles increased along with the dilutions of working fluids. The mechanisms for controllable preparations of these morphologies are proposed. XRD tests demonstrated that the poorly water-soluble model drug KET distributed all over the PVP and EC matrices in an amorphous state, which is favorable for dissolution and design of controlled release. FTIR results indicated that the drug KET was compatible with the polymeric carriers. In vitro dissolution tests verified that all the three EHDA products with various morphologies were able to provide the bi-stage drug controlled release profiles, i.e. a fast release for rapid action to eliminate the symptoms, followed by a sustained release, which is useful for keeping a longer time period of therapeutic blood concentration, and in turn the conveniences for the patients to reduce oral administration times. However, the beads-on-a-string hybrids E3 showed the best functional performances for providing the longest extended KET release time period. Based on the disclosed process-morphology-performance relationship disclosed here, many novel functional nanocomposites with various shapes can be further designed and fabricated using EHDA processes for applications in a series of applied scientific fields, such as energy, environments, hydrogen, medicine, and tissue engineering.
Author contributions
All authors made a major contribution to this work, including the conception, study design, execution, data collection, analysis, and interpretation; participated in drafting, revising, and reviewing the article; gave final approval of the version to be published; have agreed on the journal to which the article has been submitted; and agree to be accountable for all aspects of the work.
Disclosure statement
No potential conflict of interest was reported by the author(s).
Data availability statement
The original contributions presented in the study are included in the article, further inquiries can be directed to the corresponding authors.
Additional information
Funding
Notes on contributors
Wenjian Gong
Wenjian Gong is presently a Master student under the supervisions of Prof. Deng-Guang Yu, he majors in nanomaterials and drug delivery systems.
Wei Yang
Wei Yang is a PhD students under the supervisions of Prof. Ping Liu, he majors in nanomaterials and drug delivery systems.
Jianfeng Zhou
Jianfeng Zhou is presently a PhD under the supervisions of Prof. Deng-Guang Yu, he majors in nanomaterials, drug delivery systems and biomedical devices.
Shuping Zhang
Shuping Zhang is a full professor in University of Shanghai for Science and Technology. Her research interests include green chemistry, marine products and nano engineering.
Deng-Guang Yu
Deng-Guang Yu is a full professor in University of Shanghai for Science and Technology. His research interests include drug delivery and electrohydrodynamic atomization methods, such as electrospinning, electrospraying and e-jet printing.
Ping Liu
Ping Liu is a full professor in University of Shanghai for Science and Technology. Her research interests include drug delivery clinic applications.
References
- Wang JY, Yu YQ, Zhang CQ, et al. New advances in diagnosis and treatment of nano drug delivery systems across the blood-brain barrier. Nanocomposites. 2023;9(1):116–127. doi: 10.1080/20550324.2023.2256466.
- Qosim N, Majd H, Huo S, et al. Hydrophilic and hydrophobic drug release from core (polyvinylpyrrolidone)-sheath (ethyl cellulose) pressure-spun fibers. Int J Pharm. 2024;654:123972. doi: 10.1016/j.ijpharm.2024.123972.
- Zhou J, Che Y, Liu Y, et al. Electrospun meicated gelatin/polycaprlactone Janus fibers for photothermal-chem combined thrapy of liver cancer. Int J Biolog Macromol. 2024;269:132113. doi: 10.1016/j.ijbiomac.2024.132113.
- Zhan Y, Zhang S. Design of novel PLK4 inhibitors as TRIM37-amplified breast cancer drugs using 3D-QSAR, molecular docking, and molecular dynamics simulation methods. Mol Simul. 2024;50(7–9):571–587. doi: 10.1080/08927022.2024.2331237.
- Audtarat S, Hongsachart P, Dasri T, et al. Green synthesis of silver nanoparticles loaded into bacterial cellulose for antimicrobial application. Nanocomposites. 2022;8(1):34–46. doi: 10.1080/20550324.2022.2055375.
- Sun CC, Zhi HX, Li H, et al. Synthesis, characterization and antimicrobial study of cinnamic acid functionalized Ag nanoparticles. Nanocomposites. 2022;8(1):95–101. doi: 10.1080/20550324.2022.2066827.
- Yu DG, Zhao P. The key elements for biomolecules to biomaterials and to bioapplications. Biomolecules. 2022;12(9):1234. doi: 10.3390/biom12091234.
- Liao Q, Kim EJ, Tang Y, et al. Rational design of hyper-crosslinked polymers for biomedical applications. J Polym Sci. 2023;62(8):1517–1535. doi: 10.1002/pol.20230270.
- Tan PK, Kuppusamy UR, Chua KH, et al. Emerging strategies to improve the stability and bioavailability of insulin: an update on formulations and delivery approaches. Curr Drug Deliv. 2023;20(8):1141–1162. doi: 10.2174/1567201820666221102094433.
- Huang XY, Jiang WL, Zhou JF, et al. The applications of ferulic-acid-loaded fibrous films for fruit preservation. Polymers. 2022;14(22):4947. doi: 10.3390/polym14224947.
- Handayani M, Suwaji BI, Asih GIN, et al. In-situ synthesis of reduced graphene oxide/silver nanoparticles (rGO/AgNPs) nanocomposites for high loading capacity of acetylsalicylic acid. Nanocomposites. 2022;8(1):74–80. doi: 10.1080/20550324.2022.2054210.
- Murugesan R, Raman S. Recent trends in carbon nanotubes based prostate cancer therapy: a biomedical hybrid for diagnosis and treatment. Curr Drug Deliv. 2022;19(2):229–237. doi: 10.2174/1567201818666210224101456.
- Du CC, Huang W. Progress and prospects of nanocomposite hydrogels in bone tissue engineering. Nanocomposites. 2022;8(1):102–124. doi: 10.1080/20550324.2022.2076025.
- Lu HJ, Zhao Y, Qin SC, et al. Fluorine substitution tunes the nanofiber chirality of supramolecular hydrogels to promote cell adhesion and proliferation. Adv Fiber Mater. 2023;5(1):377–387. doi: 10.1007/s42765-022-00232-w.
- Yang Y, Zhang R, Liang Z, et al. Application of electrospun drug-loaded nanofibers in cancer therapy. Polymers. 2024;16(4):504. doi: 10.3390/polym16040504.
- Bai YB, Liu YA, Lv H, et al. Processes of electrospun polyvinylidene fluoride-based nanofibers, their piezoelectric properties, and several fantastic applications. Polymers. 2022;14(20):4311. doi: 10.3390/polym14204311.
- Al-Hazeem NZ, Ahmed NM, Jafri MZM, et al. The effect of deposition angle on morphology and diameter of electrospun TiO2/PVP nanofibers. Nanocomposites. 2021;7(1):70–78. doi: 10.1080/20550324.2021.1917836.
- Chen S, Zhou JF, Fang BY, et al. Three EHDA processes from a detachable spinneret for fabricating drug fast dissolution composites. Macro Mater Eng. 2023;309(4):2300361. doi: 10.1002/mame.202300361.
- Ji YX, Zhao H, Liu H, et al. Electrosprayed stearic-acid-coated ethylcellulose microparticles for an improved sustained release of anticancer drug. Gels. 2023;9(9):700. doi: 10.3390/gels9090700.
- Sivan M, Madheswaran D, Hauzerova S, et al. AC electrospinning: impact of high voltage and solvent on the electrospinnability and productivity of polycaprolactone electrospun nanofibrous scaffolds. Mater Today Chem. 2022;26:101025. doi: 10.1016/j.mtchem.2022.101025.
- Sivan M, Madheswaran D, Valtera J, et al. Alternating current electrospinning: the impacts of various high-voltage signal shapes and frequencies on the spinnability and productivity of polycaprolactone nanofibers. Mater Des. 2022;213:110308. doi: 10.1016/j.matdes.2021.110308.
- Yu DG, Xu L. Impact evaluations of articles in current drug delivery based on web of science. Curr Drug Deliv. 2024;21(3):360–367. doi: 10.2174/1567201820666230508115356.
- Peng W, Wang L, Zhang M, et al. Biodegradable flexible conductive film based on sliver nanowires and PLA electrospun fibers. J Appl Polym Sci. 2024;141(22):e55433. doi: 10.1002/app.55433.
- Li T, Ding X, Tian L, et al. The control of beads diameter of bead-on-string electrospun nanofibers and the corresponding release behaviors of embedded drugs. Mater Sci Eng C Mater Biol Appl. 2017;74:471–477. doi: 10.1016/j.msec.2016.12.050.
- Brimo N, Serdaroğlu DÇ, Uysal B. Comparing antibiotic pastes with electrospun nanofibers as modern drug delivery systems for regenerative endodontics. Curr Drug Deliv. 2022;19(9):904–917. doi: 10.2174/1567201819666211216140947.
- Li DM, Cheng YY, Luo YX, et al. Electrospun nanofiber materials for photothermal interfacial evaporation. Materials. 2023;16(16):16165676. doi: 10.3390/ma16165676.
- Li DM, Yue GC, Li S, et al. Fabrication and applications of multi-fluidic electrospinning multi-structure hollow and core-shell nanofibers. Engineering. 2022;13:116–127. doi: 10.1016/j.eng.2021.02.025.
- Zhang Y, Lu Y, Li Y, et al. Poly(glutamic acid)-engineered nanoplatforms for enhanced cancer phototherapy. Curr Drug Deliv. 2024;21(3):326–338. doi: 10.2174/1567201820666230116164511.
- Tabakoglu S, Kołbuk D, Sajkiewicz P. Multifluid electrospinning for multi-drug delivery systems: pros and cons, challenges, and future directions. Biomater Sci. 2022;11(1):37–61. doi: 10.1039/d2bm01513g.
- Mao H, Zhou J, Yan L, et al. Hybrid films loaded with 5-fluorouracil and reglan for synergistic treating colon cancer through an asynchronous dual-drug delivery. Front Bioeng Biotechnol. 2024;12:1398730. doi: 10.3389/fbioe.2024.1398730.
- Kang S, Hou SC, Chen XW, et al. Energy-saving electrospinning with a concentric Teflon-core rod spinneret to create medicated nanofibers. Polymers. 2020;12(10):2421. doi: 10.3390/polym12102421.
- Qian CH, Liu YB, Chen S, et al. Electrospun core-sheath PCL nanofibers loaded with nHA and simvastatin and their potential bone regeneration applications. Front Bioeng Biotechnol. 2023;11:1205252. doi: 10.3389/fbioe.2023.1205252.
- Shen SF, Zhu LF, Liu J, et al. Novel core-shell fiber delivery system for synergistic treatment of cervical cancer. J Drug Deliv Sci Technol. 2020;59:101865. doi: 10.1016/j.jddst.2020.101865.
- Xu L, Li Q, Wang H, et al. Electrospun multi-functional medicated tri-section Janus nanofibers for an improved anti-adhesion tendon repair. Chem Eng J. 2024;492:152359. doi: 10.1016/j.cej.2024.152359.
- Song W, Tang Y, Qian C, et al. Electrospinning spinneret: a bridge between the visible world and the invisible nanostructures. Innovation. 2023;4(2):100381. doi: 10.1016/j.xinn.2023.100381.
- Guler E, Nur Hazar-Yavuz A, Tatar E, et al. Oral empagliflozin-loaded tri-layer core-sheath fibers fabricated using tri-axial electrospinning: enhanced in vitro and in vivo antidiabetic performance. Int J Pharm. 2023;635(25):122716. doi: 10.1016/j.ijpharm.2023.122716.
- Zhang S, Yang W, Gong W, et al. Recent progress of electrospun nanofibers as burning dressings. RSC Adv. 2024;14(20):14374–14391. doi: 10.13039/501100007129.
- Yao ZC, Zhang CC, Xing Z, et al. Controlled engineering of multifunctional porous structures using tri-needle co-axial electrohydrodynamic flow and sacrificial media. Chem Eng J. 2022;429:132221. doi: 10.1016/j.cej.2021.132221.
- Zhou JF, Yi T, Zhang ZY, et al. Electrospun janus core (ethyl cellulose//polyethylene oxide) @ shell (hydroxypropyl methyl cellulose acetate succinate) hybrids for an enhanced colon-targeted prolonged drug absorbance. Adv Compos Hybrid Mater. 2023;6(6):189. doi: 10.1007/s42114-023-00766-6.
- Chen X, Liu Y, Liu P. Electrospun core-sheath nanofibers with a cellulose acetate coating for the synergistic release of zinc ion and drugs. Mol Pharm. 2023;21(1):173–182. doi: 10.1021/acs.molpharmaceut.3c00703.
- Zhou J, Pan H, Gong W, et al. Electrosprayed eudragit RL100 nanoparticles with janus polyvinylpyrrolidone patches for multiphase release of paracetamol. Nanoscale. 2024;16(17):8573–8582. doi: 10.1039/D4NR00893F.
- Wang ML, Hou JS, Yu DG, et al. Electrospun tri-layer nanodepots for sustained release of acyclovir. J Alloys Compd. 2020;846:156471. doi: 10.1016/j.jallcom.2020.156471.
- Zhao P, Zhou K, Xia Y, et al. Electrospun trilayer eccentric janus nanofibers for a combined treatment of periodontitis. Adv Fiber Mater. 2024;5:1–21. doi: 10.1007/s42765-024-00397-6.
- Liu Y, Chen X, Lin X, et al. Electrospun multi-chamber core–shell nanofibers and their controlled release behaviors: a review. WIREs Nanomed Nanobiotechnol. 2024;16:1954. doi: 10.1002/wnan.1954.
- Yao ZC, Zhang CC, Ahmad Z, et al. Designer fibers from 2D to 3D-simultaneous and controlled engineering of morphology, shape and size. Chem Eng J. 2018;334:89–98. doi: 10.1016/j.cej.2017.10.033.
- He H, Wu MA, Zhu JW, et al. Engineered spindles of little molecules around electrospun nanofibers for biphasic drug release. Adv Fiber Mater. 2022;4(2):305–317. doi: 10.1007/s42765-021-00112-9.
- Yu DG, Gong W, Zhou J, et al. Engineered shapes using electrohydrodynamic atomization for an improved drug delivery. Wiley Interdiscip Rev Nanomed Nanobiotechnol. 2024;16(3):e1964. doi: 10.1002/wnan.1964.
- Xu L, He H, Du Y, et al. Electrosprayed core (cellulose acetate)-shell (polyvinylpyrrolidone) nanoparticles for smart acetaminophen delivery. Pharmaceutics. 2023;15(9):2314. doi: 10.3390/pharmaceutics15092314.
- Zhu Y, Zhang C, Liang Y, et al. Advanced postoperative tissue antiadhesive membranes enabled with electrospun nanofibers. Biomater Sci. 2024;12(7):1643–1661. doi: 10.1039/d3bm02038j.
- Duan H, Chen HN, Chen R, et al. A novel electrospun nanofiber system with PEGylated paclitaxel nanocrystals enhancing the transmucus permeability and in situ retention for an efficient cervicovaginal cancer therapy. Int J Pharm. 2024;650:123660. doi: 10.1016/j.ijpharm.2023.123660.
- Wang ML, Ge RL, Zhang FY, et al. Electrospun fibers with blank surface and inner drug gradient for improving sustained release. Biomater Adv. 2023;150:213404. doi: 10.1016/j.bioadv.2023.213404.
- Jiang X, Zeng Y-E, Li C, et al. Enhancing diabetic wound healing: advances in electrospun scaffolds from pathogenesis to therapeutic applications. Front Bioeng Biotechnol. 2024;12:1354286. doi: 10.3389/fbioe.2024.1354286.
- Wen RY, Gao ZH, Luo L, et al. Sandwich-structured electrospun all-fluoropolymer membranes with thermal shut-down function and enhanced electrochemical performance. Nanocomposites. 2022;8(1):64–73. doi: 10.1080/20550324.2022.2057661.
- Lv H, Liu Y, Zhou J, et al. Efficient piezophotocatalysis of ZnO@PVDF coaxial nanofibers modified with BiVO4 and Ag for the simultaneous generation of H2O2 and removal of pefloxacin and Cr(VI) in water. Chem Eng J. 2024;484:149514. doi: 10.1016/j.cej.2024.149514.
- Sun L, Zhou JF, Chen YN, et al. A combined electrohydrodynamic atomization method for preparing nanofiber/microparticle hybrid medicines. Front Bioeng Biotechnol. 2023;11:1308004. doi: 10.3389/fbioe.2023.1308004.
- Wasilewska K, Winnicka K. Ethylcellulose–a pharmaceutical excipient with multidirectional application in drug dosage forms development. Materials. 2019;12(20):3386. doi: 10.3390/ma12203386.
- Grant JJ, Pillai SC, Perova TS, et al. Electrospun fibres of chitosan/PVP for the effective chemotherapeutic drug delivery of 5-fluorouracil. Chemosensors. 2021;9(4):70. doi: 10.3390/chemosensors9040070.
- Huang C, Wang M, Yu S, et al. Electrospun fenoprofen/polycaprolactone @ tranexamic acid/hydroxyapatite nanofibers as orthopedic hemostasis dressings. Nanomaterials. 2024;14(7):646. doi: 10.3390/nano14070646.
- Mostafa AM, Mwafy EA, Awwad NS, et al. Linear and nonlinear optical studies of Ag/Zn/ZnO nanocomposite thin film prepared by pulsed laser deposition technique. Radiat Phys Chem. 2021;179:109233. doi: 10.1016/j.radphyschem.2020.109233.
- ElFaham MM, Mostafa AM, Mwafy EA. The effect of reaction temperature on structural, optical and electrical properties of tunable ZnO nanoparticles synthesized by hydrothermal method. J Phys Chem Solids. 2021;154:110089. doi: 10.1016/j.jpcs.2021.110089.
- Mostafa AM. The enhancement of nonlinear absorption of Zn/ZnO thin film by creation oxygen vacancies via infrared laser irradiation and coating with Ag thin film via pulsed laser deposition. J Mol Struct. 2021;1226(B):129407. doi: 10.1016/j.molstruc.2020.129407.
- Mostafa AM, Mwafy EA, Toghan A. ZnO nanoparticles decorated carbon nanotubes via pulsed laser ablation method for degradation of methylene blue dyes. Colloids Surf A. 2021;627(20):127204. doi: 10.1016/j.colsurfa.2021.127204.
- Khattab TA, Gabr AM, Mostafa AM, et al. Luminescent plant root: a step toward electricity-free natural lighting plants. J Mol Struct. 2019;1176(15):249–253. doi: 10.1016/j.molstruc.2018.08.101.
- Mostafa AM, Lotfy VF, Mwafy EA, et al. Influence of coating by Cu and Ag nanoparticles via pulsed laser deposition technique on optical, electrical and mechanical properties of cellulose paper. J Mol Struct. 2020;1203:127472. doi: 10.1016/j.molstruc.2019.127472.
- Mwafy EA, Mostafa AM. Tailored MWCNTs/SnO2 decorated cellulose nanofiber adsorbent for the removal of Cu (II) from waste water. Radiat Phys Chem. 2020;177:109172. doi: 10.1016/j.radphyschem.2020.109172.
- El-Messery TM, Mwafy EA, Mostafa AM, et al. Spectroscopic studies of the interaction between isolated polyphenols from coffee and the milk proteins. Surf Interfaces. 2020;20:100558. doi: 10.1016/j.surfin.2020.100558.
- Darwish WM, Darwish AM, Al-Ashkar EA. Synthesis and nonlinear optical properties of a novel indium phthalocyanine highly branched polymer. Polym Adv Technol. 2015;26(8):1014–1019. doi: 10.1002/pat.3520.
- Darwish WM, Darwish AM, Al-Ashkar EA. Indium(III) phthalocyanine eka-conjugated polymer as high-performance optical limiter upon nanosecond laser irradiation. High Perform Polym. 2016;28(6):651–659. doi: 10.1177/0954008315593616.
- Lv Q, Ma X, Zhang C, et al. Nanocellulose-based nanogenerators for sensor applications: a review. Int J Biolog Macromol. 2024;259:129268.
- Peppas NA. Analysis of Fickian and non-Fickian drug release from polymers. Pharm Acta Helv. 1985;60(4):110–111.
- Zhang X, Shi XT, Gautrot JE, et al. Nanoengineered electrospun fibers and their biomedical applications: a review. Nanocomposites. 2021;7(1):1–34. doi: 10.1080/20550324.2020.1857121.
- Chen XJ, Yan S, Wen SS, et al. Chelating adsorption-engaged synthesis of ultrafine iridium nanoparticles anchored on N-doped carbon nanofibers toward highly efficient hydrogen evolution in both alkaline and acidic media. J Colloid Interface Sci. 2023;641:782–790. doi: 10.1016/j.jcis.2023.03.097.
- Song N, Ren SY, Zhang Y, et al. Confinement of Prussian blue analogs boxes inside conducting polymer nanotubes enables significantly enhanced catalytic performance for water treatment. Adv Funct Mater. 2022;32(34):2204751. doi: 10.1002/adfm.20220475.
- Ejeta F, Gabriel T, Joseph NM, et al. Formulation, optimization and in vitro evaluation of fast disintegrating tablets of salbutamol sulphate using a combination of superdisintegrant and subliming agent. Curr Drug Deliv. 2022;19(1):129–141. doi: 10.2174/1567201818666210614094646.
- Lang Y, Wang B, Chang M-W, et al. Sandwich-structured electrospun pH-responsive dental pastes for anti-caries. Colloids Surf A. 2023;668:131399. doi: 10.1016/j.colsurfa.2023.131399.
- Xu JQ, Zhong MX, Song N, et al. General synthesis of Pt and Ni co-doped porous carbon nanofibers to boost HER performance in both acidic and alkaline solutions. Chin Chem Lett. 2022;34(2):107359. doi: 10.1016/j.cclet.2022.03.082.
- Ji D, Lin Y, Guo X, et al. Electrospinning of nanofibres. Nat Rev Method Primers. 2024;4:1. doi: 10.1038/s43586-023-00278-z.
- Yan S, Qian Y, Haghayegh M, et al. Electrospun organic/inorganic hybrid nanofibers for accelerating wound healing: a review. J Mater Chem B. 2024;12(13):3171–3190. doi: 10.1039/D4TB00149D.
- Riaz Z, Baddi S, Gao FL, et al. Gallic acid-doped multifunctional hybrid hydrogel for antioxidant and antibacterial studies. Euro Polym J. 2024;206:112778. doi: 10.1016/j.eurpolymj.2024.112778.
- Zhang Y, Tang Y, Liao Q, et al. Silver oxide decorated urchin-like microporous organic polymer composites as versatile antibacterial organic coating materials. J Mater Chem B. 2024;12(8):2054–2069. doi: 10.1039/D3TB02619A.
- Shi Y, Zhang Y, Zhu L, et al. Tailored drug delivery platforms: stimulus-responsive core–shell structured nanocarriers. Adv Healthc Mater. 2024;13(1):2301726. doi: 10.1002/adhm.202301726.
- Zhang X, Yu N, Ren Q, et al. Janus nanofiber membranes with photothermal-enhanced biofluid drainage and sterilization for diabetic wounds. Adv Funct Mater. 2024;34:2315020. doi: 10.1002/adfm.202315020.