Abstract
The research project focuses on conducting an in vitro comparison between sodium alginate and chitosan electrospun nanofibers for moist wound management. These fabricated nanofibers were incorporated with levofloxacin (Lev) as a model drug to investigate their release behavior. The study included a comprehensive examination of the physicochemical, thermal, antibacterial, and cytocompatibility properties of these nanofibers. Both sodium alginate (SA-L) and chitosan (CS-L) nanofibers were successfully produced with a homogeneous and defect-free structure. XRD and FTIR analyses were conducted to validate the incorporation of Lev in the nanofibers. In terms of liquid absorption capacity, it was observed that sodium alginate nanofibers outperformed chitosan nanofibers, exhibiting absorption capacities of 5.9, 5.88, and 7.1 g/g for PBS, solution A, and DI, respectively, for SA-L, compared to 5.27, 4.42, and 5.3 g/g for CS-L nanofibers. This superior absorption ability in SA-L nanofibers may be attributed to the inherent gel-forming properties of alginate in aqueous environments. Furthermore, the release kinetics indicated that SA-L nanofibers exhibited faster drug release compared to CS-L nanofibers, which could be attributed to alginate’s natural gel-forming tendency in aqueous mediums. Both SA-L and CS-L nanofibers demonstrated remarkable efficacy against Staphylococcus aureus (S. aureus) and Pseudomonas aeruginosa (P. aeruginosa), with CS-L nanofibers displaying larger inhibition zones in agar diffusion tests due to chitosan’s inherent antibacterial properties. Moreover, cytocompatibility assessments using the HaCat cell line revealed that the prepared nanofibers were biocompatible and non-toxic. Interestingly, CS-L nanofibers exhibited superior cell proliferation when compared to SA-L nanofibers, potentially attributed to the inherent positively charged amine groups which enhance proliferation and migration of negatively charged keratinocytes.
1. Introduction
Textile fibers, once primarily used for clothing and basic household needs, have evolved significantly. In the past, these fibers were restricted to simple domestic uses. However, with the passage of time and scientific advancements, new textile fibers with enhanced properties have been developed. This has led to a broadening of their applications, extending beyond traditional uses to more advanced fields [Citation1,Citation2]. One notable area of expansion is in the medical sector, where textile fibers are now utilized in various products such as surgical gowns, sutures, non-woven meshes for hernia repair, and various types of dressings. Reflecting this growth, a 2022 market research report indicated that the medical textiles sector had a market value of USD 32,200 million. Furthermore, this sector is projected to experience a compound annual growth rate (CAGR) of 4.3% through 2030 [Citation3].
Wound dressings play a pivotal role in the healing process, and the market currently offers an extensive variety (>3000) of these products [Citation4,Citation5]. The choice of dressing is tailored to the specific requirements of the wound; for instance, wounds that exude more fluid necessitate more absorbent dressings [Citation6]. Notably, alginate microfiber-based dressings like Algisite™, Sorbsan™, and Kaltostat™ are renowned for their high absorbency [Citation7–9]. However, in terms of performance, these microfiber dressings are markedly different from nanofiber dressings. Microfiber wound dressings, though effective in various clinical settings, exhibit several disadvantages when compared to nanofiber dressings, particularly in structural and functional aspects. Microfibers have larger pore sizes, which may not be as effective in preventing microbial penetration, crucial for reducing infection risks in wound care. Additionally, nanofibers excel in fluid management due to their high surface area-to-volume ratio, enhancing exudate absorption and retention, thus promoting a moist healing environment. Nanofiber dressings also offer superior conformity and flexibility, better adherence to complex wound surfaces, and enhanced breathability and gas exchange. Furthermore, they can be engineered for advanced drug delivery and incorporate bioactive components like collagen and peptides, significantly improving therapeutic outcomes. These attributes make nanofiber dressings more suited for complex or chronic wound scenarios, where rapid healing and infection control are paramount [Citation10–12].
Numerous techniques have been employed to create polymeric nanofibers, including template synthesis, phase separation, self-assembly, drawing, and melt-blown spinning, among others. Techniques such as template synthesis, drawing, phase separation, and self-assembly face challenges in scaling up, whereas melt-blown spinning is hindered by limitations in polymer selection and the thermal degradation of polymers [Citation13]. Electrospinning stands out as a highly efficient and economical method for transforming polymers, sourced both naturally and synthetically, into ultrafine nanostructures. This technology is employed across a range of advanced applications. For instance, it is used in the field of food packaging, signifying its relevance in preserving and maintaining food quality [Citation14,Citation15]. In water processing [Citation16–20], electrospinning plays a pivotal role, contributing to various stages from filtration to purification. Additionally, this technology is integral in the development of battery separators and supercapacitors, underlining its importance in the energy sector [Citation21,Citation22]. In the realm of medical care, electrospinning has a significant impact [Citation23–27]. Researchers have extensively explored and reported the use of nanofiber-based dressings, crafted through this technique, for advanced wound care. These dressings have proven to be particularly beneficial in treating a variety of wounds, including diabetic wounds [Citation28], burn injuries [Citation29–31], and post-surgical wounds [Citation32–35].
Burn injuries, distinct from other types of wounds, necessitate specialized attention from healthcare professionals. The classification of burn wounds is contingent upon the extent of tissue damage incurred by thermal exposure, which in turn dictates the specific medical dressing needs. Notably, first-degree burns typically do not exhibit exudation, whereas second and third-degree burns are characterized by significant exudation. This requires the application of dressings that are capable of not only absorbing but also retaining the exudate, all the while preventing further tissue damage or lacerations [Citation36]. Treating burn injuries with nano-formulations has shown certain advantages, rapid and safer healing in burn injuries is achieved using nano-formulations with biocompatible, biodegradable polymer excipients. These formulations enhance healing by allowing precise control over drug release, either through manipulation of the matrix degradation rate or optimization of drug diffusion [Citation37].
Natural biopolymers have been reported to improve the skin’s wound healing. Among them, alginate and chitosan are readily used biomaterials and have been extensively used for the fabrication of burn wound dressings [Citation38]. Alginate is a linear polysaccharide, composed of β-d-mannuronate and α-l-guluronate building blocks [Citation39,Citation40]. Due to its hydrophilic nature, it is highly adopted for the fabrication of hemostatic and exudate-absorbing mats. Thus, providing rapid tissue granulation and re-epithelialization under moist environments [Citation41]. Owing to the good elasticity and exudate absorbing capacity of alginate hydrogels they are highly regarded for treating burn wounds. Alginate and its composite dressings are highly used in burn wound treatments [Citation42].
Chitosan stands out as the sole naturally occurring cationic polysaccharide [Citation38]. It is characterized by three reactive functional groups: a primary amine and two hydroxyl groups (either primary or secondary) per glucosamine unit, which are amenable to chemical modifications. Structurally, chitosan resembles the glycosaminoglycan elements of the extracellular matrix. This similarity, combined with its biocompatibility, biodegradability, antibacterial, and antioxidant properties, and mucoadhesive capabilities, makes chitosan a versatile material. However, its application in biomedical fields is somewhat constrained due to its limited solubility at the physiological pH of 7.4, despite being soluble in acidic aqueous environments. Additionally, chitosan’s antimicrobial efficacy is enhanced in acidic conditions, as a result of the interaction between its protonated amino groups and the anionic components of bacterial cells [Citation43,Citation44]. However, Alginate and chitosan are not capable of electrospinning individually due to insufficient chain entanglements and high viscosity. To overcome this problem synthetic carrier polymers can be considered [Citation41]. Poly (ethylene oxide) (PEO) is an excellent carrier polymer for electrospinning of otherwise nonspinnable polymers. PEO, a synthetic, linear, and hydrophilic polymer has gained prominence in various medical applications owing to its biocompatibility and inert nature, as cited in multiple studies [Citation31]. Selection PEO over its counterpart carrier polymer like polyvinyl alcohol (PVA) is based on the following factors, fibers formed with PEO as carrier tend to have smoother and more uniform morphology with less diameter variations [Citation45]. This could be since PEO generally have higher molecular weight as compared to PVA, which allows for better polymer chain entanglements, essential for forming continuous and stable jets during electrospinning, and as a resultant smoother and more uniform fibers are formed. Another factor for PEO selection over PVA is its solubility in aqueous as well as organic solvents making it a versatile polymers for making solutions with ideal viscosities and conductivities for electrospinning. As a contrary PVA is only soluble in water and limit it use with the hydrophobic drugs [Citation46].
This study demonstrated the development of electrospun nanofibers composed of alginate and chitosan, infused with the antibiotic levofloxacin. The focus of this research was to assess the effectiveness of these nanofibers in managing moist wounds through an in vitro evaluation. To thoroughly examine the properties of the prepared nanofibers, a series of comprehensive physicochemical analyses were employed. These included scanning electron microscopy (SEM) for microstructure observation, X-ray diffraction (XRD) for crystallography, Fourier transform infrared spectroscopy (FTIR) for chemical bonding analysis, thermogravimetric analysis (TGA) for thermal stability, and tests for liquid absorption capacity. Furthermore, the study delved into the functional aspects of the nanofibers, investigating their drug release dynamics, antibacterial efficacy, and their biocompatibility and cytotoxicity in a controlled environment. The latter was assessed using the WST-1 colorimetric assay for cell viability and the lactose dehydrogenase (LDH) assay for cellular damage. The comprehensive approach of this study aimed to provide a detailed understanding of the potential applications and effectiveness of levofloxacin-loaded alginate and chitosan nanofibers in modern wound care.
2. Materials and methods
2.1. Materials
Chitosan (CS) with a molecular weight of approximately 100–200 kDa was acquired from GTL Biotech China. Poly (ethylene oxide) (PEO), sodium alginate (SA) of medium viscosity, acetic acid, Triton X-100, and Levofloxacin (Lev) were obtained from Sigma-Aldrich. Distilled water served as the solvent for preparing the electrospinning solutions. All chemicals were used as received.
2.2. Preparation of electrospinning solutions
To prepare the Chitosan solution for electrospinning, 2 g of CS was dissolved in a 50% acetic acid solution in distilled water with constant stirring (300 rpm) at room temperature. Simultaneously, in a separate glass vial, 5 g of PEO was dissolved in distilled water under the same stirring conditions as the CS solution. Both solutions were stirred continuously for 24 h. Subsequently, the solutions were combined, with 8 parts of PEO mixed with 2 parts of Chitosan, and stirred until a homogeneous solution was achieved.
To prepare the alginate solution, 2 g of sodium alginate (SA) was dissolved in distilled water. Separately, a 5% (w/v) polyethylene oxide (PEO) solution was also prepared in distilled water. These solutions were then combined in an 8:2 ratio of PEO to SA in a separate container and mixed until a homogeneous solution was obtained. Subsequently, 1% Triton X-100 (surfactant) was added to the SA solution to facilitate its electrospinning process.
To the prepared electrospinning solutions of SA and CS, 5 µg/ml of Lev was added as a model drug before the electrospinning process.
2.3. Electrospinning process
The electrospinning process was conducted on FLUIDNATEK electrospinning machine (Model LE-100, Bionica, Spain) with a 30 kV high voltage system. The electrospinning solutions were loaded onto a 10 ml plastic syringe equipped with a 20-gauge stainless steel needle. A high voltage of 15 kV was applied between the needle tip and collector. The flow rate was adjusted to 0.4 ml/h using an electronic syringe pump (KDS-100, KD scientific, USA) and the tip-to-collector distance was adjusted to 150 mm. The collector was covered with butter paper for easy removal of the collected nanofiber web. The same electrospinning parameters were used for both CS and SA nanofibers. The fabricated nanofibers were named SA-L and CS-L.
2.4. Morphological assessment
Nanofiber morphology was studied using the FEI Quanta-200 Scanning electron microscope (SEM). Before characterization, nanofibers were coated with Gold using a sputter coater for 60 s. The nanofiber diameter was analyzed by ImageJ software version 1.4.3.
2.5. Physicochemical characterization
The chemical identification of the composite nanofiber was analyzed by Fourier transform infrared spectroscopy (FT/IR-6600•IRT-5200, Jaso, Japan), Crystal structure of the nanofibers was determined by X-ray diffraction (XRD, Miniflex 300, Rigaku Co. Ltd, Japan).
Thermal degradation of the sample was determined by thermogravimetric analysis (TGA, TG-8120, Rigaku, Japan). Thermal degradation was observed from 30 to 600 °C under an air atmosphere with a 10 °C/min rise in temperature.
2.6. Liquid absorption
The liquid absorption capability of the manufactured nanofibers was evaluated using phosphate-buffered saline (PBS), distilled water, and solution A. In summary, a 1 cm2 nanoweb sample was weighed using a four-digit analytical balance before being immersed in a petri dish containing PBS for 1 h. Subsequently, the fibers were extracted with tweezers and placed on tissue paper to eliminate any remaining moisture. Finally, the sample was reweighed to assess the extent of liquid absorption [Citation47].
2.7. Drug release profile
For the determination of drug release, the known weight of drug-loaded nanofibers was submerged in phosphate-buffered saline (PBS) with a pH of 5.5 for predetermined time intervals. To establish calibration curves, various standard solutions of levofloxacin were prepared in PBS with a pH of 5.5 (acetic acid was utilized to adjust the pH and enhance the drug’s solubility in the buffer solution). The samples were then placed in an incubator set at 37 °C and rotated at 100 rpm. At specified time points, 1 ml aliquots were extracted, and their absorbance readings were measured using a UV–visible spectrophotometer. Subsequently, the withdrawn solution was replenished into the main flask to maintain sink conditions [Citation31].
2.8. Biocompatibility analysis
The Keratinocytes HaCaT cell line was cultured in Dulbecco’s modified Eagle’s medium (DMEM) supplemented with 10% fetal bovine serum (FBS) and 1% streptomycin–penicillin (Gibco™) in a humidified incubator maintained at 37 °C with 5% CO2. Before seeding the cells onto electrospun mats, the nanofibers were initially collected on 14 mm coverslips (CS) and subjected to UV sterilization for 1 h on each side. Following sterilization, the samples were positioned in 24-well plates and secured with stainless steel O-rings to prevent the mats from curling up off the CS surface. After this, the mats underwent gentle washing with DMEM twice to remove any residual solvent or debris. The cells were then trypsinized, and a seeding density of 1 × 104 cells/well was employed for seeding onto the samples. Additionally, a plain CS was utilized as the control in this experiment.
The viability and proliferation of the cell cultures on the nanofiber mat were evaluated by WST-1 assay as described previously [Citation27]. Briefly, the WST-1 solution was added to the cell culture on the nanofibers in triplicate at three different time intervals of 1, 3, and 7 days. After an incubation period of 4 h, the absorbance of the cell culture medium was recorded at 450 nm by SPECTROstar® nano microplate reader, BMG Labtech, Germany.
The toxicity of the prepared nanofiber was determined by the lactase dehydrogenase (LDH) release assay. Briefly, the prepared nanofibers were introduced to the already cultured HaCaT cell in 24 well plates. The HaCaT cells were cultured for 24 h with nanofibers. After 24 h LDH solution was added to the culture medium and was further incubated for 30 min at 37 °C under darkness. The LDH release was measured by recording the absorbance of the cell culture medium at 490 nm.
Cell adhesion was studied by culturing cells on nanofiber sheets as explained earlier. Using paraformaldehyde 4% cells were fixed on the nanofiber sheets for 20 min, followed by washing with PBS and dehydrating using ethanol washes. SEM micrographs were obtained to understand the morphology and interaction of HaCaT cells over the nanofiber sheets.
To study cell morphology HaCaT cells were cultured on nanofibers placed in 24 well plates for 4 days with a seeding density of 1 × 104 cells/well. The cells were fixed using 4% paraformaldehyde and stained with rhodamine-phalloidin for actin and Hoechst for nuclei. Cells were imaged using a confocal microscope (63X oil immersion objective lens, Nikon Eclipse Ti, Nikon Instruments Inc, NY, USA).
2.9. Antibacterial activity
The antibacterial efficacy of the produced nanofiber webs was evaluated against both Gram-negative and Gram-positive strains, specifically AATCC 6538 Staphylococcus aureus and AATCC 9097 Pseudomonas aeruginosa, utilizing the disk diffusion test method. Initially, Mueller Hinton Agar was meticulously prepared by following the instructions on the bottle, autoclaved, and subsequently cooled to 50 °C. The solution was then poured into sterilized 4-in. plastic Petri dishes within a laminar flow cabinet that had been priorly sterilized with UV light for an hour. Following this, the freshly prepared inoculum of each bacterial strain was evenly spread onto separate solidified agar plates using a cotton swab. The nanofiber samples were carefully placed at the center of the Petri dishes, and thereafter, the dishes were transferred to an incubator set at 37 °C for 24 h to facilitate bacterial growth. After the stipulated incubation period, the zones of inhibition surrounding each nanofiber sample were measured, providing a qualitative assessment of their antibacterial properties.
3. Results and discussions
3.1. Morphology of produced nanofibers
Nanofibers with smooth and defect-free characteristics were successfully produced under the reported electrospinning conditions. For CS-L nanofibers, the average diameter was measured at 165 ± 38 nm, while for SA-L nanofibers, it was 235 ± 43 nm. These dimensions and their standard deviations were determined using SEM (Scanning Electron Microscopy) images at 8,000× magnification and analyzed with Image J software. The data presented are the mean values from 50 samples.
It was noted that the diameter of CS-L nanofibers was consistently smaller than that of alginate nanofibers. This difference is likely due to the intrinsic properties of the polymers and their varying conductivity. Specifically, the CS-L solutions contained 50% acetic acid, which not only enhances conductivity but also exhibits greater volatility compared to water. The rapid evaporation of the solvent and the consequent pulling of the nanofibers toward the collector result in a stretching effect, leading to finer and smaller diameters. This correlation between decreased diameter and fiber stretching is well documented in several published studies [Citation48,Citation49]. Furthermore, as the size of the nanofibers decreases, their surface area increases. Consequently, smaller nanofibers are often preferred over larger ones due to their enhanced properties. The CS-L-based nanofibers, with their smaller diameters and superior morphological characteristics, exemplify this preference, as is also evident in .
3.2. Physicochemical analysis
To obtain crystallographic structural information, the diffraction pattern of the nanofibers and the drug was analyzed. Levofloxacin showed several intense peaks at ∼2θ = 7°, 10°, 13°, 16°, 19°, 23°, and 26° [Citation50]. Both SA-L and CS-L showed characteristic peaks at 2θ 19.1° and 23.3° which can be attributed to the PEO crystals [Citation51]. It was difficult to distinguish the Lev crystal peaks in SA-L nanofiber except for the peak at 2θ = 26°. In CS-L nanofibers peaks associated with Lev were distinguished as can be seen in .
Figure 2. (a) XRD diffractogram, (b) FTIR fingerprints, (c) thermal behavior (TGA), (d) liquid absorption, and (e, f) release behavior of SA-L and CS-L nanofibers.
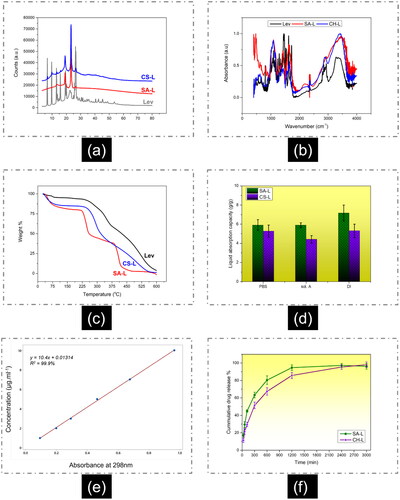
From the FTIR spectra () of SA-L nanofibers a typical band at 1621 cm−1 is related to the vibration of the C=O group and another at 1425 cm−1 is related to the symmetric and asymmetric stretching of the carboxylate group. The broad band around 3100 − 3500 cm−1 corresponds to the O–H group. Finally, the broadband at wavenumber 1320 cm−1, 1090 cm−1, and 950 cm−1 corresponds to C–O stretching, C–C stretching, and C–O–C bonds stretching, while a weak band at 2950 cm−1 corresponds to CH2 stretching of PEO [Citation52,Citation53].
In CS-L nanofibers the band at 3250 cm−1 corresponds to the amine group in chitosan. The bands at 1650 cm−1 and 1580 cm−1 correspond to the vibration of its primary and secondary amine groups. The bands at 1360 cm−1 and 2900 cm−1 are attributed to CH2 stretching and deformation [Citation54].
The pure Lev drug shows strong bands in the range of 1600–1625 cm−1 and 1380–1395 cm−1 assigned to O–C–O asymmetric and symmetric stretching vibrations, respectively. The band at wavenumber 1623 cm−1 corresponds to C = O groups in the ring carbonyl groups. The FTIR spectra also include a broad split band between 3600 and 3100 cm−1 assigned to the adsorbed water O–H and the N–H stretching vibration of the piperazinyl moiety [Citation55]. It was difficult to distinguish between the bands of the loaded drug in the composite nanofibers.
The TGA thermogram () of the SA-L nanofibers shows three distinct regions of weight loss. The first refers to the removal of adsorbed moisture between 25 and 100 °C, the second refers to the degradation of alginate between 225 and 250 °C, while the third one refers to the PEO degradation between 375–400 °C [Citation56]. In the case of CS-L nanofibers, the chitosan degradation occurs between 265 and 325 °C, while further weight loss starting from 360 to 420 °C corresponds to PEO degradation. While comparing the onset of the mass loss of the SA-L and CS-L nanofibers it can be said that CS-L nanofibers have better thermal stability.
3.3. Liquid absorption behavior
Liquid absorption is a crucial parameter in primary wound dressing, particularly for wounds with high exudate levels such as second and third-degree burns. Alginates serve as hydrogel dressings renowned for their exceptional liquid absorption capacity [Citation57]. For instance, the sodium alginate-based product Kaltostat™ can absorb up to 16.30 grams of liquid per gram of dressing [Citation58].
It was observed that the liquid absorption capacity of SA-L nanofibers exceeded that of CS-L nanofibers. Specifically, CS-L nanofibers demonstrated a liquid absorption capacity of approximately 530% in distilled water, which was lower than the 715% absorption capacity exhibited by SA-L nanofibers. Similarly, in solution A, CS-L nanofibers absorbed 441% of liquid, and in saline solution, they absorbed 527%. In contrast, SA-L nanofibers displayed higher liquid absorption rates, reaching 588% and 590% in solution A and saline solutions, respectively. Thus, it can be concluded that SA-L nanofibers exhibit superior liquid absorption capacity compared to CS-L nanofibers, as depicted in .
Previous studies have documented a swelling capacity of approximately 300% for alginate-based nanofibers [Citation59]. In contrast, chitosan-based nanofibers have shown similar results, with around 80% swelling in water [Citation60]. Additionally, another research team reported varying swelling percentages ranging from 80% to 140% by adjusting the weight percentage of chitosan in chitosan–PVA nanofibers for environmental applications [Citation61]. Given the direct correlation between liquid absorption capacity and swelling percentage, it can be inferred that alginates hold a slight advantage over chitosan nanofibers for treating highly exudating wounds. In summary, SA-L nanofibers demonstrate superior liquid absorption capabilities compared to CS-L nanofibers, making them more effective for managing highly exudative wounds. This finding underscores the advantage of alginate-based dressings in optimizing wound healing environments.
3.4. Drug release profile
Polymeric nanofibers have garnered significant attention due to their capacity to release drugs in a controlled and customizable manner. Literature reports a wide range of drug release durations, spanning from seconds to hours, and even extending over months. For instance, researchers have achieved complete drug release from PVA nanofibers in less than 60 s, facilitating the development of fast-dissolving pain relief systems based on nanofibers [Citation62]. Conversely, prolonged drug release exceeding 90 days has been documented from electrospun nanofibers made from polycaprolactone, particularly for anticancer applications [Citation32].
Lev drug was loaded into both SA-L and CS-L nanofibers at very low concentrations (5 µg/ml of electrospinning solutions). Utilizing the calibration curve provided in , drug release from the nanofibers was determined after specific time intervals. Interestingly, it was observed that SA-L nanofibers exhibited a more pronounced burst release compared to CS-L nanofibers. Notably, approximately 95% of the drug was released within 20 h from the SA-L nanofibers, as illustrated in .
The burst release from alginate nanofibers aligns with prior research in this area. Other research groups have also documented high burst release from SA-based nanofibers, corroborating our observations. For instance, Kaassis et al. [Citation63] noted that nearly 90% of drugs were released from SA-based nanofibers within 15 min. They further suggested that the percentage of SA in SA-PEO blended nanofibers did not significantly alter the drug release profiles at pH 6.8 [Citation63]. The gel-forming nature and high absorbency of SA, coupled with the substantial surface area at the nanoscale, likely contribute to the burst release of drugs from SA-PEO nanofibers. Additionally, PEOs high solubility in water may also play a role in facilitating rapid drug release from these systems.
In contrast to alginate nanofibers, the drug release from chitosan nanofibers exhibited a less abrupt profile, as depicted in . Following the initial 6 h, CS-L nanofibers displayed a more gradual release pattern, reaching a plateau around the 50 h mark. Conversely, SA-L nanofibers reached their plateau at approximately 20 h. Notably, in this study, CS-L nanofibers demonstrated a higher release rate compared to some previous literature. This variance could be attributed to the presence of 80 parts of PEO in CS-L nanofibers, as PEO is highly soluble in water. Various studies have reported different drug release rates from CS-based nanofibers. For instance, Cheng et al. [Citation64] documented about 80% of drug releases within 40 h from CS-based nanofibers. The discrepancy in release rates could be linked to the higher proportion of chitosan in blended nanofibers, as they utilized over 90% chitosan in the CS-PEO blend. SA-L nanofibers demonstrate a quicker and more significant drug release than CS-L nanofibers, emphasizing their effectiveness for applications that demand immediate drug delivery responses. On the other hand, CS-L nanofibers can be used for sustained long term drug delivery.
3.5. In vitro biocompatibility analysis: WST-1 cell proliferation; LDH cytotoxicity, cell adhesion and morphology
The WST-1 colorimetric assay was employed to assess HaCaT cell viability following incubation with SA-L and CS-L nanofibers. In metabolically active cells, the tetrazolium compound undergoes metabolism to produce formazan, which is then released into the medium. The resultant change in medium color was quantitatively measured using spectrophotometry to gauge mitochondrial activity, a proxy for cell viability. Measurements were taken at 1, 3, and 7-day intervals (see ). Results from the WST-1 assay indicated that CS-L nanofibers exhibited higher absorbance values compared to SA-L nanofibers at the specified time points. However, there was no significant variance observed between the absorbance values of the tested nanofibers and the negative control (NC), represented by the cell culture plate. According to ISO-10993-5 guidelines, cell viability exceeding 80% is considered non-toxic, while viability between 80% and 60% indicates weak toxicity. Viability ranging from 60% to 40% suggests moderate toxicity and viability below 40% indicates high toxicity [Citation65]. At all predetermined time points, the cell viability of both SA-L and CS-L nanofibers remained above 80%. Specifically, on day 1, the viability was 82% for SA-L and 87% for CS-L. On day 3, it was 83% for SA-L and 90% for CS-L. Finally, on day 7, the viability reached 87% for SA-L and 95% for CS-L nanofibers.
Figure 3. Biocompatibility analysis of keratinocyte HaCaT cell line (a) WST-1 mitochondrial activity, (b) LDH cytotoxicity analysis, and (c) cell viability in the presence of SA-L and CS-L nanofibers.
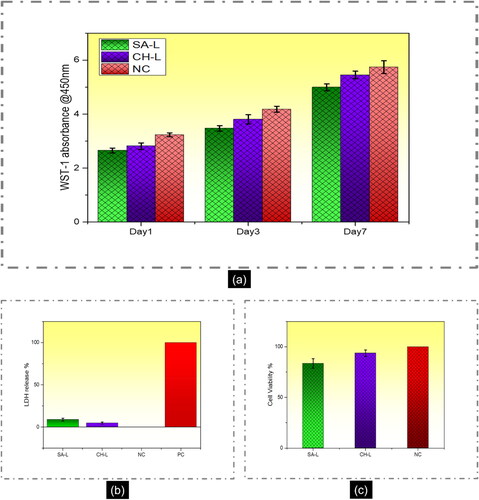
The toxicity of the prepared nanofibers was assessed using the LDH release assay, which measures the release of the LDH enzyme from damaged plasma membranes within the cellular culture. Increased LDH release correlates with greater cellular damage and cytotoxicity. Results depicted in illustrate that after 24 h of incubation with SA-L and CS-L nanofibers, the amount of LDH released from HaCaT cells was ∼9% for SA-L and ∼6% for CS-L nanofibers. The results reveal that the amount of LDH released was less than 10% in both cases and it could be due to the apoptosis of the HaCaT cells during incubation. illustrates the viable cells during the LDH toxicity test. The LDH assay reveals that the prepared nanofiber mats are not cytotoxic.
Cell adhesion and morphology play pivotal roles in assessing the suitability of a scaffold for tissue engineering and wound care applications. depicts scanning electron microscopy (SEM) micrographs of HaCaT cells on SA-L and CS-L nanofibers on day 3 and day 7 of incubation. Observation of the SEM micrographs reveals that HaCaT cells on the SA-L nanofibers appeared more rounded, agglomerated, and formed clusters at both day 3 and day 7 of incubation. These findings suggest that on alginate-based nanofibers, cell– interactions may be more pronounced than cell–material interactions, potentially leading to diminished cell adhesion to the material surface and the formation of cell clusters [Citation2,Citation66].
Figure 4. SEM micrographs: (a) HaCaT cell adhesion on SA-L and CS-L nanofibers after 3 and 7 days of incubation (b) confocal images of keratinocytes actin stained (red) and nuclei stained (blue).
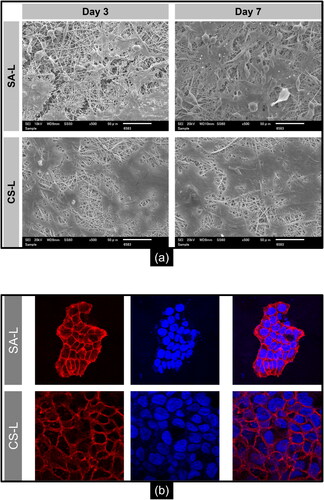
Though on CS-L nanofibers the microcolonies of the keratinocytes are rather smooth and show strong cell–material interactions. A healthy human skin has a negative surface charge developed by the keratinocytes [Citation67]. Chitosan with a positive charge due to its free amino groups, allows the effective interaction with the negatively charged cellular membranes, thus promoting better cell attachment which is significant for cell proliferation and migration. On the contrary, alginate possesses anionic charge which results in the clustering of the already negatively charged keratinocytes cellular membrane.
The confocal images of the HaCaT cells on SA-L and CS-L are shown in . In the case of SA-L nanofibers, the cells can be seen in an agglomerated form while in the case of CS-L nanofibers, the keratinocytes formed intact microcolonies of the surface of the randomly collected nanofibers.
The SEM and confocal microscopy data provide insightful comparisons between the cell adhesion and morphology on SA-L and CS-L nanofibers. HaCaT cells on SA-L nanofibers display a rounded, clustered morphology indicative of stronger cell– over cell–material interactions, possibly due to the alginate’s anionic charge which repels the similarly charged cell membranes. Conversely, the positively charged CS-L nanofibers facilitate robust cell–material interactions and enhance keratinocyte attachment and proliferation, reflecting their potential suitability for applications in tissue engineering and wound healing.
3.6. Antibacterial testing
Levofloxacin operates by blocking the function of DNA gyrase and topoisomerase IV in bacteria, essential enzymes for DNA replication, leading to broad-spectrum efficacy against many aerobic Gram-negative bacteria. However, specific strains such as Providencia rettgeri and Pseudomonas aeruginosa may exhibit moderate sensitivity, while Serratia marcescens can show resistance [Citation68]. shows the antibacterial efficacy of the SA-L and CS-L nanofibers in agar disc diffusion tests. The results from show that the levofloxacin-loaded nanofibers were efficient in disrupting bacteria growth as can be seen by halos created around the nanofiber samples. The inhibition zones against P. aeruginosa were bigger than the zones in the S. aureus case which correlates to the higher MIC values required for gram-positive bacteria in the case of levofloxacin [Citation68].
4. Conclusion
This research project presents a comparative in vitro analysis of sodium alginate (SA-L) and chitosan (CS-L) electrospun nanofibers, both incorporated with levofloxacin (Lev) for moist wound management. The study thoroughly investigates the physicochemical, thermal, antibacterial, and cytocompatibility properties of these nanofibers. Results show that both SA-L and CS-L nanofibers were produced successfully with a homogeneous, defect-free structure, and the incorporation of Lev was confirmed through XRD and FTIR analyses. Sodium alginate nanofibers exhibited superior liquid absorption capabilities compared to chitosan nanofibers, likely due to alginate’s inherent gel-forming properties in aqueous environments, enhancing their suitability for moist wound management. Furthermore, SA-L nanofibers showed faster Lev release rates, which could be advantageous for initial high-dose drug delivery to wound sites. Both nanofibers demonstrated effective antibacterial activity against Staphylococcus aureus and Pseudomonas aeruginosa, with CS-L nanofibers showing larger inhibition zones, attributable to chitosan’s inherent antibacterial properties. Cytocompatibility tests using the HaCat cell line indicated that both nanofibers are biocompatible and non-toxic, with CS-L nanofibers promoting superior cell proliferation. This difference is potentially due to the positively charged amine groups in chitosan, which favor the proliferation and migration of keratinocytes. This research highlights the potential of these nanofibers in wound management applications, offering insights into their specific advantages and suitability for different wound care scenarios.
Author contributions
Sharjeel Abid: Conceptualization, Methodology, Writing – original draft, Investigation, Visualization, Data curation. Ling Wang: Methodology, Investigation, writing – review and editing, Visualization, Data curation. Md. Kaiser Haider: Formal analysis, data curation. Gopiraman Mayakrishnan: Resource, Validation. Rajamani Lakshminarayanan: Resource. Kyu Oh Kim: Formal analysis, Resource. Azeem Ullah: Writing – original draft, Writing – review and editing, Supervision. Ick Soo Kim: Validation, Supervision.
Disclosure statement
The authors declare that there are no conflicts of interest.
Data availability
No data was used for the research described in the article.
Additional information
Funding
Notes on contributors
Sharjeel Abid
Dr., Ph.D. Sharjeel Abid is a distinguished researcher in the field of materials science, specifically focusing on biopolymers, drug delivery systems, and electrospinning technologies. He is currently serving as an assistant professor at National Textile University, Faisalabad, Pakistan. His research interests are electrospinning, biopolymers, drug delivery, and textile processing. Email: [email protected]
Ling Wang
Ling Wang is a Doctoral Student at Shinshu University, in Japan. Her research interest includes the fabrication of electrospun nonwoven membranes for tissue engineering and wound care. Email: [email protected]
Md. Kaiser Haider
Md. Kaiser Haider is a Doctoral Student at Shinshu University, in Japan. His research interests include lignin-mediated nanocomposite for biomedical applications. Email: [email protected]
Gopiraman Mayakrishnan
Dr., Ph.D. Gopiraman Mayakrishnan is serving as an Associate Professor in the Institute of Fiber Engineering, Shinshu University, Japan. His research expertise includes the fabrication of electrospun membranes for biomedical, energy, and catalysis applications. Email: [email protected]
Rajamani Lakshminarayanan
Prof., Ph.D. Rajamani Lakshinarayan is an Associate Professor and is currently heading the anti-infective research Singapore Eye Research Institute. His research interests include the fabrication of bioinspired materials and biomineralization. Email: [email protected]
Kyu Oh Kim
Dr, Ph.D. Kyu Oh Kim is an Assistant Professor at the Department of Fiber System Engineering, at Dankook University, Korea. Her research interests include biosensors, nanofibers, and drug delivery systems. Email: [email protected]
Azeem Ullah
Dr., D. Eng. Azeem Ullah is an Assistant Professor at the Institute of Fiber Engineering, Shinshu University, Japan. His research expertise includes electrospinning of biopolymers, tissue engineering, drug delivery, biomineralization, and bioactive food packaging. Email: [email protected]
Ick Soo Kim
Prof., D. Eng. Ick Soo Kim is a Distinguished Professor at the Institute of Fiber Engineering, Shinshu University, Japan. His area of expertise includes functional nanofibers for biomedical, sensor, and energy applications. Email: [email protected]
References
- Afshari M, Kotek R, Chen P. High performance fibers. In: High performance polymers and engineering plastics. New York: John Wiley & Sons, Inc; 2011. p. 269–340. doi: 10.1002/J9781118171950.ch9.
- Umar M, Ullah A, Nawaz H, et al. Wet-spun bi-component alginate based hydrogel fibers: development and in-vitro evaluation as a potential moist wound care dressing. Int J Biol Macromol. 2021;168:601–610. doi: 10.1016/j.ijbiomac.2020.12.088.
- Grand View Research. 2023. Medical textiles market size, share and trend analysis.
- Dhivya S, Padma VV, Santhini E. Wound dressings: a review. Biomedicine (Taipei). 2015;5(4):22. doi: 10.7603/s40681-015-0022-9.
- Masood R, Hussain T, Miraftab M, et al. Development of tri-component antibacterial hybrid fibres for potential use in wound care. J Wound Care. 2018;27(6):394–402. doi: 10.12968/jowc.2018.27.6.394.
- Dabiri G, Damstetter E, Phillips T. Choosing a wound dressing based on common wound characteristics. Adv Wound Care (New Rochelle). 2016;5(1):32–41. doi: 10.1089/wound.2014.0586.
- Boateng JS, Matthews KH, Stevens HNE, et al. Wound healing dressings and drug delivery systems: a review. J Pharm Sci. 2008;97(8):2892–2923. doi: 10.1002/jps.21210.
- Hussain T, Masood R, Umar M, et al. Development and characterization of alginate–chitosan–hyaluronic acid (ACH) composite fibers for medical applications. Fibers Polym. 2016;17(11):1749–1756. doi: 10.1007/s12221-016-6487-7.
- Masood R, Hussain T, Miraftab M, et al. Novel alginate, chitosan, and psyllium composite fiber for wound-care applications. J Ind Text. 2017;47(1):20–37. doi: 10.1177/1528083716632805.
- Ambekar RS, Kandasubramanian B. Advancements in nanofibers for wound dressing: a review. Eur Polym J. 2019;117(March):304–336. doi: 10.1016/j.eurpolymj.2019.05.020.
- Liu L, Zhang J, Zou X, et al. A high-stable and sensitive colorimetric nanofiber sensor based on PCL incorporating anthocyanins for shrimp freshness. Food Chem. 2022;377(July 2021):131909. doi: 10.1016/j.foodchem.2021.131909.
- Yang J, Xu L. Electrospun nanofiber membranes with various structures for wound dressing. Materials (Basel). 2023;16(17). doi: 10.3390/ma16176021.
- Rogalski JJ, Bastiaansen CWM, Peijs T. Rotary jet spinning review: a potential high yield future for polymer nanofibers. Nanocomposites. 2017;3(4):97–121. doi: 10.1080/20550324.2017.1393919.
- Ullah A, Sun L, Wang F, et al. Eco-friendly bioactive β-caryophyllene/halloysite nanotubes loaded nanofibrous sheets for active food packaging. Food Packag Shelf Life. 2023;35(March):101028. doi: 10.1016/j.fpsl.2023.101028.
- Ullah A, Yang H, Takemae K, et al. Sustainable bioactive food packaging based on electrospun zein-polycaprolactone nanofibers integrated with aster Yomena extract loaded halloysite nanotubes. Int J Biol Macromol. 2024;267(Pt 2):131375. doi: 10.1016/j.ijbiomac.2024.131375.
- Hakro RA, Mehdi M, Qureshi RF, et al. Efficient removal of reactive blue-19 dye by co-electrospun nanofibers. Mater Res Expr. 2021;8(5):055502. doi: 10.1088/2053-1591/abfc7d.
- Hashmi M, Ullah S, Ullah A, et al. An optimistic approach “from hydrophobic to super hydrophilic nanofibers” for enhanced absorption properties. Polym Test. 2020;90(March):106683. doi: 10.1016/j.polymertesting.2020.106683.
- Jamali AA, Mahar FK, Hussain N, et al. Fabrication of chitosan-β-cyclodextrin-Fe nanofibers for the adsorption of As-III from aqueous solution using a lab-scale filtration system. J Appl Polym Sci. 2023;140(36):10. doi: 10.1002/app.54367.
- Kausar A, Ahmad I, Aldaghri O, et al. Cutting-edge green nanoclay nanocomposites: fundamentals and technological opportunities for packaging, dye removal, and biomedical sectors. Nanocomposites. 2024;10(1):172–196. doi: 10.1080/20550324.2024.2336356.
- Wang F, Zhang Y, Shi J, et al. Bioinspired and biodegradable functionalized graphene oxide/deacetylated cellulose acetate composite Janus membranes for water evaporation-induced electricity generation. ACS Sustain Chem Eng. 2023;11(26):9792–9803. doi: 10.1021/acssuschemeng.3c01952.
- Sun L, Cai Y, Kim D, et al. Enhanced properties of solid polymer electrolytes by a bilayer nonwoven pet/nanofiber PVDF substrate for use in all-solid-state lithium metal batteries. J Power Sources. 2023a;564(April):232851. doi: 10.1016/j.jpowsour.2023.232851.
- Sun L, Miyagi D, Cai Y, et al. Rational construction of hierarchical nanocomposites by growing dense polyaniline nanoarrays on carbon black-functionalized carbon nanofiber backbone for freestanding supercapacitor electrodes. J Storage Mater. 2023b;61(vember 2022):106738. doi: 10.1016/j.est.2023.106738.
- Audtarat S, Hongsachart P, Dasri T, et al. Green synthesis of silver nanoparticles loaded into bacterial cellulose for antimicrobial application. Nanocomposites. 2022;8(1):34–46. doi: 10.1080/20550324.2022.2055375.
- Haider MK, Kharaghani D, Sun L, et al. Synthesized bioactive lignin nanoparticles/polycaprolactone nanofibers: a novel nanobiocomposite for bone tissue engineering. Biomater Adv. 2023;144(June 2022):213203. doi: 10.1016/j.bioadv.2022.213203.
- Parın FN, El-Ghazali S, Yeşilyurt A, et al. PVA/inulin-based sustainable films reinforced with pickering emulsion of niaouli essential oil for potential wound healing applications. Polymers (Basel). 2023;15(4):1002. doi: 10.3390/polym15041002.
- Ullah A, Haider K, Wang F, et al. Clay-corn-caprolactone” a novel bioactive clay polymer nanofibrous scaffold for bone tissue engineering. Appl Clay Sci. 2022a;220:106455. doi: 10.1016/j.clay.2022.106455.
- Ullah A, Sarwar MN, Wang F, et al. In vitro biocompatibility, antibacterial activity, and release behavior of halloysite nanotubes loaded with diclofenac sodium salt incorporated in electrospun soy protein isolate/hydroxyethyl cellulose nanofibers. Curr Res Biotechnol. 2022b;4(May):445–458. doi: 10.1016/j.crbiot.2022.09.008.
- Liu Y, Zhou S, Gao Y, et al. Electrospun nanofibers as a wound dressing for treating diabetic foot ulcer. Asian J Pharm Sci. 2019;14(2):130–143. doi: 10.1016/J.AJPS.2018.04.004.
- Abid S, Hussain T, Nazir A, et al. Development of nanofibers based neuropathic patch loaded with Lidocaine to deal with nerve pain in burn patients. IOP Conf Ser: Mater Sci Eng. 2018;414(1):012019. doi: 10.1088/1757-899X/414/1/012019.
- Abid S, Hussain T, Nazir A, et al. Acetaminophen loaded nanofibers as a potential contact layer for pain management in burn wounds. Mater Res Expr. 2018;5(8):085017. doi: 10.1088/2053-1591/aad2eb.
- Abid S, Hussain T, Nazir A, et al. Enhanced antibacterial activity of PEO-chitosan nanofibers with potential application in burn infection management. Int J Biol Macromol. 2019;135:1222–1236. doi: 10.1016/j.ijbiomac.2019.06.022.
- Chen S, Boda SK, Batra SK, et al. Emerging roles of electrospun nanofibers in cancer research. Adv Healthc Mater. 2018;7(6):e1701024. doi: 10.1002/adhm.201701024.
- Haider MK, Ullah A, Sarwar MN, et al. Lignin-mediated in-situ synthesis of CuO nanoparticles on cellulose nanofibers: a potential wound dressing material. Int J Biol Macromol. 2021;173:315–326. doi: 10.1016/j.ijbiomac.2021.01.050.
- Rasouli R, Barhoum A, Bechelany M, et al. Nanofibers for biomedical and healthcare applications. Macromol Biosci. 2019;19(2):e1800256. doi: 10.1002/mabi.201800256.
- Sridhar R, Venugopal JR, Sundarrajan S, et al. Electrospun nanofibers for pharmaceutical and medical applications. J Drug Deliv Sci Technol. 2011;21(6):451–468. doi: 10.1016/S1773-2247(11)50075-9.
- Sen S, Kumbhar AP, Patil JR, et al. Nanofibers: an effective biomedical tool for burn management. J Drug Deliv Sci Technol. 2023;87(July):104882. doi: 10.1016/j.jddst.2023.104882.
- Sari MHM, Cobre ADF, Pontarolo R, et al. Status and future scope of soft nanoparticles-based hydrogel in wound healing. Pharmaceutics. 2023;15(3):874. doi: 10.3390/pharmaceutics15030874.
- Nozari M, Gholizadeh M, Zahiri Oghani F, et al. Studies on novel chitosan/alginate and chitosan/bentonite flexible films incorporated with ZnO nano particles for accelerating dermal burn healing: in vivo and in vitro evaluation. Int J Biol Macromol. 2021;184(June):235–249. doi: 10.1016/j.ijbiomac.2021.06.066.
- Aboomeirah AA, Sarhan WA, Khalil EA, et al. Wet electrospun nanofibers-fortified gelatin/alginate-based nanocomposite as a single-dose biomimicking skin substitute. ACS Appl Bio Mater. 2022;5(8):3678–3694. doi: 10.1021/acsabm.2c00147.
- Hu Y, Zhang Z, Li Y, et al. Dual-crosslinked amorphous polysaccharide hydrogels based on chitosan/alginate for wound healing applications. Macromol Rapid Commun. 2018;39(20):e1800069. doi: 10.1002/marc.201800069.
- Sadeghi-Aghbash M, Rahimnejad M, Adeli H, et al. Fabrication and development of PVA/alginate nanofibrous mats containing Arnebia Euchroma extract as a burn wound dressing. React Funct Polym. 2022;181(June):105440. doi: 10.1016/j.reactfunctpolym.2022.105440.
- Stoica AE, Chircov C, Grumezescu AM. Hydrogel dressings for the treatment of burn wounds: an up-to-date overview. Materials (Basel). 2020;13(12):2853. doi: 10.3390/ma13122853.
- Dragostin OM, Samal SK, Dash M, et al. New antimicrobial chitosan derivatives for wound dressing applications. Carbohydr Polym. 2016;141:28–40. doi: 10.1016/j.carbpol.2015.12.078.
- Sami DG, Abdellatif A, Azzazy HME. Turmeric/oregano formulations for treatment of diabetic ulcer wounds. Drug Dev Indus Pharm. 2020;46(10):1613–1621. doi: 10.1080/03639045.2020.1811305.
- Safi S, Morshed M, Hosseini Ravandi SA, et al. Study of electrospinning of sodium alginate, blended solutions of sodium alginate/poly(vinyl alcohol) and sodium alginate/poly(ethylene oxide). J Appl Polym Sci. 2007;104(5):3245–3255. doi: 10.1002/app.25696.
- Duval M, Sarazin D. Properties of PEO in dilute solution under stirring. Macromolecules. 2003;36(4):1318–1323. doi: 10.1021/ma0208638.
- Rafiq M, Hussain T, Abid S, et al. Development of sodium alginate/PVA antibacterial nanofibers by the incorporation of essential oils. Mater Res Expr. 2018;5(3):035007. doi: 10.1088/2053-1591/aab0b4.
- Haider A, Haider S, Kang I-K. A comprehensive review summarizing the effect of electrospinning parameters and potential applications of nanofibers in biomedical and biotechnology. Arab J Chem. 2015;11(8):1165–1188. doi: 10.1016/j.arabjc.2015.11.015.
- Subbiah T, Bhat GS, Tock RW, et al. Electrospinning of nanofibers. J Appl Polym Sci. 2005;96(2):557–569. doi: 10.1002/app.21481.
- Islan GA, Tornello PC, Abraham GA, et al. Smart lipid nanoparticles containing levofloxacin and DNase for lung delivery: design and characterization. Colloids Surf B Biointerfaces. 2016;143:168–176. doi: 10.1016/j.colsurfb.2016.03.040.
- Singh YP, Dasgupta S, Nayar S, et al. Optimization of electrospinning process and parameters for producing defect-free chitosan/polyethylene oxide nanofibers for bone tissue engineering. J Biomater Sci Polym Ed. 2020;31(6):781–803. doi: 10.1080/09205063.2020.1718824.
- Bonino CA, Krebs MD, Saquing CD, et al. Electrospinning alginate-based nanofibers: from blends to crosslinked low molecular weight alginate-only systems. Carbohydr Polym. 2011;85(1):111–119. doi: 10.1016/j.carbpol.2011.02.002.
- Kane SN, Mishra A, Dutta AK. Preface: international conference on recent trends in physics (ICRTP 2016). J Phys Conf Ser. 2016;755(1). doi: 10.1088/1742-6596/755/1/011001.
- Nista SVG, Bettini J, Mei LHI. Coaxial nanofibers of chitosan–alginate–PEO polycomplex obtained by electrospinning. Carbohydr Polym. 2015;127:222–228. doi: 10.1016/j.carbpol.2015.03.063.
- Qassim AW. Determination of levofloxacin in pharmaceutical formulationtavanic by visible spectrophotometry of its chelating complex with aluminum ion (III). Researchgate.Net, June. 2015. https://www.researchgate.net/publication/285494150.
- Çaykara T, Demirci S, Eroğlu MS, et al. Poly(ethylene oxide) and its blends with sodium alginate. Polymer. 2005;46(24):10750–10757. doi: 10.1016/j.polymer.2005.09.041.
- Qin Y. Absorption characteristics of alginate wound dressings. J Appl Polym Sci. 2004;91(3):1641–1645. doi: 10.1002/app.13291.
- Yong K, Mooney DJ. Alginate: properties and biomedical applications. Prog Polym Sci. 2012;37(1):106–126. doi: 10.1016/j.progpolymsci.2011.06.003.
- Aadil KR, Nathani A, Sharma CS, et al. Fabrication of biocompatible alginate-poly(vinyl alcohol) nanofibers scaffolds for tissue engineering applications. Mater Technol. 2018;33(8):507–512. doi: 10.1080/10667857.2018.1473234.
- Qasim SB, Najeeb S, Delaine-Smith RM, et al. Potential of electrospun chitosan fibers as a surface layer in functionally graded GTR membrane for periodontal regeneration. Dent Mater. 2017;33(1):71–83. doi: 10.1016/J.DENTAL.2016.10.003.
- Pervez M, Stylios G. Investigating the synthesis and characterization of a novel “green” H2O2-assisted, water-soluble chitosan/polyvinyl alcohol nanofiber for environmental end uses. Nanomaterials. 2018;8(6):395. doi: 10.3390/nano8060395.
- Li X, Kanjwal MA, Lin L, et al. Electrospun polyvinyl-alcohol nanofibers as oral fast-dissolving delivery system of caffeine and riboflavin. Colloids Surf B Biointerfaces. 2013;103:182–188. doi: 10.1016/j.colsurfb.2012.10.016.
- Kaassis AYA, Young N, Sano N, et al. Pulsatile drug release from electrospun poly(ethylene oxide)–sodium alginate blend nanofibres. J Mater Chem B. 2014;2(10):1400–1407. doi: 10.1039/C3TB21605E.
- Cheng F, Gao J, Wang L, et al. Composite chitosan/poly(ethylene oxide) electrospun nanofibrous mats as novel wound dressing matrixes for the controlled release of drugs. J Appl Polym Sci. 2015;132(24):1–8. doi: 10.1002/app.42060.
- Ullah A, Saito Y, Ullah S, et al. Bioactive Sambong oil-loaded electrospun cellulose acetate nanofibers: preparation, characterization, and in-vitro biocompatibility. Int J Biol Macromol. 2021;166:1009–1021. doi: 10.1016/j.ijbiomac.2020.10.257.
- Sarker B, Singh R, Silva R, et al. Evaluation of fibroblasts adhesion and proliferation on alginate–gelatin crosslinked hydrogel. PLoS One. 2014;9(9):e107952. doi: 10.1371/journal.pone.0107952.
- Denda M, Nakanishi S. Do epidermal keratinocytes have sensory and information processing systems? Exp Dermatol. 2022;31(4):459–474. doi: 10.1111/exd.14494.
- Davis R, Bryson HM. Levofloxacin a review of its antibacterial activity, pharmacokinetics and therapeutic efficacy. Drug Eval. 2012;47:677–700. doi: 10.2165/00003495-199447040-00008.