ABSTRACT
Background: For over a decade, resistance to newly synthesized antibiotics has been observed worldwide. The challenge of antibiotic resistance has led to several pharmaceutical companies to abandon the synthesis of new drugs in fear of bacteria developing resistance in a short period hence limiting initial investment return. To this effect, alternative approaches such as the use of bacteriophages to treat bacterial infections are being explored. This review explores the recent advances in phage-mediated antibacterial applications and their limitations.
Methods: We conducted a comprehensive literature search of PubMed, Lib Hub and Google Scholar databases from January 2019 to November 2019. The search key words used were the application of bacteriophages to inhibit bacterial growth and human phage therapy to extract full-text research articles and proceedings from International Conferences published only in English.
Results: The search generated 709 articles of which 95 full-text research articles fulfilled the inclusion guidelines. Transmission Electron Microscopy morphological characterization conducted in 23 studies registered Myoviruses, Siphoviruses, Podoviruses, and Cytoviruses phage families while molecular characterization revealed that some phages were not safe to use as they harbored undesirable genes. All in vivo phage therapy studies in humans and model animals against multidrug-resistant (MDR) bacterial infection provided 100% protection. Ex vivo and in vitro phage therapy experiments exhibited overwhelming results as they registered high efficacies of up to 100% against MDR clinical isolates. Phage-mediated bio-preservation of foods and beverages and bio-sanitization of surfaces were highly successful with bacterial growth suppression of up to 100%. Phage endolysins revealed efficacies statistically comparable to those of phages and restored normal ethanol production by completely eradicating lactic acid bacteria in ethanol fermenters. Furthermore, the average multiplicity of infection was highest in ex vivo phage therapy (557,291.8) followed by in vivo (155,612.4) and in vitro (434.5).
1. Background
Currently, the world populace is deemed to be at a great risk as a result of the ever-escalating prevalence of antibiotic resistance bringing about an epoch where many familiar bacterial infections are becoming increasingly hard to treat [Citation1]. Similar to many other developing countries, Sub-Saharan Africa is experiencing an elevated burden of bacterial infectious diseases which calls for the overuse of antibiotics and consequently emergence of resistant microorganisms [Citation1,Citation2]. The development of antibiotic resistance is also contributed by self-medication with uncontrolled over-the-counter access to drugs without any guidance from qualified medical practitioners. In addition, there is excessive application of antibiotics in poultry, aquaculture, and livestock production. The unrestricted access and use of antibiotics for animal disease treatment and prophylaxis as well as growth promotion have been implicated as one of the major drivers for antibiotic resistance that may spillover to humans [Citation3–5]. Infectious food and water-borne illnesses are acquired through the consumption of contaminated food and water; and are the major cause of mortality and morbidity worldwide owing to their extensive and spontaneous transmission [Citation6,Citation7]. It was estimated that water, sanitation, and hygiene (WSH) associated infectious diseases are accountable for 4.0% of the worldwide deaths and 5.7% of the universal disease burden [Citation7,Citation8]. Furthermore, WHO reported that 600 million or 1 in 10 people fall ill worldwide as a result of foodborne infections and more than 91 million people affected are in Africa [Citation6].
The rate at which drug resistance emerges has resulted in big pharmaceutical companies backing away from developing new antibiotics since the latter are rendered non-effective within a short period, making the venture not cost-effective [Citation9]. Therefore, affordable alternative approaches such as the use of probiotics, phytomedicines, and bacteriophages to manage bacterial infections and control the emergence of antibiotic resistance are highly commendable.
Bacteriophages (phages) are natural enemies of bacteria which are the most abundant replicating entities on earth. Phages are viruses that specifically attack and multiply in bacterial cells and have no effect on other cell types. They are self-replicating and self-limiting as long as the specific bacterial host cells exist. Similar to other viruses, their genomes may either be double-stranded or single-stranded DNA or RNA [Citation10]. Phages have either a lytic or lysogenic type of replication cycle. The lytic cycle, also referred to as the virulent cycle, results in the production of progeny viruses that are released through cell lysis. The lysogenic or temperate cycle results in the incorporation of the phage genome into the host chromosome without the production of new virus particles. Depending on some circumstances, some phages can exhibit both replication cycles [Citation10]. Lytic phages are applied as bacterial growth inhibitors, which can be categorized as phage therapy or phage-mediated decontaminants. For therapy, phages are mainly used like antibiotics, whereas for decontamination, they are applied as disinfectants. Literally, phage therapy is the application of phages as therapeutic agents more especially in a clinical context to treat bacterial infections while phage-mediated biocontrol can be defined as the use of phages to suppress bacterial growth on non-living surfaces. Safety and efficacy of phage therapy or phage-mediated biocontrol relies on isolation and use of only professional lytic phages, which are obligately lytic or virulent but they are neither temperate nor directly linked to temperate phages [Citation11]. Phage therapy is a proven eco-friendly alternative approach to prevent and control pathogenic bacterial infections [Citation12,Citation13].
Phages were used to treat bacterial infections in Europe during the pre-antibiotic era. However, with the discovery of antibiotics and the substandard medical trials conducted in the western world without putting into consideration that phages were specific, phage therapy was shortly after deemed impotent in the treatment of bacterial infections. Nevertheless, phage therapy continued to be used for the treatment of bacterial infections in the Soviet Union since 1940 [Citation14]. The advantages of phage applications, such as disruption of bacterial biofilms and nondependency on the drug resistance status of the organisms, have rekindled their use as antibacterial agents [Citation15,Citation16]. Furthermore, renewed attention to phage therapy has been registered due to an overall decline in the total reserves of effective antibiotics. Hence, phage therapy clinical trials and experiments in poultry, aquaculture, crop husbandry, model animals, in vitro model systems, and humans have been widely carried out [Citation17,Citation18]. Currently, the notable human phage therapy under application is the compassionate use of phages as individualized therapeutic options to manage MDR bacterial infections unresponsive to all classes of conventional antibiotics [Citation19]. Furthermore, phage preparations have been used and experimented with as diagnostic tools for bacterial infections to supplement the available methods [Citation12].
For use as decontaminants, several studies have been conducted to evaluate the efficacy of phages as bio-control agents against food and beverage borne pathogens [Citation20]. Phages have been experimented with in bio-sanitization of equipment surfaces to eradicate biofilms in food industries [Citation21]; and bio-preservation of perishable processed foods to increase shelf-life. Some phage-specific enzymes; such as lysins which degrade the cell wall of gram-positive bacteria, have been applied to processed foods to enhance their safety for human consumption [Citation18,Citation22–24]. The use of bacteriophages in food products in the US, Europe, and Australia has been reported [Citation25]. Indeed, some phage preparations have been approved in the USA and are commercially available; such as LISTEX P100; LMP-102TM, ListshieldTM, ECP-100TM (EcoshieldTM), SALMONELEXTM, AgriPhageTM, and Biophage-PA [Citation26].
This review expounds on the current level, limitations, and prospects of phage applications such as enhancing food safety and fermentation of biofuels; phage therapy clinical trials and experiments in humans and model animals; animal and plant disease control and environmental bioremediation.
2. Methods
2.1. Literature search strategy
A comprehensive literature search of PubMed, Lib Hub, and Google Scholar databases was conducted from January 2018 to November 2018. The search key words used were “application of bacteriophages to inhibit bacterial growth” and “human phage therapy,” .
3. Study selection criteria
The search targeted articles published in English without restriction on year of publication in an attempt to capture all available literature about the application of phages as antibacterial agents worldwide, . In addition, only full-text research articles and proceedings from the International Conference on Prevention & Infection Control were selected, , S1. Review articles were excluded from this search. To avoid bias, all the seven coauthors were involved in the selection process. Articles were assigned to the different coauthors blindly, review reports on the merits and demerits of the studies as per inclusion criteria were submitted to the lead researcher (JLN) and the entire selection process was conducted based on the review reports by all the seven coauthors. In case of any disagreement, powers were entrusted to the most experienced researchers in bacteriophages (JLN, DKB, and FE) to make the final decision.
Table 1. In vivo human phage therapy trials
Table 2. Phage-mediated biocontrol of bacterial growth in ethanol fermentation, foods, and beverages
Table 3. In vitro phage therapy against clinical isolates assays
Table 4. In vivo and ex vivo phage therapy experiments in animal models/tissues, fish, plants, poultry, piggery, and bees
Table 5. Phage application in biosanitization
4. Data extraction
A database was created in which the field of phage application, type of phage or phage part used, source of phages, level of phage application, type of bacteria and strain or serovar challenged, level of phage efficacy, physiochemical properties of phages, the multiplicity of infection (MOI) of phages and methods used in the characterization of phages were included. Studies where MOIs were not reported but the number of plaque-forming units/mL (PFU/mL) and the number of colony-forming units/mL (CFU/mL) given, MOIs were computed by dividing the PFU/mL by CFU/mL units (O’Flynn et al., 2004). To compare the MOI of different investigations, all studies were grouped into three categories namely; in vivo phage therapy, ex vivo phage therapy, and in vitro phage therapy.
5. Data analysis
Data analysis was performed using Tukey’s multiple comparisons test in STATA version 2018.1 to establish whether; (a) the number of studies that reported in vivo human phage therapy efficacy of 100% was more pronounced than the number of studies that recorded efficacy lower than 100%, (b) phages are more efficient inhibitors of bacterial growth in ethanol fermenters than phage endolysins, (c) there is a considerable difference in in vitro phage therapy outcomes against different species of clinical bacterial isolates, (d) the outcomes of phage-mediated biocontrol in different fields are momentously dissimilar, (e) MOIs used for ex vivo phage therapy/phage-mediated biocontrol experiments, in vivo phage therapy and in vitro phage therapy are soundly similar. A P value of ≤ 0.05 indicated a significant statistical difference. For comparison of phage therapy and phage-mediated biocontrol efficacy across the different fields, only fields that had three or more studies reporting phage therapy efficacy in percentages were considered for Tukey’s multiple comparisons test to prevent skewing of data.
6. Results and discussion
6.1. Literature search
A total of 709 articles were generated through an electronic database literature search conducted between January and November 2018. The databases were PubMed, Lib Hub, and Google Scholar, which yielded 51, 416, and 242 articles, respectively. Following the removal of duplications, 204 articles were screened on the basis of their titles and abstracts. Of the 204 articles; 90 did not meet the specified inclusion criteria; and five full-text articles were not accessible. Finally, 109 full-text articles were reviewed, of which 95 full-text research articles fulfilled the inclusion guidelines for this review, . Studies included in this review were grouped into in vivo human phage therapy, in vivo phage therapy in model organisms, phages as biocontrol agents in biofuels fermentation, phages as biocontrol agents in foods and beverages, in vitro phage therapy experiments using clinical isolates, in vivo phage therapy in crop protection, application of phages as biocontrol agents in water purification, in vivo phage therapy in aquaculture, in vivo phage therapy in apiculture, in vivo phage therapy in a piggery in vivo and in vitro phage therapy in poultry, application of phages as bio-sanitizers, and in vitro use of phages as biocontrol agents in creams, , .
Phage characterization; a prerequisite for phage-mediated biocontrol of bacterial growth and in vivo phage therapy
Phage-mediated biocontrol and phage therapy rely on the ability of lytic phages to infect bacterial host cells, hijacking the host metabolism and utilizing it to produce their progeny. As a result, the lytic phages lyse bacteria cells to release multiple phage virions which spread to infect other host cells [Citation10]. Contrary to that, after infecting the bacterial host cells, lysogeny phages incorporate their genetic material into the host genome resulting in their permanent existence as prophages within host cells and all their offspring. Phages neither replicate into virions nor lyse bacteria throughout their lysogeny life time, hence called temperate phages [Citation10]. Furthermore, the integration of the phage nucleic acids into its host bacterium protects the temperate phage genome and has the ability to modify the phenotype of the host bacterium cell [Citation27]. Unfortunately, temperate phages might harbor toxin encoding genes, virulent genes, and genetic determinants of antibiotic resistance acquired from other bacterial hosts. Therefore, temperate phages may transform the phenotype of the host bacteria and all their progeny from avirulent/less virulent and antibiotic susceptible strains to highly virulent and antibiotic-resistant strains [Citation28,Citation29]. Appropriately professionally isolated and characterized phages must be used to prevent horizontal gene transfer of undesirable genes through phage-mediated biocontrol and phage therapy [Citation18,Citation30,Citation31]. Therefore, phages must be characterized morphologically by TEM and SDS PAGE protein profiling to establish their families or if they are novel phages followed by molecular characterization by WGS to confirm their families and to detect any integrase, toxin, and virulent genes in addition to antibiotic resistance genes by cross-referencing with known phage genomes, virulent factors, toxin genes, and antibiotic-resistant genes libraries. A cheaper but less-sensitive alternative to detect the presence of known integrase gene, virulent factors (VF) and genetic determinants of antibiotic resistance in phages is PCR amplification using conventional integrase gene VF, toxin genes, and antibiotic resistance genes primers. However, PCR amplification has limitations as it will not detect any possible novel VF and antibiotic resistance genes harbored by phages hence making molecular characterization of phages by WGS a prerequisite prior to phage-mediated biocontrol of bacterial growth and in vivo phage therapy [Citation32,Citation33]. However, only 12.6% (12) of the studies included in this review conducted WGS. Bioinformatics analyses and annotation demonstrated that myophages BɸC62 [Citation34], DL52, DL60 and DL680 [Citation35], DRA88 and phage K [Citation36], EcoInf [Citation37], coliphagesɸAPCEc01, ɸAPCEc02 and ɸAPCEc03 [Citation38], Phage P100 [Citation39], leB, leE and leN [Citation40]:, podophages DL54, DL 62 and DL 64 [Citation35]:, fHe-Yen3-01 [Citation41] and siphophages EcoSau [Citation37], phSE-1, phSE-2 and phSE-5 [Citation42], fHe-Yen3-01, fHe-Yen9-01, fHe-Yen9-02 and fHe-Yen9-03 [Citation41] were safe to use since they harbored no undesirable genes while siphophage HB10c2 had a gene encoding a putative beta-lactamase like protein [Citation43]. Additionally, PCR detected Stx I and II proteins encoding genes and lysogeny module genetic determinants in phages CB60P, MFA60N, CCO103, CBO103, and CCO113 [Citation44], Table S1. If such phages are used in phage therapy and phage-mediated biocontrol, they can facilitate the horizontal flow of undesirable genes. This exorbitantly underlines the importance of screening phages using very sensitive tools like WGS. Nevertheless, only 36.8% (39) research articles included in this review attempted to characterize phages; 3.2% (3) used PCR to detect VFs and lysogeny modules while only 12.6% (12) studies carried out WGS to fully illustrate the phage genomes indicating that there is still a big gap in ensuring phage therapy safety as per all the reviewed articles that were in English, though all the phages used for in vivo human phage therapy were previously characterized by committed phage research hubs. Furthermore, the morphology of phages was determined by transmission electron microscopy (TEM) in only 24.2% (23) studies. Basing on morphology, the phages belonged to various families as follows: Myoviridae; Siphoviridae; and Podoviridae in twenty, nine and ten studies respectively. Whereas, one study in each case reported phages as B1 morphology, Phage-like particle, and Cytoviridae family, Table S1.
7. Phage stability
Establishing the abiotic conditions affecting phage activity and/or viability was done in 16.8% (16) studies. This is an important criterion for selection since phage viability, occurrence, and storage are affected by temperature, pH, humidity, salinity, and other environmental conditions. Deviation from the favorable physicochemical factors can lead to the destruction of phages’ structural elements, protein envelope, and loss of genetic material thereby inactivating the phages [Citation45,Citation46]. These phages are isolated from natural environments such as sewage, hospital, and animal farm effluents, water bodies, foods, and beverages and evaluated for in vitro, in vivo, and ex vivo phage therapy and phage-mediated biocontrol where the prevailing physicochemical factors are completely different, . Hence, the need to establish the optimum conditions for the highest phage efficacy. However, such drawbacks can be mitigated by isolation of phages from local geographical locations and similar hosts as for in vivo phage therapy accompanied by assessing phage stability via exposing them to different physicochemical factors. Furthermore, during the preparation of commercial phage-based remedy, physicochemical properties are supposed to be investigated as they determine the shelf-life of phages [Citation47]. Despite that concern, only 9 (9.5%) and 7 (7.4%) out of 95 research articles included in this review evaluated the thermal and pH stability of phages, respectively, Table S1. This partly explains why some research articles reported very low or 0% phage efficacy in in vivo studies.
8. Specificity of phages
Specificity restricts phage infections to only certain bacteria with corresponding receptors to which they can bind; this determines the phage’s host range [Citation48]. For that reason, the application of phage therapy relies on an accurate characterization of all the strains, pathotypes, and serotypes of the target bacteria. Interestingly, if phage therapy overcomes the current obstacles hindering its approval universally, single phage and phage cocktail formulations must be designed indicating the pharmaceutical dosage and the phage host range for a given bacteria which calls for robust characterization of given target host bacteria. Conversely, this review identified gross deviation from the recommended procedure if meaningful phage therapy outcomes are to be achieved as only 55.8% (53) of studies reviewed attempted to use identified bacterial host strains, serovars, and pathotypes, . Worst still, no human in vivo phage therapy trial reported characterization of the target bacteria to their strains, pathotypes, and serotypes. Nevertheless, the spectrum and efficacy of phages can be enhanced by the use of phage cocktails. Phage cocktails also present another advantage of preventing phage resistance [Citation49,Citation50].
9. Multiplicity of infection (MOI)
MOI is defined as PFU/CFU ratio [Citation51]. MOI is an imperative factor to be considered for prospective phage therapy application. Increasing the PFU/CFU ratio enhances the probability of phage particles infecting their host bacteria. Therefore, in vivo and ex vivo phage therapies require higher MOIs than in vitro phage therapy as it is harder for phages to locate and infect their hosts within living tissues, surface of foods, and other materials being infected by phages. Some studies recommend an MOI of over 100 for ex vivo and in vivo phage therapy and less than 10 for in vitro phage therapy [Citation52]. This is in agreement with the studies incorporated in this review that reported MOI. The average MOI was highest in ex vivo experiments (557,291.8), followed by in vivo phage therapy (155,612.4) and in vitro phage biocontrol experiments had the lowest average MOI of 434.5 significantly different from ex vivo and in vivo MOIs, Table S1 and . Contrary to this, other studies disregard the term MOI as it only describes the phage quantities administered during dosing in relation to the population of the target bacteria but does not put into consideration the fact that; some phages fail to penetrate tissues/materials and get inactivated before adsorbing to the host cells, the host cell population is liable to change before phage application, the bacterial population may not easily be determined in case of infections and physicochemical factors such as temperature, pH, salinity, and humidity may inactivate phages before adsorption. As a result, MOI input may differ from the actual effective MOI [Citation53]. Furthermore, to increase the prospect of phages adhering and infecting their hosts; for experimental-induced infections a very high MOI of >105 is recommended [Citation54] whereas in vivo phage therapy of natural infection a very high titer value of > 1 × 108 PFU is appropriate as bacterial host cells are lysed by simply adsorption of phages before injection of their nucleic acids into the host cells and replication [Citation52,Citation54]. However, phages are immunogenic when applied at very high doses [Citation55]; therefore, the host immune system may identify and inactivate them. Additionally, the MOI against biofilm infections should be higher as indicated by the studies reviewed which compared optimum MOI against bacterial suspension or free-living bacteria to that against biofilms and/or immobile bacteria, Table S1 and . In in vitro experiments, MOIs of 0.1, 1, and 10; and 100, 1,000, and 10,000 [Citation56]; 0.1 and 10 [Citation36] were administered against bacterial planktons and biofilms, respectively. It is worth mentioning that in addition to high MOI, the most suitable phages for phage-mediated management of biofilm infections should encode polysaccharide depolymerase which degrades the biofilm polysaccharide matrix to ease phage interaction with the host cells in the lower layers of the matrix [Citation57].
Figure 2. Comparison of mean MOIs between in vivo, in vitro, and ex vivo phage therapy. Tukey’s multiple-comparison test was used to compute and compare MOIs P value of 0.0002 < 0.05 generated indicating significant variation between ex vivo/in vivo PT and in vitro PT
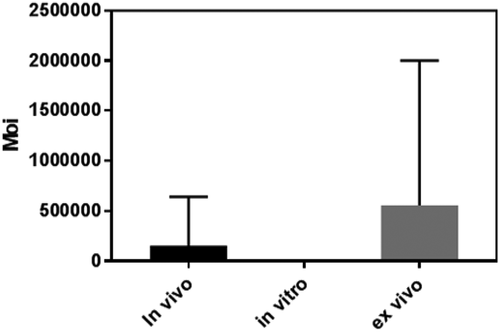
Figure 3. Comparison of phage therapy (PT), phage-mediated biocontrol/diagnosis mean efficacy percentages. Tukey’s multiple-comparison test was used to calculate and compare the mean percentage efficacies generating a P value of 0.148 > 0.05 after exclusion of fields with less than three studies (water, piggery poultry, and apiculture)
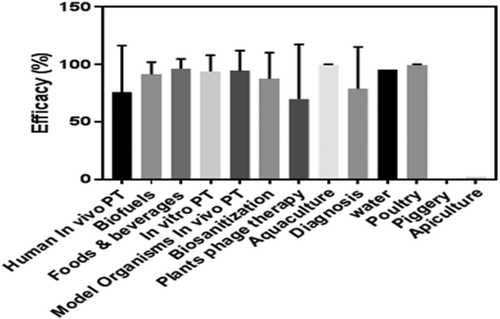
10. Efficacy of phage therapy against drug resistant and sensitive bacterial infections and isolates
In vivo human phage therapy studies reported mixed levels of efficacy ranging from 0% to 100%. The mode and median efficacies were 100% while Tukey’s multiple comparison test generated a P value of 0.009 < 0.05 indicating that phage therapy efficacies of 100% were more pronounced than efficacies lower than 100% in all the in vivo human phage therapy. Interestingly, efficacies of 100% were scored when treating MRSA diabetic foot ulcers, GIT MRSA infection, VRSA corneal abscess and interstitial keratitis, and MDR Pseudomonas aeruginosa UTI with phages. Furthermore, in vivo phage treatment of MRSA osteomyelitis in Rabbits [Citation58], carbepenem resistant Acinetobacter baumanii infection in mice [Citation34], MDR Acinetobacter baumanii pneumonia in mice [Citation59] and pan drug resistant (PDR) Acinetobacter. baumannii infections in mice [Citation60] provided 100% protection to model animals against the super bugs while in vivo phage therapy of MDR Pseudomonas aeruginosa ulcerative lesions in catfish species achieved 100% success. It is also worth noting that ex vivo phage therapy against MDR Pseudomonas aeruginosa skin infections, MRSA biofilms induced onto porcine skin burns, and PDR Acinetobacter baumanni human HeLa cells infections recorded overwhelming success. In vitro phage therapy against MRSA, MDR Acinetobacter baumanni, MDR Pseudomonas aeruginosa scored an inhibitory efficacy ranging from 78% to 100% with an average of 95.4%. Data from around the globe show an overall decline in the total reserves of antibiotics efficacy: resistance to all first-line and last-resort antibiotics is increasing [Citation3]. For instance, in sub-Saharan Africa, India, Latin America, and Australia, MRSA incidence is still intensifying [Citation3,Citation61Citation62], and estimated at 47% in India in 2014, and 90% in Latin American hospitals in 2013 [Citation61][]. MRSA causes 35–46% of wound complication in Mulago referral hospital [Citation63,Citation64]. The increased prevalence of community acquired E. coli isolates coding for extended-spectrum beta lactamases competent of hydrolyzing approximately all beta lactams antibiotic except carbapenems has been reported globally [Citation65]. In more than a decade, carbapenem resistance in Enterobacteriaceae bacteria has been observed yet Carbapenems such as imipenem, ertapenem, meropenem, and doripenem are the newest synthesized molecules with the broadest spectrum of activity and consequently considered the first-line therapy antibiotics in the treatment of multi-resistant gram-negative bacterial infections [Citation66,Citation67]. The magnitude of MDR Pseudomonas aeruginosa and Acinetobacter baumannii is a great threat to the health sector worldwide [Citation68]. The promising outcomes of in vivo, ex vivo, and in vitro phage therapy of MDR bacterial infections and isolates exhibit that phage therapy if employed appropriately is more effective than antibiotics and therefore can replace or supplement antibiotics as a routine in the management of both resistant and sensitive bacterial infections. However, limited success was attained when treating S. aureus and P. aeruginosa wound infection in humans, acute human E. coli infections, MRSA nasal infections in pigs and American foulbrood caused by Paenibacillus larvae [Citation43,Citation69–71]. This is in contrary to the in vitro experiments carried out in two of the studies where total eradication of the bacteria was achieved [Citation43,Citation71]. This can be attributed to the change in physiological conditions: loss of phage viability due to deviation from their optimum temperature and pH in unnatural environments [Citation46].
11. Endolysins versus phage particles
Phages code tail spike proteins for identification and adhesion to receptors on the host cell surface. The tail spikes proteins are often incorporated with peptidoglycan hydrolases that locally hydrolyze the bacterial cell wall peptidoglycan, thus creating an opening for injection of phage nucleic acids which marks the initiation of the infection process [Citation72]. An additional type of phage-derived enzymes; the peptidoglycan hydrolases called endolysins degrade the peptidoglycan liberating the progeny virions from the host cell at the end of the lytic phage cycle [Citation73]. Gram-positive bacteria do not possess a shielding outer layer thereby making exogenous application of endolysins achieve speedy and effective lysis. This property makes endolysins promising possible alternative antimicrobial agents [Citation23]. Several studies have reported endolysins as potential therapeutic agents with high efficacy and safety [Citation74]. In addition, endolysins possess an added advantage over conventional antibiotics as; they exhibit great specificity exerting selective pressure on target pathogenic bacteria populations [Citation75,Citation76], emergence of resistance against endolysins is implausible given that phage (endolysins) coevolve with their host bacteria, the host receptor site where endolysins bind are highly conserved thereby making their alteration highly detrimental to the host bacterium [Citation76,Citation77]. Furthermore, endolysins degrade the cell wall externally without the burden of entering the bacterial cell hence evading the common antibiotic resistance mechanisms such as the active efflux pump and decreased membrane permeability [Citation78]. A lot of ethical and safety concerns have been vehemently expressed about the use of live viruses as therapeutics in the treatment of bacterial infections; currently, the immediate hope lies in the use of phage endolysins in the near future to combat the increasing antibiotic resistance. Fortunately, to meet the high demand, endolysins can be produced using recombinant DNA technology [Citation79–81]. This review compared the use of phages and endolysins to suppress bacterial growth during ethanol fermentation. Phages demonstrated superior efficacy than recombinant phage endolysins with mean efficacy of 99.5% for phages and 83.6% for phage endolysins but not significantly divergent as revealed by one-way ANOVA (P value of 0.13 > 0.05). This clearly supports the use of phages and endolysins hand in hand as therapeutic agents.
12. Application of phages in Biosanitization and Biopreservation
Infectious food and water-associated diseases are the major causes of mortality and morbidity worldwide [Citation6,Citation7]. Irrational use of antibiotics in livestock has resulted in antibiotic resistance which spillover to humans through contaminated food, water, and environment [Citation3–5,Citation67]. Fortunately, in 2006 the US Food and Drug Administration (FDA) approved the utilization of 6 independently purified LMP-102 phages as biopreservative antimicrobial agents in RTE meat and poultry products against Listeria monocytogenes [Citation82]. In this review, the literature search yielded 21 (22.1%) research articles reporting foods and beverages phage-mediated bio-preservation with average, mode, median efficacy of 96.5%, 100%, and 100%, respectively. In a water decontamination study, phages eradicated 95.4% of the coliform. This is a clear indicator of the potency of phages as bio-preservative and bio-decontamination agents and consequently their approval to preserve food and decontaminate water following robust characterization should be considered to prevent transmission of antibiotic-resistant and susceptible food and water-associated infection.
Furthermore, the hospital environment polluted by infected patients with antibiotic-resistant bacteria is incriminated as the main route of transmission [Citation83,Citation84]. This has been a result of the emergence of bacterial resistance to the conventional disinfectants [Citation83]. The possibility of a horizontal flow of mobile genetic elements encoding antibiotic resistance from clinical to environmental bacteria within the hospital is high hence advancing the evolution of new antibiotic-resistant bacterial strains [Citation85]. On a good note, bio-disinfection using phages as demonstrated by this review is promising: for instance, phage-mediated bio-sanitization eradicated 90% of Staphylococcus aureus, Escherichia coli, and Pseudomonas aeruginosa tainted on plastic, glass, and ceramic materials mimicking hospital surfaces [Citation86] while phage-mediated sterilization trial of the ICU reduced the prevalence of carbapenem-resistant Acinetobacter baumanii by 47.5% [Citation87]. In another phage sanitization trial, phages completely eliminated staphylococci, streptococci, enterococci, proteus, Klebsiella pneumoniae, Klebsiella oxytoca, Pseudomonas aeruginosa, and Escherichia coli from the hospital environment [Citation88] while phage-based sanitization cream completely inhibited MRSA and Propionibacterium acnes growth [Citation89,Citation90]. With those laudable bio-sanitization results, the use of phages to complement conventional disinfection strategies could exhibit valuable outcomes.
13. Phages and endolysin as alternative antibacterial decontamination agents
Lactic acid bacteria (LAB) are by far the commonest bacterial contaminants of biofuel production facilities and are believed to hamper the ethanol fermentation process hence limiting ethanol production. Ethanol fermentation presents an environment of high ethanol concentration, low pH, and low oxygen concentration thereby favoring the growth of Lactobacillus sp which are well adapted to survive under such conditions. Currently, there is no appropriate strategy to combat ethanol loss due to LAB contamination as all possible measures have limitations [Citation91]. Contrary to that, the four experimental studies which employed phages and endolysins to control LAB growth during ethanol fermentation analyzed in this review demonstrated eye-catching bacterial growth suppression outcomes with mean efficacy of 91.6%. Most importantly, phage and endolysins mediated ethanol fermentation facility decontamination restored normal ethanol yield without losing their viability [Citation37,Citation92]. Because of the promising results, to eliminate the use of antibiotics for decontamination in the ethanol fermentation business, phages and endolysins should be considered as alternatives.
14. Limitations
Hypothetically, all bacteria can be lysed by at least one type of bacteriophage. In the light of this, phages are considerably more efficacious than antibiotics. However, phage antibacterial applications have limitations. Most phages have demonstrated a broad spectrum hence can lyse both the target pathogenic strains and potentially beneficial bacterial strains. Additionally, it is difficult to isolate phages without any undesirable genes such as antibiotic-resistant genes, bacterial virulent genes, and integrase genes. Phages with such genes may contribute to the development of highly pathogenic antimicrobial-resistant bacteria. Furthermore, phage-based therapeutic formulation and stabilization is still a challenge as previous studies reported that the stability of phage formulations for clinical use is stringently influenced by the phage type. Thus, each phage type requires its unique stabilization strategy and this is extremely complicated for phage cocktail formulations. The evolution of bacterial resistance against phages mainly mediated by loss or alteration of the bacterial phage receptors and bacterial secretions that prevent phage adsorption has been implicated as another limitation affecting phage therapy. Inactivation of phages by the immune system has also been reported as a drawback of phage therapy.
15. Conclusion
The high prevalence of MDR infections has resulted in familiar bacterial diseases becoming difficult to treat. Moreover, hospital-associated infections (both sensitive and MDR) are mainly acquired through contaminated surfaces and medical equipment. However, phage-mediated bio-sanitization, in vivo, ex vivo, and in vitro phage therapy experiments and trials analyzed by this review showed that phages can mitigate the burden caused by MDR infections and contamination of hospital surfaces as well as medical devices. Furthermore, water and food-borne bacterial infections have been implicated as the major cause of mortality and morbidity globally and LAB as the main cause of yield loss in the biofuels fermentation industry. Analysis of phage/endolysin mediated bio-preservation and bio-decontamination studies by this review showed that phages and endolysins were highly effective. Thus, phage technology presents an opportunity for developing alternative therapeutic, bio-preservative, bio-decontamination, and bio-sanitization approaches. Despite the undisputable efficacy of phage therapy and phage-mediated biocontrol, rigorous investigations using highly sensitive techniques should be carried out to ensure that only appropriate professionally lytic and safe phages are used. Thus, for low- and middle-income countries, there is a need to develop affordable and appropriate methods for screening of phages for undesirable genes. Moreover, the challenge of immunogenicity that may be associated with in vivo application of phages needs to be explored further.
Ethics and consent to participate:
Not applicable
Consent for publication:
Not applicable
Availability of Data and Materials:
Supplementary data summarized in tabular form have been submitted with the manuscript.
Authors’ contributions
This work was carried out in collaboration between all authors. Jesca L. Nakavuma (JLN), Dennis K Byarugaba (DKB), Robert Tweyongyere (RB), and Francis Ejobi (FB) conceptualized this project and designed the format for this review. Kenneth Ssekatawa (KS), Edward Wampande (EW), Charles Kato Drago (CKD) & JLN performed the literature search and data analysis. All authors drafted the section of the literature review. KS, JLN, and CKD wrote the first draft of the manuscript and managed manuscript revisions. All authors read and approved the final manuscript.
Supplemental Material
Download MS Word (57.8 KB)Acknowledgements
We are thankful to MAPRONANO ACE for funding this work.
Disclosure statement
The authors declare that they have no competing interests.
Supplementary material:
Supplemental data for this article can be accessed here.
Additional information
Funding
Notes on contributors
Kenneth Ssekatawa
Kenneth Ssekatawa is a PhD candidate at Makerere University and Lecturer Department of Biochemistry, Kampala International University. Kenneth specializes in molecular microbiology, antimicrobial resistance and nanobiotechnology.
Denis K. Byarugaba
Prof. Denis K. Byarugaba He is a Professor of Microbiology, College of Veterianary Medicine Animal Resources and Biosecurity, Makerere University. He is currently involved in research on influenza viruses and highly pathogenic pathogens with potential for causing pandemic threats and antimicrobial resistance.
Charles D. Kato
Dr. Charles D. Kato is a lecturer at college of Veterinary Medicine Animal Resources and Biosecurity. Charles' research interests are in biomark research regarding disease co-infection and host-parasite interactions in infectious and zoonotic diseases. Charles is conducting research in phage mediated therapy.
Eddie M. Wampande
Dr. Eddie M. Wampande currently works as a senior lecturer College Veterinary Animal Resources and Biosecurity, Makerere University. He specializes in Microbiology and Parasitology.
Francis Ejobi
Prof. Francis Ejobi is a Professor of Microbiology, Makerere University specializing in infectious disease, epidemiology and antimicrobial resistance
Robert Tweyongyere
Prof. Robert Tweyongyere Robert is a research fellow at the Uganda Virus Research Institute, Entebbe and a Senior Lecturer at College of Veterinary Medicine Animal Resources and Biosecurity Makerere University. His research interests are in infectious diseases.
Jesca L. Nakavuma
Dr. Jesca L. Nakavuma Jesca works as a senior lecturer and microbiologist, Department of Biomolecular and Biolaboratory Sciences, College of Veterinary Medicine Animal Resources and Biosecurity, Makerere University. She is the Founder Chair Phage Team Uganda and currently working on a project aimed at using phage therapy to control and treat bacterial infections in fish.
References
- CDC, Facility Guidance for Control of Carbapenem-resistant Enterobacteriaceae (CRE) 2015.
- UNAS. Antibiotic resistance in Uganda: situation analysis and recommendations. Uganda National Academy of Science; 2015.
- Centre for Disease Dynamics Economics and Policy-CDDEP. The state of the world’s antibiotics. Washington DC-New Delhi; 2015.
- Economou V, Gousia P. Agriculture and food animals as a source of antimicrobial-resistant bacteria. J Infec drug resist. 2015;8: 49.
- Warnes SL, Highmore CJ, Keevil CW. Horizontal transfer of antibiotic resistance genes on abiotic touch surfaces: implications for public health. 2012 3(6); J MBio.
- World Health Organization., World health organization estimates of the global burden of foodborne diseases. WHO, Geneva, Switzerland (2015). http://apps.who.int
- Lewin S, et al. Estimating the burden of disease attributable to unsafe water and lack of sanitation and hygiene in South Africa in 2000. S. Afr Med Journal; 2007;97(8):755–762.
- Fenwick AJS. Waterborne infectious diseases—could they be consigned to history? 2006;313(5790):1077–1081.
- Shlaes DM. The perfect storm, in antibiotics. The Netherlands: Springer; 2010. p. 1–7.
- Guttman B, Raya R, Kutter EJBBA. Basic phage biology. 4 (2005).
- Abedon S. Bacteriophage clinical use as antibacterial “drugs”: utility and precedent. Microbiol. Spectr. 2017;5:BAD-0003-2016.
- Kutateladze M, Adamia R. Bacteriophages as potential new therapeutics to replace or supplement antibiotics. G. Eliava Institute of Bacteriophages. Trends Biotechnol. 2010;28(12):591–595.
- Silva YJ, et al. Phage therapy as an approach to prevent Vibrio anguillarum infections in fish larvae production. 2014;9(12):e114197.
- Cortés ME, Bonilla JC, Sinisterra RD. Biofilm formation, control and novel strategies for eradication. 2011;2:896–905. %J Sci Against Microbial Pathog Commun Curr Res Technol Adv.
- Abedon S. Bacteriophages and biofilms: ecology. 2011;557: J Phage Therapy Plaques.
- Hughes KA, Sutherland IW, Jones M. Biofilm susceptibility to bacteriophage attack: the role of phage-borne polysaccharide depolymerase. 1998;144(11):3039–3047. V %J Microbio.
- Wittebole X, De Roock S, Opal S. A historical overview of bacteriophage therapy as an alternative to antibiotics for the treatment of bacterial pathogens. M %J Virulence. 2014;5(1):226–235.
- Borysowski J, Międzybrodzki R, Górsk A. Phage therapy: current research and applications. Caister Academic Press; 2014.
- Chan BK, et al. Phage treatment of an aortic graft infected with Pseudomonas aeruginosa. 2018;2018(1):60–66.
- Kazi M, Annapure US. Bacteriophage biocontrol of foodborne pathogens. J food sci tech. 2016;53(3):1355–1362.
- Roy B, et al. Biological inactivation of adhering Listeria monocytogenes by listeriaphages and a quaternary ammonium compound. 1993;59(9):2914–2917.
- Greer G. Effects of phage concentration, bacterial density, and temperature on phage control of beef spoilage. J Food Sci. 1988;53(4):1226–1227.
- Fischetti VA. Bacteriophage endolysins: a novel anti-infective to control Gram-positive pathogens. Int J Med Microbiol. 2010;300(6):357–362.
- Le TS, et al. Bacteriophages as biological control agents of enteric bacteria contaminating edible oysters. 2018;75(5):611–619.
- Hudson J, et al. Bacteriophages as biocontrol agents in food. 2005;68(2):426–437.
- Chatain-LY MH. The factors affecting effectiveness of treatment in phages therapy. 2014;5:51. J Frontiers microbio.
- Gill J, Abedon STJAF. Bacteriophage ecology and plants. 2003;1–17.
- Colomer-Lluch M, Jofre J, Muniesa M. Antibiotic resistance genes in the bacteriophage DNA fraction of environmental samples. PLoS One. 2011;6(3):e17549.
- Faruque S, Mekalanos J. Phage-bacterial interactions in the evolution of toxigenic Vibrio cholerae. Virulence. 2012;3(7):556–565.
- McCallin S, et al. Safety analysis of a Russian phage cocktail: from metagenomic analysis to oral application in healthy human subjects. 2013;443(2):187–196.
- Gill JJ, Hyman PJCPB. Phage choice, isolation, and preparation for phage therapy. 2010;11(1):2–14.
- Hagens S, Loessner MJJCPB. Bacteriophage for biocontrol of foodborne pathogens: calculations and considerations. 2010;11(1):58–68.
- Mahony J, et al. Bacteriophages as biocontrol agents of food pathogens. 2011;22(2):157–163.
- Jeon J, et al. In vivo application of bacteriophage as a potential therapeutic agent to control OXA-66-like carbapenemase-producing Acinetobacter baumannii strains belonging to sequence type 357. 2016;82(14):4200–4208.
- Alves DR, et al. A novel bacteriophage cocktail reduces and disperses P seudomonas aeruginosa biofilms under static and flow conditions. J Microbial biotech. 2016;9(1):61–74.
- Alves D, et al. Combined use of bacteriophage K and a novel bacteriophage to reduce Staphylococcus aureus biofilm formation. J App Environ Microbio. 2014;80(21):6694–6703.
- Liu M, et al. Bacteriophage application restores ethanol fermentation characteristics disrupted by Lactobacillusfermentum. 2015;8(1):132.
- Dalmasso M, Strain R, Neve H, et al. Three new Escherichia coli phages from the human gut show promising potential for phage therapy. PLoS One. 2016;11(6):e0156773. .
- Carlton RM, Noordman WH, Biswas B, et al. Bacteriophage P100 for control of Listeria monocytogenes in foods: genome sequence, bioinformatic analyses, oral toxicity study, and application. Regul Toxicol Pharmacol. 2005;43(3):301–312. .
- Endersen L, Buttimer C, Nevin E, et al. Investigating the biocontrol and anti-biofilm potential of a three phage cocktail against Cronobacter sakazakii in different brands of infant formula. Int J Food Microbiol. 2017;253:1–11.
- Jun JW, et al. Bacteriophages reduce Yersinia enterocolitica contamination of food and kitchenware. 2018;271:33–47.
- Pereira C, et al. Bacteriophages with potential to inactivate Salmonella Typhimurium: use of single phage suspensions and phage cocktails. 2016;220:179–192.
- Beims H, et al. Paenibacillus larvae-directed bacteriophage HB10c2 and its application in American foulbrood-affected honey bee larvae. 2015;81(16):5411–5419.
- Dini C, De Urraza PJ. Isolation and selection of coliphages as potential biocontrol agents of enterohemorrhagic and Shiga toxin-producing E. coli (EHEC and STEC) in cattle. J Appl Microbiol. 2010;109(3):873–887.
- Ackermann H-W, Tremblay D, Moineau S. Long-term bacteriophage preservation. 2004;38:35e40. WFCC Newsletters.
- Jończyk E, et al. The influence of external factors on bacteriophages. 2011;56(3):191–200.
- Vandenheuvel D, Lavigne R, Brüssow H. Bacteriophage therapy: advances in formulation strategies and human clinical trials. Annu Rev Virol. 2015;2(1):599–618.
- Hyman P, Abedon ST. Bacteriophage host range and bacterial resistance, in advances in applied microbiology. Elsevier; 2010. p. 217–248.
- Markoishvili K, et al. A novel sustained‐release matrix based on biodegradable poly (ester amide) s and impregnated with bacteriophages and an antibiotic shows promise in management of infected venous stasis ulcers and other poorly healing wounds. 2002;41(7):453–458.
- Jikia D, et al. The use of a novel biodegradable preparation capable of the sustained release of bacteriophages and ciprofloxacin, in the complex treatment of multidrug‐resistant Staphylococcus aureus‐infected local radiation injuries caused by exposure to Sr90. 2005;30(1):23–26.
- O’flynn G, et al. Evaluation of a cocktail of three bacteriophages for biocontrol of Escherichia coli O157: H7. J Applied Environ Microbio. 2004;70(6):3417–3424.
- Johnson R, et al. Bacteriophages for prophylaxis and therapy in cattle, poultry and pigs. 2008;9(2):201.
- Abedon S. Phage therapy dosing: the problem(s) with multiplicity of infection (MOI). Bacteriophage. 2016;6(3):e1220348.
- Bach SJ, et al. Effect of bacteriophage DC22 on Escherichia coli O157: H7 in an artificial rumen system (Rusitec) and inoculated sheep. 2003;52(2):89–101.
- Majewska J, et al. Oral application of T4 phage induces weak antibody production in the gut and in the blood. 2015;7(8):4783–4799.
- Chen L-K, et al. Potential of bacteriophage ΦAB2 as an environmental biocontrol agent for the control of multidrug-resistant Acinetobacter baumannii. 2013;13(1):1–10.
- Sutherland IW, et al. The interaction of phage and biofilms. 2004;232(1):1–6.
- Kishor C, et al. Phage therapy of staphylococcal chronic osteomyelitis in experimental animal model. Indian J Med Res. 2016;143(1):87–94. .
- Wang Y, et al. Intranasal treatment with bacteriophage rescues mice from Acinetobacter baumannii-mediated pneumonia. 2016;11(5):631–641.
- Yin S, Huang G, Zhang Y, et al. Phage Abp1 rescues human cells and mice from infection by pan-drug resistant Acinetobacter Baumannii. Cell Physiol Biochem. 2017;44(6):2337–2345. .
- EARS-NET., European antimicrobial resistance surveillance network (EARS-Net). 2014. EARS-Net Report,Quarters 1–4. Dublin. 2014. This is a permanent publication in PDF, thus access date not require
- Kateete DP, et al. High prevalence of methicillin resistant Staphylococcus aureus in the surgical units of Mulago hospital in Kampala, Uganda. BMC Res Notes. 2011;4(1):326.
- Seni J, et al. Molecular characterization of Staphylococcus aureus from patients with surgical site infections at Mulago Hospital in Kampala, Uganda. PLoS One. 2013;8(6):e66153.
- Pitout JD, Laupland KB. Extended-spectrum beta-lactamase-producing Enterobacteriaceae: an emerging public-health concern. Lancet Infect Dis. 2008;8(3):159–166.
- EFSA Panel on Biological Hazards. Scientific Opinion on Carbapenem resistance in food animal ecosystems. J EFSA. 2013;11(12):3501.
- Nordmann P, Dortet L, Poirel LJTIMM. Carbapenem resistance in Enterobacteriaceae: here is the storm! 2012;18(5):263–272.
- CDC, Antibiotic resistance threats in the United States. 2013.
- Rose T, et al. Experimental phage therapy of burn wound infection: difficult first steps. 2014;4(2):66.
- Sarker S, et al. Oral phage therapy of acute bacterial diarrhea with two coliphage preparations: a randomized trial in children from Bangladesh. 2016;4:124–137.
- Verstappen KM, et al. The effectiveness of bacteriophages against methicillin-resistant Staphylococcus aureus ST398 nasal colonization in pigs. PLoS One. 2016;11(8):e0160242.
- Rodríguez-Rubio L, et al. Bacteriophage virion-associated peptidoglycan hydrolases: potential new enzybiotics. Crit Rev Microbiol. 2013;39(4):427–434. .
- Young R. Phage lysis: do we have the hole story yet? Curr Opin Microbiol. 2013;16(6):790–797.
- Haddad Kashani H, et al. Recombinant endolysins as potential therapeutics against antibiotic-resistant <span class=“named-content genus-species” id=“named-content-1”>Staphylococcus aureus</span>: current status of research and novel delivery strategies. 2018;31(1):e00071–17.
- García P, Rodríguez L, Rodríguez A, et al. Food biopreservation: promising strategies using bacteriocins, bacteriophages and endolysins. Trends Food SciTechnol. 2010;21(8):373–382. .
- Borysowski J, Weber-Dąbrowska B, Górski A, et al. Bacteriophage endolysins as a novel class of antibacterial agents. Exp Biol Med (Maywood). 2006;231(4):366–377. .
- Kusuma C, Jadanova A, Chanturiya T, et al. Lysostaphin-resistant variants of Staphylococcus aureus demonstrate reduced fitness in vitro and in vivo. Antimicrob Agents Chemother. 2007;51(2):475–482. .
- Viertel T, Ritter K, Horz HP. Viruses versus bacteria-novel approaches to phage therapy as a tool against multidrug-resistant pathogens. J Antimicrob Chemother. 2014;69(9):2326–2336.
- Mao J, et al. Chimeric Ply187 endolysin kills Staphylococcus aureus more effectively than the parental enzyme. 2013;342(1):30–36.
- Yang H, Yu J, Wei H. Engineered bacteriophage lysins as novel anti-infectives. 5(542):2014.
- Donovan D, Foster-Frey J. LambdaSa2 prophage endolysin requires Cpl-7-binding domains and amidase-5 domain for antimicrobial lysis of streptococci. FEMS Microbiol Lett. 2008;287(1):22–33.
- Lang LH. FDA approves use of bacteriophages to be added to meat and poultry products. Gastroenterology. 2006;131(5):1370.
- de Oliveira AC, Damasceno QS. [Surfaces of the hospital environment as possible deposits of resistant bacteria: a review]. Rev Esc Enferm USP. 2010;44(4):1118–1123.
- French GL, et al. Tackling contamination of the hospital environment by methicillin-resistant Staphylococcus aureus (MRSA): a comparison between conventional terminal cleaning and hydrogen peroxide vapour decontamination. J Hosp Infect. 2004;57(1):31–37. .
- Ssekatawa K, et al. A systematic review: the current status of carbapenem resistance in East Africa. BMC Res Notes. 2018;11(1):629. .
- D’Accolti M, et al. Efficient removal of hospital pathogens from hard surfaces by a combined use of bacteriophages and probiotics: potential as sanitizing agents. 2018;11:1015.
- Ho YH, et al. Application of bacteriophage-containing aerosol against nosocomial transmission of carbapenem-resistant acinetobacter baumannii in an intensive care unit. PLoS One. 2016;11(12):e0168380. .
- Akimkin V, Shestopalov N, Shumilov V, et al. ICPIC, meeting abstracts from international conference on prevention & infection control (ICPIC 2017), biological disinfection with bacteriophages. Antimicrob Resist Infect Control. 2017;6(3):52.
- O’flaherty S, et al. Potential of the polyvalent anti-Staphylococcus bacteriophage K for control of antibiotic-resistant staphylococci from hospitals. 2005;71(4):1836–1842.
- Brown TL, et al. The formulation of bacteriophage in a semi solid preparation for control of propionibacterium acnes growth. PLoS One. 2016;11(3):e0151184. .
- Beckner M, Ivey ML, Phister TG. Microbial contamination of fuel ethanol fermentations. 2011;53(4):387–394.
- Silva J, Sauvageau D. Bacteriophages as antimicrobial agents against bacterial contaminants in yeast fermentation processes. J Biotechn biofuels. 2014;7(1):123.
- Fish R, et al. Bacteriophage treatment of intransigent diabetic toe ulcers: a case series. 2016;25(Sup7):S27–S33.
- Leszczyński P, et al. Successful eradication of methicillin-resistant Staphylococcus aureus (MRSA) intestinal carrier status in a healthcare worker–case report. Folia Microbiol (Praha). 2006;51(3):236–238. .
- Marza JAS, et al. Multiplication of therapeutically administered bacteriophages in Pseudomonas aeruginosa infected patients. Burns. 2006;32(5):644–646. .
- Fadlallah A, Chelala E, Legeais J-MJTOOJ. Corneal infection therapy with topical bacteriophage administration. 2015;9:167.
- Wright A, et al. A controlled clinical trial of a therapeutic bacteriophage preparation in chronic otitis due to antibiotic‐resistant Pseudomonas aeruginosa; a preliminary report of efficacy. 2009;34(4):349–357.
- Khawaldeh A, et al. Bacteriophage therapy for refractory Pseudomonas aeruginosa urinary tract infection. J Med Microbiol. 2011;60(Pt 11):1697–1700. .
- Letkiewicz S, et al. Eradication of Enterococcus faecalis by phage therapy in chronic bacterial prostatitis—case report. 2009;54(5):457.
- Roach DR, et al. Bacteriophage-encoded lytic enzymes control growth of contaminating Lactobacillus found in fuel ethanol fermentations. 2013;6(1):20.
- Khatibi PA, et al. Saccharomyces cerevisiae expressing bacteriophage endolysins reduce Lactobacillus contamination during fermentation. 2014;7(1):104.
- Obeso JM, et al. Use of logistic regression for prediction of the fate of Staphylococcus aureus in pasteurized milk in the presence of two lytic phages. Appl Environ Microbiol. 2010;76(18):6038–6046. .
- Lee Y-D, Park J-H. Characterization and application of phages isolated from sewage for reduction of Escherichia coli O157:H7 in biofilm. Lebensmittel-Wissenschaft + [i.e. und] Tech. 2015;60(no. 1):571–577–2015.
- Tomat D, Mercanti D, Balagué C, et al. Phage biocontrol of enteropathogenic and Shiga toxin-producing Escherichia coli during milk fermentation. Lett Appl Microbiol. 2013;57(1):3–10. .
- Guenther S, Loessner MJ. Bacteriophage biocontrol of Listeria monocytogenes on soft ripened white mold and red-smear cheeses. J App Bacteri. 2011;1(2):94–100.
- Vonasek EL, et al. Incorporating phage therapy into WPI dip coatings for applications on fresh whole and cut fruit and vegetable surfaces. 2018;83(7):1871–1879.
- Leverentz B, et al. Biocontrol of Listeria monocytogenes on fresh-cut produce by treatment with lytic bacteriophages and a bacteriocin. 2003;69(8):4519–4526.
- Guenther S, et al. Virulent bacteriophage for efficient biocontrol of Listeria monocytogenes in ready-to-eat foods. J App environ microbio. 2009;75(1):93–100.
- Deasy T, et al. Isolation of a virulent Lactobacillus brevis phage and its application in the control of beer spoilage. 2011;74(12):2157–2161.
- Augustine J, Bhat SG. Biocontrol of Salmonella Enteritidis in spiked chicken cuts by lytic bacteriophages ΦSP‐1 and ΦSP‐3. J basic microbio. 2015;55(4):500–503.
- Patel J, et al. Inactivation of Escherichia coli O157: H7 attached to spinach harvester blade using bacteriophage. 2011;8(4):541–546.
- Jun JW, et al. Eating oysters without risk of vibriosis: application of a bacteriophage against Vibrio parahaemolyticus in oysters. 2014;188:31–35.
- Bandara N, et al. Bacteriophages BCP1-1 and BCP8-2 require divalent cations for efficient control of Bacillus cereus in fermented foods. 2012;31(1):9–16.
- Lone A, et al. Development of prototypes of bioactive packaging materials based on immobilized bacteriophages for control of growth of bacterial pathogens in foods. 2016;217:49–58.
- Kim KP, Klumpp J, Loessner MJ. Enterobacter sakazakii bacteriophages can prevent bacterial growth in reconstituted infant formula. Int J Food Microbiol. 2007;115(2):195–203.
- Huang C, et al. Isolation, characterization, and application of a novel specific Salmonella bacteriophage in different food matrices. Food Res Int. 2018;111:631–641.
- Gouvêa DM, et al. Acetate cellulose film with bacteriophages for potential antimicrobial use in food packaging. 2015;63(1):85–91.
- Gouvêa DM, et al. Absorbent food pads containing bacteriophages for potential antimicrobial use in refrigerated food products. 2016;67:159–166.
- Pereira C, et al. Application of phage therapy during bivalve depuration improves Escherichia coli decontamination. 2017;61:102–112.
- Ryan EM, et al. Synergistic phage-antibiotic combinations for the control of Escherichia coli biofilms in vitro. 2012;65(2):395–398.
- Kusmiatun A, Rusmana I, Budiarti S. Characterization of bacteriophage specific to bacillus pumilus from Ciapus River in Bogor, West Java, Indonesia. HAYATI J Biosci. 2015;22(1):27–33.
- Kvachadze L, et al. Evaluation of lytic activity of staphylococcal bacteriophage Sb‐1 against freshly isolated clinical pathogens. 2011;4(5):643–650.
- Sillankorva S, Neubauer P, Azeredo JJBB. Pseudomonas fluorescens biofilms subjected to phage phiIBB-PF7A. 2008;8(1):79.
- Jung L-S, et al. Evaluation of lytic bacteriophages for control of multidrug-resistant Salmonella Typhimurium. 2017;16(1):1–9.
- Alves DR, et al. Development of a high-throughput ex-vivo burn wound model using porcine skin, and its application to evaluate new approaches to control wound infection. J Frontiers cellular infec microbio. 2018;8:196.
- Ghosh A, et al. Combined application of essential oil compounds and bacteriophage to inhibit growth of Staphylococcus aureus in vitro. 2016;72(4):426–435.
- Lungren MP, et al. Bacteriophage K for reduction of Staphylococcus aureus biofilm on central venous catheter material. 2013;3(4):e26825.
- Carson L, Gorman S, Gilmore B. The use of lytic bacteriophages in the prevention and eradication of biofilms of Proteus mirabilis and Escherichia coli. 2010;59(3):447–455.
- Nouraldin AAM, et al. Bacteriophage-antibiotic synergism to control planktonic and biofilm producing clinical isolates of Pseudomonas aeruginosa. 2016;52(2):99–105.
- Vipra AA, et al. Antistaphylococcal activity of bacteriophage derived chimeric protein P128. 2012;12(1):41.
- Chhibber S, Nag D, Bansal SJBM. Inhibiting biofilm formation by Klebsiella pneumoniae B5055 using an iron antagonizing molecule and a bacteriophage. 2013;13(1):1–8.
- Abdulamir AS, et al. The potential of bacteriophage cocktail in eliminating Methicillin-resistant Staphylococcus aureus biofilms in terms of different extracellular matrices expressed by PIA, ciaA-D and FnBPA genes. 2015;14(1):49.
- Didamony GE, Askora A, Shehata AA. Isolation and characterization of T7-Like Lytic Bacteriophages Infecting multidrug resistant pseudomonas aeruginosa isolated from Egypt. Curr Microbiol. 2015;70(6):786–791.
- Niculae C-M, et al. The 12th Edition of the Scientific Days of the National Institute for Infectious Diseases “Prof. Dr. Matei Bals” and the 12th National Infectious Diseases Conference. 99. Neguț AC, Săndulescu O, Streinu-Cercel A, et al. Influence of bacteriophages on sessile Gram-positive and Gramnegative bacteria. . in BMC Infectious diseases. 2016. BioMed Central.
- Woo J, Ahn JJAOM. Assessment of synergistic combination potential of probiotic and bacteriophage against antibiotic-resistant Staphylococcus aureus exposed to simulated intestinal conditions. 2014;196(10):719–727.
- Morris J, et al. Evaluation of bacteriophage anti-biofilm activity for potential control of orthopedic implant-related infections caused by Staphylococcus aureus. 2019;20(1):16–24.
- Fu W, et al. Bacteriophage cocktail for the prevention of biofilm formation by Pseudomonas aeruginosa on catheters in an in vitro model system. 2010;54(1):397–404.
- Olszak T, et al. In vitro and in vivo antibacterial activity of environmental bacteriophages against Pseudomonas aeruginosa strains from cystic fibrosis patients. 2015;99(14):6021–6033.
- Guang-Han O, et al. Experimental phage therapy for Burkholderia pseudomallei infection. 2016;11(7):e0158213.
- Chhibber S, Gupta P, Kaur SJBM. Bacteriophage as effective decolonising agent for elimination of MRSA from anterior nares of BALB/c mice. 2014;14(1):212.
- Abdulamir AS, et al. Novel approach of using a cocktail of designed bacteriophages against gut pathogenic E. colifor bacterial load biocontrol. 2014;13(1):39.
- Furusawa T, et al. Phage therapy is effective in a mouse model of bacterial equine keratitis. 2016;82(17):5332–5339.
- Yen M, Cairns LS, Camilli AJNC. A cocktail of three virulent bacteriophages prevents Vibrio cholerae infection in animal models. 2017;8(1):1–7.
- Augustine J, Gopalakrishnan MV, Bhat SG. Application of ΦSP-1 and ΦSP-3 as a therapeutic strategy against Salmonella Enteritidis infection using Caenorhabditis elegans as model organism. J FEMS Microbio Letters. 2014;356(1):113–117.
- Vieira A, et al. Phage therapy to control multidrug-resistant Pseudomonas aeruginosa skin infections: in vitro and ex vivo experiments. 2012;31(11):3241–3249.
- Kumari S, Harjai K, Chhibber Sjtjoiidc. Topical treatment of Klebsiella pneumoniae B5055 induced burn wound infection in mice using natural products. 2010;4(6):367–377.
- Wei C, et al. Developing a bacteriophage cocktail for biocontrol of potato bacterial wilt. Virol Sin. 2017;32(6):476–484. .
- Kocharunchitt C, Ross T, McNeil DL. Use of bacteriophages as biocontrol agents to control Salmonella associated with seed sprouts. Int J Food Microbiol. 2009;128(3):453–459.
- Elhalag K, et al. Potential use of soilborne lytic Podoviridae phage as a biocontrol agent against Ralstonia solanacearum. J Basic Microbiol. 2018;58(8):658–669. .
- Soleimani-Delfan A, et al. Isolation of Dickeya dadantii strains from potato disease and biocontrol by their bacteriophages. 2015;46(3):791–797.
- Tan D, et al. Vibriophages differentially influence biofilm formation by Vibrio anguillarum strains. Appl Environ Microbiol. 2015;81(13):4489–4497.
- Khairnar K, et al. Novel bacteriophage therapy for controlling metallo-beta-lactamase producing Pseudomonas aeruginosainfection in Catfish. BMC Vet Res. 2013;9(1):264. .
- Christiansen RH, et al. Effect of bacteriophages on the growth of flavobacterium psychrophilum and development of phage-resistant strains. Microb Ecol. 2016;71(4):845–859.
- Stalin N, Srinivasan P. Characterization of Vibrio parahaemolyticus and its specific phage from shrimp pond in Palk Strait, South East coast of India. Biologicals. 2016;44(6):526–533.
- Kittler S, et al. Effect of bacteriophage application on Campylobacter jejuni loads in commercial broiler flocks. 2013;79(23):7525–7533.
- Lau GL, Sieo CC, Tan WS, et al. Characteristics of a phage effective for colibacillosis control in poultry. J Sci Food Agric. 2012;92(13):2657–2663. .
- Belgini DRB, et al. Culturable bacterial diversity from a feed water of a reverse osmosis system, evaluation of biofilm formation and biocontrol using phages. World J Microbiol Biotechnol. 2014;30(10):2689–2700. .
- Maal KB, et al. Isolation and identification of two novel Escherichia Coli bacteriophages and their application in wastewater treatment and coliform’s phage therapy. 2015;8:3. J Jundishapur j microbio.
- Bull JJ, Levin BR, DeRouin T, et al. Dynamics of success and failure in phage and antibiotic therapy in experimental infections. BMC Microbiol. 2002;2(1):35.