ABSTRACT
Campylobacter jejuni is a predominant cause of gastroenteritis in humans but rather harmless in chickens. The basis of this difference is unknown. We investigated the effect of the chicken immune defense on the behavior of C. jejuni using glucocorticoid (GC)-treated and mock-treated 17-day old Ross 308 chicken bearing in mind that GCs have immunosuppressive effects and dampen the innate immune response. The effect of GC administration on the behavior of C. jejuni was compared with that on infection with Salmonella Enteritidis to address possible microbe-associated differences. Our results revealed that GC treatment fastened the intestinal colonization of C. jejuni (p < 0.001) and enhanced its dissemination to the liver (p = 0.007). The effect of GC on intestinal colonization of S. Enteritidis was less pronounced (p = 0.033) but GC did speed up the spread of this pathogen to the liver (p < 0.001). Cytokine transcript analysis showed an up to 30-fold reduction in baseline levels of IL-8 mRNA in the cecal (but not spleen) tissue at Day 1 after GC treatment (p < 0.005). Challenge with C. jejuni strongly increased intestinal IL-8, IL-6, and iNOS transcript levels in the non-GC treated animals but not in the GC-treated birds (P < 0.005). In vitro assays with chicken macrophages showed that GC dampened the TLR agonist- and C. jejuni induced-inflammatory gene transcription and production of nitric oxide (P < 0.005). Together, the results support the hypothesis that C. jejuni has the intrinsic ability to invade chicken tissue and that an effective innate immune response may limit its invasive behavior.
Introduction
The bacterial food-borne pathogen Campylobacter jejuni (C. jejuni) is estimated to cause about 100 million cases of diarrheal illness each year.Citation1,2 One major source of C. jejuni is contaminated chicken meat.Citation2 C. jejuni frequently colonizes the ceca of chicken at high concentrations (109 CFU/g cecal content) usually without or with very mild clinical manifestations.Citation3-7 The molecular basis of the different effects of C. jejuni in humans and chickens is still unknown. Factors that may contribute include a differential expression of bacterial virulence traits in the different hosts, a variable composition of the local microbiota, intrinsic differences in the intestinal mucosal architecture, and/or differences in immune defense between the species. Detailed analysis of the interaction of C. jejuni with chicken cecal tissue has yielded variable results ranging from a lack of C. jejuni penetration of the intestinal mucus layer to C. jejuni invasion of chicken tissue with signs of a local inflammatory response.Citation6-10
One important step in the mucosal defense against bacterial pathogens is the early recognition of microbial products by the innate immune system. One class of host receptors that signals the presence of bacteria is the Toll-like receptor (TLR) family. Activation of members of this receptor family by bacterial ligands initiates downstream signaling events that result in nuclear translocation of transcription factors (such as NF-κB) that regulate the expression of pro- or anti-inflammatory genes. Comparative analysis of the human and chicken TLR repertoire revealed several differences between the mammalian and avian species (for a review see refs. 11,12). Chicken-specific TLR characteristics include a broader subset of TLR1/2 receptors,Citation13 the absence of a TLR4/MyD88-independent signaling pathway,Citation14 the presence of a protease-activated TLR15,Citation16 and the presence of TLR21 as a functional ortholog of human TLR9.Citation16,17 C. jejuni can activate both human and chicken TLRs,Citation18 but the contribution of TLRs to the chicken defense against C. jejuni remains to be determined.
One major class of regulators of mammalian biological systems including the immune system, is the adrenal-derived glucocorticoids. These steroid hormones bind to the cytosolic glucocorticoid (GC) receptor. The formed complex translocates to the nucleus and binds to response elements in the promoter regions of GC-responsive genes.Citation19,20 GCs also influence transcription factors that induce potent anti-inflammatory activity.Citation19,21,22 GCs are always detectable in serum, but their concentration strongly varies with the environmental stress encountered by the host. The hypothalamus-pituitary-adrenal axis responsible for the stress-related production of GC in mammals is also functional in chicken.Citation23,24 On poultry farms, environmental stress influences serum corticosterone levels and affects the susceptibility of chicken to infectious diseases,Citation25,26 possibly through its dampening effect on the immune system.
In the present study, we applied GC-induced immunosuppression to investigate the contribution of the chicken immune defense to the apparent commensal behavior of C. jejuni in chicken. GC-treated and control chickens were challenged with C. jejuni or (as control) with Salmonella enterica serovar Enteritidis (S. Enteritidis). Bacterial colonization, systemic dissemination, and tissue expression of pro-inflammatory genes were followed in time. In addition, the effect of GCs on C. jejuni-induced chicken innate immune (TLR) activation was assessed in cultured chicken cells. Our results demonstrate that bacterial challenge of GC treated chicken results in a poor inflammatory response and a more rapid intestinal colonization and dissemination of C. jejuni.
Results
Effect of glucocorticoids on the colonization and dissemination of C. jejuni in chicken
To investigate the reason for the behavior of C. jejuni in chicken, we first investigated the effect of GC administration on the intestinal colonization and dissemination of C. jejuni. Hereto, different groups of SPF chicken were injected with either the glucocorticoid Depo-Medrol (groups 1 and 3) or PBS (groups 2 and 4). After 24 h, groups 3 and 4 were challenged orally with 105 CFU of C. jejuni strain 81116. Groups 1 and 2 served as non-challenged controls. The following days, at least 5 chicken of each group were sacrificed to determine the number of C. jejuni in the ceca and liver, and to isolate tissue RNA for gene expression analysis.
Enumeration of C. jejuni in the collected cecal contents demonstrated significantly higher loads of Campylobacter in the ceca of the GC-treated group (group 3) compared to those of the PBS-injected group (group 4) (β = 0.56, 95%CI 0.26–0.86, p < 0.001) (). In the GC-treated animals, the number of C. jejuni had geometric mean values of 3.0 × 109 CFU/g content at Day 2 to 4.5 × 109 CFU/g content at Day 4 after challenge. In the control animals, C. jejuni values reached mean levels of 1.0 × 103 and 1.5 × 106 CFU/g at Days 2 and 3 respectively, rising to 7.1 × 107CFU at Day 4. Bacterial culture of liver tissue demonstrated significantly higher numbers of C. jejuni in the liver of the GC-treated group compared to the control group (β = 0.46, 95%CI 0.13–0.79, p = 0.007). At Day 2 and Day 3 after challenge, the number of C. jejuni in the liver reached mean values of 2.2 × 103 CFU/g and 4.1 × 103 CFU/g, respectively. At these time points, C. jejuni was still virtually absent in the liver of the control animals (1.1 CFU/g tissue at Day 2 and 16.7 CFU/g tissue at Day 3) ().
Figure 1. C. jejuni and S. Enteritidis colonization kinetics in GC-treated and control chickens. Chicken were injected with GC (closed blocks) or PBS (open circles) and 24 h later challenged orally with 105 CFU of C. jejuni or S. Enteritidis. At Day 1–4 post-challenge, C. jejuni (panel A) and S. Enteritidis (panel B) colonization of the ceca and the liver was estimated by CFU counting. Data are plotted as CFU per gram of cecal content or liver tissue for each chicken and expressed as the geometric mean (horizontal bars) of CFU per group of chicken. Statistical analysis of differences between treated and non-treated chickens were calculated with a gamma generalized linear model and gave the following values: Campylobacter in the ceca: β = 0.56, 95%CI 0.26–0.86, p < 0.001; Campylobacter in the liver: β = 0.46, 95%CI 0.13–0.79, p = 0.007; Salmonella in the ceca: β = 0.10, 95%CI 0.01–0.19, p = 0.033; Salmonella in the liver: β = 0.75, 95%CI 0.47–1.02, p < 0.001.
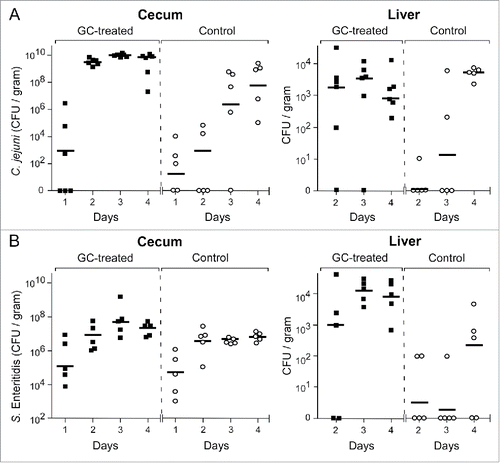
Experiments with chicken challenged with S. Enteritidis strain CVI-1 instead of C. jejuni revealed a slightly faster intestinal colonization (β = 0.10, 95%CI 0.01–0.19, p = 0.033) () and much faster bacterial spread to the liver (β = 0.75, 95%CI 0.47–1.02, p < 0.001) () for the GC-treated group (group 5) compared to the control group (group 6).
Inflammatory gene expression in chicken challenged with C. jejuni
To learn more about the mechanism(s) contributing to the more rapid colonization and dissemination of C. jejuni after GC administration, we first determined the effect of the C. jejuni challenge on the expression of inflammatory genes for the non-GC treated animals. RT-qPCR analysis on mRNA derived from cecal tissue at Day 1 after challenge with C. jejuni (group 4) showed a 50–100 fold up-regulation of IL-6, IL-8, and inducible nitric oxide synthase (iNOS) transcripts when compared to transcript levels in the non-challenged control group (group 2) (). At Day 4 after bacterial challenge, the difference in cecal IL-6, IL-8, IL-1β and iNOS transcripts was less pronounced (). In spleen tissue, the challenge with C. jejuni caused an increase in IL-6 and IL-8 transcripts at Day 1 and an additional increase in IL-1β mRNA at Day 4. The bacterial challenge did not change iNOS and IFNβ transcript levels ().
Figure 2. Effect of C. jejuni and S. Enteritidis colonization on cytokine mRNA levels. Transcript levels for the indicated cytokines were determined in cecal mucosa and spleen tissue isolated from individual birds at Day 1 and 4 after challenge with C. jejuni (panels A and B) or S. Enteritidis (panels C and D). RT-qPCR results were expressed as fold difference between the average mRNA levels in the indicated tissues of challenged chicken compared to (PBS-injected) control birds. Significant differences in ΔmRNA values were analyzed using log transformed data as described in Materials and Methods. Significant differences are indicated: **P < 0.005; *P < 0.05.
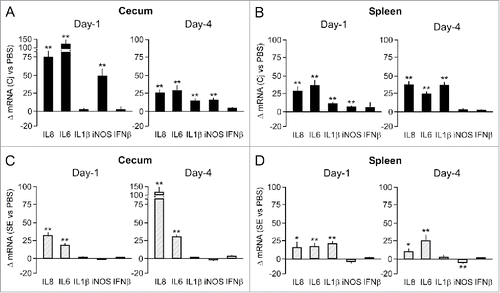
To determine the microbe specificity of the host response, we performed similar transcript analysis after challenge with S. Enteritidis. This pathogen induced an increase in cecal IL-8 and IL-6 transcript levels at Day-1 and Day-4. Transcript levels of iNOS did not differ between the infected and non-infected groups of animals (). This result clearly differed from the cecal response upon challenge with C. jejuni (cf. ). Like C. jejuni, infection with S. Enteritidis variably increased IL-8, IL-6 and IL-1β transcript levels in spleen tissue (). Neither C. jejuni nor S. Enteritidis significantly increased iNOS transcript levels in the spleen (). Overall, our results indicate that colonization of chicken with C. jejuni induces a robust host response and that C. jejuni and S. Enteritidis elicit bacteria- and tissue-specific cytokine responses.
Glucocorticoid-induced downregulation of pro-inflammatory gene expression
Transcript analysis on the same panel of inflammatory genes at Day 1 after administration of GC (i.e. without additional bacterial challenge)(group 1) revealed a 30-fold reduction of cecal baseline IL-8 mRNA levels compared to those in the PBS-injected control group (). At Day 4, only a minor difference in the cytokine mRNA levels between both groups was measured (). RT-qPCR assays on spleen tissue revealed no significant differences in baseline transcripts between the GC-treated and control group during the period of analysis (). The transient downregulation of baseline cytokine transcript levels in the ceca may reflect the suppression of the mucosal immune response elicited by the commensal bacterial flora. Notably, comparison of cytokine transcript levels between PBS-injected and non-injected chickens revealed no differences (data not shown), indicating that the acute stress that may be associated with the handling of the animals did not cause changes in the expression of the tested genes.
Figure 3. Effect of GC treatment on inflammatory gene expression in cecal mucosa and spleen tissue. Transcript levels of the indicated genes at Day 1 and 4 after injection of chicken with GC or PBS were determined by real-time RT-qPCR. Results are expressed as the mean ± SEM fold difference in tissue mRNA levels in the GC-treated vs. control animals. Significant differences in ΔmRNA values were analyzed using log transformed data as described in Materials and Methods. Significant differences are indicated: **P < 0.005; *P < 0.05.
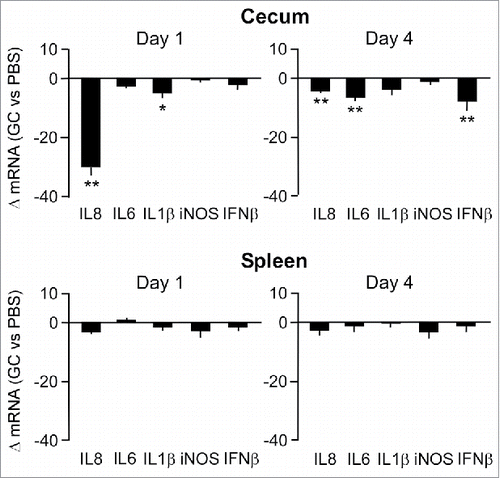
Effect of glucocorticoids on C. jejuni induced inflammatory gene transcripts
Next, we determined cytokine transcript levels for the complex combination of GC treatment plus bacterial challenge. Comparison of transcript levels in tissues from the GC-treated, C. jejuni challenged birds (group 3) with those in the PBS-injected, non-challenged animals (group 2) demonstrated significantly reduced cecal mRNA levels for IL-6, IL-8, and IL-1β at Day-1 post challenge. In spleen tissue, the effect was limited to a minor increase in IL-6 mRNA in the GC-treated, C. jejuni challenged group (). At Day 4 post-challenge, the reduced cytokine transcript levels in cecal tissue of the GC-treated and challenged animals had returned to baseline levels. At this point in time, a moderate increase in multiple pro-inflammatory gene transcripts was measured in spleen tissue ().
Figure 4. Effect of the combination of GC treatment plus challenge with C. jejuni or S. Enteritidis on inflammatory gene expression. Chicken were injected with GC or PBS and after 24 h challenged with C. jejuni (panels A and C) or S. Enteritidis (panels D and E). Real-time RT-qPCR was performed on mRNA isolated from cecal mucosa and spleen tissue collected at Days 1 and 4 post-challenge. Results are expressed as the mean ± SEM fold difference in mRNA levels in GC-treated, treated and challenged chicken versus PBS-injected and non-challenged animals (panels A and D), PBS-injected and C. jejuni challenged chicken (panel B), or GC-treated and non-challenged chicken (panels C and E). Significant differences in ΔmRNA values were analyzed using log transformed data as described in Materials and Methods. Significant differences are indicated: **P < 0.005; *P < 0.05.
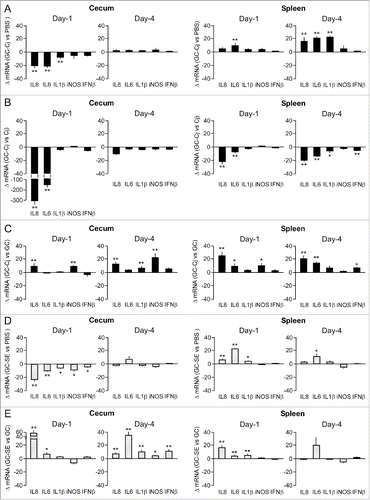
To more specifically determine the strong dampening effect of the GC treatment on the C. jejuni-induced tissue response, we compared gene transcript levels in the GC-treated, C. jejuni challenged animals (group 3) with those in the non-GC treated, C. jejuni-challenged chickens (group 4). This demonstrated that GC treatment reduced the C. jejuni-induced IL-8 and IL-6 response by >150-fold at Day 1 post-challenge (). On Day 4, the strong immunosuppressive effect had virtually disappeared. In spleen tissue, GC treatment reduced several of the measured C. jejuni-induced cytokine transcripts by a factor 5 to 20 on both Day 1 and Day 4 ().
To learn whether C. jejuni was still able to induce a cytokine response in the GC-treated birds, we compared the gene transcript levels in the cecal tissue of the GC-treated, C. jejuni challenged animals (group 3) with those of the GC-treated, non-challenged chickens (group 1). This showed only a small increase in the cecal IL-8 and iNOS mRNA levels after C. jejuni challenge (). This indicates that GC treatment largely prevents the robust C. jejuni-induced inflammatory response that is observed after C. jejuni infection of non GC-treated chickens (). This effect may contribute to the observed more rapid colonization of the ceca and liver in these animals ().
Effect of glucocorticoids on S. enteritidis induced inflammatory gene transcripts
Comparative analysis of gene transcripts in tissues derived from GC-treated chicken challenged with S. Enteritidis (group 5) with those from non-infected control chickens (group 2) indicated that the GC treatment inhibited the Salmonella-induced increase in intestinal IL-8 and IL-6 response at both Day-1 and Day-4 (compare ). In spleen tissue the changes in IL-8, IL-6 and IL-1β transcript levels were also less pronounced than in the non-GC treated, S. Enteritidis challenged animals (cf. ). When the transcript data of the GC-treated, non-challenged animals were used as a reference, moderate increases in mainly IL-8 (Day-1) and IL-6 transcript levels (Day-4) were measured for both intestinal and spleen tissue samples (). Overall, these data point to the presence of a GC treatment-induced attenuation of the host response toward S. Enteritidis, as was seen for C. jejuni.
GC modulation of the TLR response
In search for a potential explanation of the apparent attenuated inflammatory response in the GC-treated chickens, we investigated the expression of inflammatory genes and the production of nitric oxide (NO) in 2 different chicken macrophages cell lines (MQ-NCSU and HD11). Hereto, cells were exposed to the glucocorticoid dexamethasone (10−6 M) or solvent solution for 17 h prior to the addition of defined bacterial TLR agonists. RT-qPCR analysis on mRNA isolated from the non GC-treated macrophages demonstrated a strong induction (150–200 fold) of iNOS transcript after exposure of the cells to LPS, flagellin, and live C. jejuni strain 81116 (). GC pretreatment of the cells fully abrogated this response in both cell lines. A similar strong reduction was measured for the IL-8 and IL-6 response in MQ-NCSU cells and for the IL-6, IL-1β and IFNβ transcripts in HD11 cells ().
Figure 5. Effect of GC-treatment gene TLR- and C. jejuni-induced gene transcription in chicken macrophages. (A) MQ-NCSU and HD11 cells pre-incubated (17 h) with or without dexamethasone were stimulated with LPS (100 ng/ml) or flagellin (FliC, 1 µg/ml) for 16 h or with live C. jejuni (2 × 105) for 8 h. Then, real-time RT-qPCR was performed on mRNA isolated from the cells to measure differences in iNOS mRNA levels between the Dex-treated and control cells. (B) Fold difference in the indicated inflammatory gene transcript levels in the GC-treated and non-GC treated cells after stimulation with LPS (100 ng/ml, 16 h). All results are expressed as the mean ± SEM fold difference in the transcript levels between the Dex-treated ands control cells (n = 3). For each response significant differences between Dex-treated and control cells are indicated: **P < 0.001; *P < 0.05.
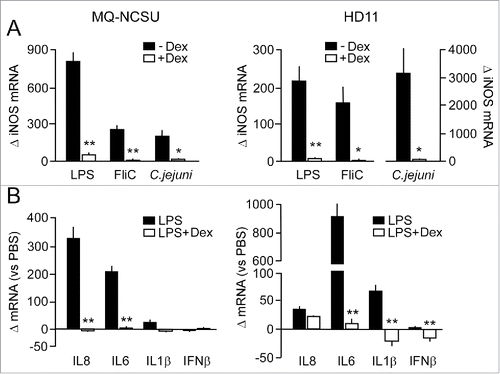
To ensure that the observed changes in inflammatory gene transcription translated to the protein level, we measured the production of cellular nitric oxide (NO) under the different conditions. This revealed that both bacterial LPS and flagellin (FliC) induced a dose-dependent increase in NO production in the chicken macrophages (), in line with the observed induction of iNOS mRNA (). Again, GC pretreatment (10−6 M, 17 h) of the cells strongly reduced these responses (). The inhibitory effect required preincubation of the cells with GC. The effect was not observed when GC and the TLR agonists were added simultaneously (data not shown). Together, these results indicate that GC strongly inhibits both the TLR agonist- and bacteria-induced stimulation of the inflammatory response in chicken macrophages. This is in line with the observed attenuated in vivo response and may contribute to the observed invasiveness of C. jejuni in GC-treated chickens.
Figure 6. Effect of GC treatment on LPS or flagellin-induced production of nitric oxide in chicken macrophages. NCSU and HD11 chicken macrophages were incubated with dexamethasone (Dex, 10−6 M) for 17 h and then stimulated with the indicated concentrations of LPS (A) or flagellin (FliC) (B). After 24 h of stimulation, NO production was measured using the Griess assay. Results are the mean ± SEM of 6 (A) and 4 (B) experiments. Significant differences in ligand-induced NO production between Dex-treated and control cells are indicated: **P < 0.005; *P < 0.05.
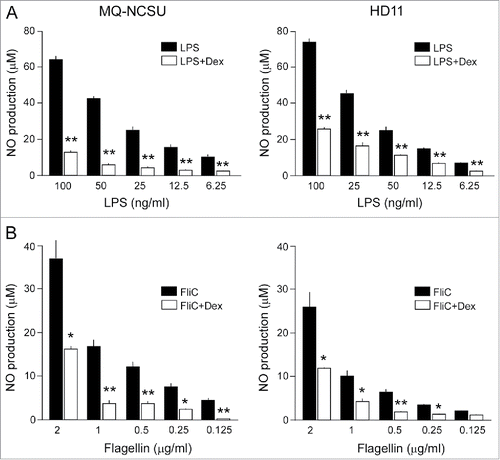
Discussion
In the present study, we investigated the role of the host immune defense in the behavior of C. jejuni in chickens. We provide evidence that GC treatment results in more rapid intestinal colonization and dissemination of C. jejuni to the liver in conjunction with a reduced pro-inflammatory gene expression at the infection niche. Stimulation of macrophages with different TLR ligands demonstrated strong inhibition of the chicken TLR response for GC-treated cells confirming that GC weakens at least one component of the innate host defense. Our results support the concept that C. jejuni has the intrinsic property to spread in chicken but that the natural host defense may limit C. jejuni invasion and dissemination to distant organs.
The rationale for our study was the still unexplained host specificity of C. jejuni infection. C. jejuni is the leading cause of bacterial enterocolitis worldwide but relatively rarely induces clinical manifestations in the chicken.Citation8,10 Potential reasons for this difference are numerous and may range from a different expression of bacterial virulence traits or mucosal receptors to the existence of a different microbiota in the human and chicken host. Alternatively, it can be imagined that chickens have a more effective host defense against C. jejuni, although this appears to vary between chicken breeds.Citation7,27 We investigated the influence of the chicken immune response on the colonization and dissemination of C. jejuni in chicken by administering GC to the animals prior to C. jejuni challenge. GC has immunosuppressive effects, also in the chicken.Citation28,29 Our results indicate that administration of GC to chicken indeed results in a downregulation of baseline transcript levels of distinct inflammatory genes in the cecal tissue (). This result likely reflects the GC-induced dampening of the innate immune response.
Challenge of Ross 308 chicken with C. jejuni strain 81116 resulted in a more rapid colonization of the ceca and bacterial spread to the liver in the GC-treated animals (). The faster colonization of C. jejuni after GC treatment was unexpected. This effect was less pronounced for S. Enteritidis and has not been observed with S. Typhimurium.Citation30 One possible explanation for the more rapid colonization with C. jejuni may be that GC treatment induces C. jejuni favorable alterations in the intestinal microenvironment by influencing e.g. the composition of the microbiota, the production or composition of the mucus, or the status of the local antimicrobial defense.Citation6,31 Alternatively, the lack of a potent innate immune response may contribute to the rapid bacterial expansion after GC treatment.
The rapid dissemination of C. jejuni to the liver in the GC-treated birds indicates that the bacterium has the intrinsic ability to spread to distant organs. Dissemination of C. jejuni to the blood and liver of chickens occurs infrequently and seems to vary between bacterial strains and chicken lines.Citation5,32,33 Multiple mechanisms may contribute to the dissemination in the GC-treated animals. One hypothesis is that the more rapid cecal colonization (3.109 CFU at Day-2 after challenge) simply results in a more rapid bacterial dissemination. Another is that GC treatment causes a general increased translocation of bacteria across the intestinal barrier. We considered these explanations as less likely as GC treatment has been reported to support rather than weaken intestinal barrier function.Citation34,35 We favored the scenario that the GC-induced dampening of the local innate immune response facilitates the bacterial spread to distant organs.
Analysis of the expression of inflammatory genes in the cecal tissue after challenge with C. jejuni revealed a strong increase IL-6, IL-8 and iNOS transcripts. This pro-inflammatory signature in the non-GC treated animals indicates that C. jejuni is sensed by the chicken immune system and elicits a potent local immune response as previously noted.Citation7-10,36-38 The measured gene expression levels induced by C. jejuni were relatively high but this may obviously vary between chicken lines and depend on the composition of the microbiota which likely determines the baseline cytokine transcript levels. GC treatment reduced the baseline transcript levels in the cecal tissue and these levels barely increased after challenge with C. jejuni (). This lack of response is likely caused by the immunosuppressive effect of GC on the mucosal cells but perhaps also by a reduced influx of inflammatory cells into the mucosal tissues. Both instances result in a weakened host defense and thus may contribute to the more invasive behavior of C. jejuni.
To ascertain that GC administration can limit the innate immune response in chicken cells, we tested the effect of GC on chicken macrophages in vitro. Avian cells respond to C. jejuni with increased transcript levels of cytokines and chemokines and the production of nitric oxide.Citation18,39-41 This response may involve the activation of different types of pathogen recognition receptors. One major molecular mechanism via which GC may limit the mucosal host defense is the inhibition of the TLR response.Citation42,43 TLRs are key sensors of environmental danger signals including microbial products and are major drivers of the innate immune response. The chicken TLR repertoire is well established and has been shown to respond to C. jejuni.Citation18 Our finding that GC administration severely dampens chicken macrophage gene transcription and nitric oxide production in response to the TLR agonists LPS (TLR4) and flagellin (TLR5) as well as after exposure to C. jejuni () shows that the TLR pathway in chickens is responsive to GC. These results support the scenario that GC administration contributes to the invasive behavior of C. jejuni at least partially by suppression of the local innate immune response. To some extent, this situation may resemble observations in mice that show that animals with innate immune deficiencies become prone to C. jejuni infection.Citation44-46
Overall, our results for the first time demonstrate that GC treatment of chickens dampens the intestinal immune defense and causes a more rapid colonization and dissemination of C. jejuni in chicken. The data imply that C. jejuni has the intrinsic ability to invade chicken tissue and that the innate defense is important to limit this invasive behavior.
Materials and methods
Bacterial culture
C. jejuni strain 81116 (NTCT 11828)Citation47,48 was grown on Blood agar base II medium (Oxoid) containing 5% horse blood lysed with 0.5% saponin at 42°C under microaerophilic (5% O2, 10% CO2, 85% N2) conditions. Salmonella enterica serovar Enteritidis (S. Enteritidis, nalidixic acid resistant) strain CVI-1 was grown on Luria-Bertani (LB) plates (Biotrading) at 37°C. Bacteria from cloacal swabs and serial dilutions of tissue homogenates were grown on Campylobacter selective blood-free agar plates with CCDA-selective (Oxoid SR0155E) supplement (C. jejuni) or on Brilliant Green Agar supplemented with 100 µg/ml of nalidixic acid (S. Enteritidis) to suppress growth of resident flora. For use in challenge experiments, C. jejuni and S. Enteritidis were grown in Heart Infusion broth and Brain Heart Infusion broth (Biotrading) with nalidixic acid (Sigma-Aldrich) respectively, collected by centrifugation (4,000 × g, 10 min), and resuspended in PBS. Bacterial suspensions (105 CFU in 0.25 ml) were administered orally to the chicken using a 1 ml syringe that was carefully placed deeply into the mouth (7 cm).
Cell culture
The chicken macrophage HD1149 and MQ-NCSUCitation50 cell lines were propagated in 25 cm2 tissue culture flasks (Corning) containing 5 ml of Dulbecco's modified Eagle medium (Life Technologies) supplemented with 5% fetal bovine serum (FBS) at 37°C and 10% CO2. For use in cell stimulation assays, cells were seeded onto 12- or 24-well plates (105 cells/well) in 1 ml of DMEM plus 5% FBS per well.
Chicken experiments
Fertilized SPF (Campylobacter and Salmonella free) chicken eggs (Ross 308) were kept at 38°C and 65–75% relative humidity in a forced air chicken egg incubator. After hatch, the chickens were divided into 6 groups (1–6) of 27 birds and housed in pens with ad libitum access to water and feed. Bacterial cultures of cloaca swabs taken at Day 1 after hatch confirmed the Campylobacter and Salmonella negative status of the animals. At Day 17 after hatch groups 1, 3, and 5 received the synthetic glucocorticoid methylprednisolone (Depo-Medrol®, intramuscular, 10 mg/kg body weight; Pfizer), while groups 2, 4, and 6 were injected with phosphate buffered saline (PBS). At 24 h after administration, groups 3 and 4 were challenged orally with 105 CFU of C. jejuni strain 81116, while group 5 and 6 were challenged with the equivalent number of S. Enteritidis. Groups 1 and 2 served as non-challenged controls.
At Days 1, 2, 3, and 4 after bacterial challenge, at least 5 birds from each group were sacrificed by cervical dislocation by professional staff to be able to determine the number of C. jejuni or S. Enteritidis in the cecal contents and liver, and to collect tissues for transcript analysis (see below). For bacterial enumeration, serial dilutions of 1 g of cecal content or 1 g of liver tissue homogenized in 3 ml of peptone water, were grown as described above. The number of colonies (CFU) was counted after 24–48 h of incubation. Collected tissues were snap-frozen in liquid nitrogen and stored at −80°C until further analysis. All chicken were cared for in accordance with accepted procedures of the Dutch law of animal welfare and all animal experiments were approved by the Ethics Committee of the Central Veterinary Institute of Wageningen University, Lelystad, the Netherlands.
A gamma generalized linear model with a log link function (as the CFU data were continuous, positive, right-skewed and with both constant variance and normally distributed residuals on the log scale) was used to indicate statistically significant differences between treated and non-treated chicks. This method allows assessing the overall effect of GC treatment on Campylobacter and Salmonella CFUs in the chicks’ ceca and livers over the entire course of bacterial colonization, while accounting for the day of sampling.
In vitro treatment of cultured chicken cells
To measure the effects of GC on cultured chicken cells, dexamethasone (Sigma) or the equivalent amount of solvent (ethanol, final concentration <0.5%) was added at 12 h after seeding of the cells onto the 12- or 24-well plates (low cell density). Seventeen hours later, cells were stimulated with the indicated amounts of purified LPS, flagellin, or bacteria. After the indicated incubation periods (37°C, 10% CO2), cell culture supernatants were collected for measurement of nitric oxide (NO). The cells were treated with RNA-Bee™ (Bio-connect) to extract RNA for transcript analysis (see below). Purified LPS and Salmonella flagellin (FliC) were isolated as previously described.Citation14,51
RNA isolation from tissue and cells
RNA was isolated from the collected tissues by placing approximately 50 mg of tissue in a LYSING matrix tube (MP Biomedical GmbH) containing 1 ml of RNA-BEE™ on ice. Cells were disrupted in MagNA lyser instrument (Roche) (6,500 × g, 50 sec, 20°C). Total RNA was extracted from the lysate using the RNA-Bee™ isolation kit according to the instruction of the manufacturer. Contaminating DNA was removed by treatment of the RNA samples with DNase (1 U/µg of RNA, Fermentas). The quantity and purity of the isolated RNA was verified using a NanoDrop ND-1000 spectrophotometer. For isolation of RNA from cultured cells, 105 cells were collected in 250 µl of RNA-Bee™ and directly subjected to the RNA-Bee extraction method described above. Purified RNA was stored in 25 µl of RNase-free water and stored at -80°C until further analysis.
Quantitative real-time RT-PCR analysis
Quantitative real-time PCR on isolated RNA was routinely performed using the Reverse Transcriptase RT-qPCR Master Mix kit (Eurogentec). The reaction was performed in a Roche LightCycler®480 using 50 ng of DNase I-treated RNA samples as template and the primer sets and probes listed in . Probes for IL-8 (CXCL8-CXCLi2), IL-6, IL-1β and IFNβ were labeled with the fluorescent reporter dye 5-carboxyfluorescein (FAM) at the 5′-end and with the quencher N, N, N, N'- tetramethyl-6-carboxyrhodamine (TAMRA) at the 3′-end (Eurogentec). Inducible isoform of nitric oxide synthase (iNOS) transcripts were measured using the one-step RT-qPCR Master Mix Plus SYBR Green1 kit (Eurogentec). The following reaction conditions were used: Reverse Transcription step at 48°C for 30 min followed by incubation for 10 min at 95°C, 40 cycles of 15 s at 95°C, and 60 s at 60°C. Each sample was run in duplicate. Non-reverse transcriptase-treated samples, a template free sample, and a nuclease free water sample served as controls. Transcript levels were normalized to those for the chicken internal control genes glyceraldehyde-3-phosphate dehydrogenase (chGAPDH) or glucose-6-phosphate dehydrogenase (chG6PDH, HD11 cells only). For each gene, results were expressed as fold change in mRNA level compared to the PBS-injected, GC-injected or C. jejuni challenged control group according to Schmittgen & LivakCitation52 using the formula: (1) Δ Ct target gene - Δ Ct GAPDH (or G6PDH) for each sample, (2) Δ Ct target gene treated - Δ Ct target gene control. The fold change for each gene transcript was determined using: Fold change = 2-Δ(Δ Ct gene treated -Δ C gene controll). To calculate the relative expression levels for each of the bird groups, the transcript levels in samples of individual birds were compared with the mean value of the group of control birds, yielding a mean ± SEM value for the treated groups. The SEM values thus represent the variation in fold difference between individual chicken. The expression of the internal control genes was not influenced by the GC treatment.
Table 1. Primers and probes used in this study.
Statistical analysis on all RT-qPCR results was performed on log transformed data the GraphPad Prism 6 multiple t test corrected for multiple comparison using the Holm-Šidák method, with α = 5.000%
Nitric oxide assay
For measurement of nitric oxide (NO) production, the Griess assay was employed. In brief, cell supernatants collected after 17 h of incubation with the bacterial TLR agonist (or controls) were incubated (10 min, 20°C, in dark) with an equal volume (50 µl) of Griess reagent 1 (1% sulfanilamide, Sigma-Aldrich). Then, an equal volume of Griess reagent 2 (0.1% N-naphthyl ethylene diamine dihydrochloride (Sigma-Aldrich) in 2.5% phosphoric acid) was added. After an additional 5 min of incubation in the dark, absorbance was measured at 550 nm in a spectrophotometer. The amount of produced NO was calculated from a calibration curve established by serial dilution (1–100 µM) of sodium nitrite (NaNO2) in tissue culture medium. Data were analyzed using the GraphPad Prism 6 multiple t test. Values are expressed as the mean +/− SEM of at least 3 independent experiments.
Abbreviations
C. jejuni | = | Campylobacter jejuni |
chGAPDH | = | chicken glyceraldehyde-3-phosphate dehydrogenase |
chG6PDH | = | chicken glucose-6-phosphate dehydrogenase |
CFU | = | Colony forming units |
Dex | = | Dexamethasone |
DMEM | = | Dulbecco's modified Eagle medium |
FBS | = | Fetal bovine serum |
GC | = | Glucocorticoid |
IL | = | Interleukin |
iNOS | = | Inducible isoform of nitric oxide synthase |
LPS | = | Lipopolysaccharide |
NO | = | Nitric oxide |
PBS | = | Phosphate buffered saline |
RT-qPCR | = | Reverse transcription polymerase chain reaction |
S. Enteritidis | = | Salmonella enterica serovar Enteritidis |
SPF | = | Specific pathogen free |
TLR | = | Toll-like receptor. |
Disclosure of potential conflicts of interest
No potential conflicts of interest were disclosed.
Funding
The laboratory acknowledges the financial support for Campylobacter research from the Dutch Ministry of Economics, Agriculture and Innovation, the Danish Agency for Science, Technology and Innovation (CamVac grant number: 09-067131) and the European Union (CamCon grant number: 244547).
References
- Ruiz-Palacios GM. The health burden of Campylobacter infection and the impact of antimicrobial resistance: playing chicken. Clin Infect Dis 2007; 44:701-03; PMID:17278063; http://dx.doi.org/10.1086/509936
- Havelaar AH, van Pelt W, Ang CW, Wagenaar JA, van Putten JP, Gross U, Newell DG. Immunity to Campylobacter: its role in risk assessment and epidemiology. Crit Rev Microbiol 2009; 35:1-22; PMID:19514906; http://dx.doi.org/10.1080/10408410802636017
- Beery JT, Hugdahl MB, Doyle MP. Colonization of gastrointestinal tracts of chicks by Campylobacter jejuni. Appl Environ Microbiol 1998; 54:2365-70
- Newell DG, Fearnley C. Sources of Campylobacter colonization in broiler chickens. Appl Environ Microbiol 2003; 69:4343-51; PMID:12902214; http://dx.doi.org/10.1128/AEM.69.8.4343-4351.2003
- Van Deun K, Pasmans F, Ducatelle R, Flahou B, Vissenberg K, Martel A, Van den Broeck W, Van Immerseel F, Haesebrouck F. Colonization strategy of Campylobacter jejuni results in persistent infection of the chicken gut. Vet Microbiol 2008; 130:285-97; PMID:18187272; http://dx.doi.org/10.1016/j.vetmic.2007.11.027
- Hermans D, Pasmans F, Heyndrickx M, Van Immerseel F, Martel A, Van Deun K, Haesebrouck F. Tolerogenic mucosal immune response leads to persistent Campylobacter jejuni colonization in the chicken gut. Crit Rev Microbiol 2012; 38:17-29; PMID:21995731; http://dx.doi.org/10.3109/1040841X.2011.615298
- Humphrey S, Chaloner G, Kemmett K, Davidson N, Williams N, Kipar A, Humphrey T, Wigley P. Campylobacter jejuni is not merely a commensal in commercial broiler chickens and affects bird welfare. MBio 2014; 5:e01364-14; PMID:24987092; http://dx.doi.org/10.1128/mBio.01364-14
- Smith CK, Abuoun M, Cawthraw SA, Humphrey TJ, Rothwell L, Kaiser P, Barrow PA, Jones MA. Campylobacter colonization of the chicken induces a proinflammatory response in mucosal tissues. FEMS Immunol Med Microbiol 2008; 54:114-21; PMID:18647351; http://dx.doi.org/10.1111/j.1574-695X.2008.00458.x
- Shaughnessy RG, Meade KG, McGivney BA, Allan B, O'Farrelly C. Global gene expression analysis of chicken cecal response to Campylobacter jejuni. Vet Immunol Immunopathol 2011; 142:64-71; PMID:21605915; http://dx.doi.org/10.1016/j.vetimm.2011.04.010
- Awad WA, Molnár A, Aschenbach JR, Ghareeb K, Khayal B, Hess C, Liebhart D, Dublecz K, Hess M. Campylobacter infection in chickens modulates the intestinal epithelial barrier function. Innate Immun 2015; 21:151-60; PMID:24553586; http://dx.doi.org/10.1177/1753425914521648
- Cormican P, Lloyd AT, Downing T, Connell SJ, Bradley D, O'Farrelly C. The avian Toll-like receptor pathway–subtle differences amidst general conformity. Dev Comp Immunol 2009; 33:967-73; PMID:19539094; http://dx.doi.org/10.1016/j.dci.2009.04.001
- Keestra AM, de Zoete MR, Bouwman LI, Vaezirad MM, van Putten JP. Unique features of chicken Toll-like receptors. Dev Comp Immunol 2013; 41:316-23; PMID:23628643; http://dx.doi.org/10.1016/j.dci.2013.04.009
- Keestra AM, de Zoete MR, van Aubel RA, van Putten JP. The central leucine-rich repeat region of chicken TLR16 dictates unique ligand specificity and species-specific interaction with TLR2. J Immunol 2007; 178:7110-19; http://dx.doi.org/10.4049/jimmunol.178.11.7110
- Keestra AM, van Putten JP. Unique properties of the chicken TLR4/MD-2 complex: selective lipopolysaccharide activation of the MyD88-dependent pathway. J Immunol 2008; 181:4354-62; PMID:18768894; http://dx.doi.org/10.4049/jimmunol.181.6.4354
- de Zoete MR, Bouwman LI, Keestra AM, van Putten JP. Cleavage and activation of a Toll-like receptor by microbial proteases. Proc Natl Acad Sci U S A 2011; 108:4968-73; PMID:21383168; http://dx.doi.org/10.1073/pnas.1018135108
- Brownlie R, Zhu J, Allan B, Mutwiri GK, Babiuk LA, Potter A, Griebel P. Chicken TLR21 acts as a functional homologue to mammalian TLR9 in the recognition of CpG oligodeoxynucleotides. Mol Immunol 2009; 46:3163-70; PMID:19573927; http://dx.doi.org/10.1016/j.molimm.2009.06.002
- Keestra AM, de Zoete MR, Bouwman LI, van Putten JP. Chicken TLR21 is an innate CpG DNA receptor distinct from mammalian TLR9. J Immunol 2010; 185:460-67; PMID:20498358; http://dx.doi.org/10.4049/jimmunol.0901921
- de Zoete MR, Keestra AM, Roszczenko P, van Putten JP. Activation of human and chicken Toll-like receptors by Campylobacter spp. Infect Immun 2010; 78:1229-38; PMID:20038539; http://dx.doi.org/10.1128/IAI.00897-09
- Barnes PJ. Glucocorticosteroids: current and future directions. Br J Pharmacol 2011; 163:29-43; PMID:21198556; http://dx.doi.org/10.1111/j.1476-5381.2010.01199.x
- Kadmiel M, Cidlowski JA. Glucocorticoid receptor signaling in health and disease. Trends Pharmacol Sci 2013; 34:518-30; PMID:23953592; http://dx.doi.org/10.1016/j.tips.2013.07.003
- Ratman D, Vanden Berghe W, Dejager L, Libert C, Tavernier J, Beck IM, De Bosscher K. How glucocorticoid receptors modulate the activity of other transcription factors: a scope beyond tethering. Mol Cell Endocrinol 2013; 380:41-54; PMID:23267834; http://dx.doi.org/10.1016/j.mce.2012.12.014
- Gupte R, Muse GW, Chinenov Y, Adelman K, Rogatsky I. Glucocorticoid receptor represses proinflammatory genes at distinct steps of the transcription cycle. Proc Natl Acad Sci U S A 2013; 110:14616-21; PMID:23950223; http://dx.doi.org/10.1073/pnas.1309898110
- de Matos R. Adrenal steroid metabolism in birds: Anatomy, physiology, and clinical considerations. Vet Clin Exot Anim 2008; 11:35-57; http://dx.doi.org/10.1016/j.cvex.2007.09.006
- Kaiser P, Wu Z, Rothwell L, Fife M, Gibson M, Poh TY, Shini A, Bryden W, Shini S. Prospects for understanding immune-endocrine interactions in the chicken. Gen Comp Endocrinol 2009; 163:83-91; PMID:18957294; http://dx.doi.org/10.1016/j.ygcen.2008.09.013
- Quinteiro-Filho WM, Rodrigues MV, Ribeiro A, Ferraz-de-Paula V, Pinheiro ML, Sá LR, Ferreira AJ, Palermo-Neto J. Acute heat stress impairs performance parameters and induces mild intestinal enteritis in broiler chickens: role of acute hypothalamic-pituitary-adrenal axis activation. J Anim Sci 2012; 90:1986-94; PMID:22228037; http://dx.doi.org/10.2527/jas.2011-3949
- Gomes AV, Quinteiro-Filho WM, Ribeiro A, Ferraz-de-Paula V, Pinheiro ML, Baskeville E, Akamine AT, Astolfi-Ferreira CS, Ferreira AJ, Palermo-Neto J. Overcrowding stress decreases macrophage activity and increases Salmonella Enteritidis invasion in broiler chickens. Avian Pathol 2014; 43:82-90; PMID:24350836; http://dx.doi.org/10.1080/03079457.2013.874006
- Humphrey S, Lacharme-Lora L, Chaloner G, Gibbs K, Humphrey T, Williams N, Wigley P. Heterogeneity in the infection biology of Campylobacter jejuni isolates in three infection models reveals an invasive and virulent phenotype in a ST21 isolate from poultry. PLoS One 2015; 10:e0141182; PMID:26496441; http://dx.doi.org/10.1371/journal.pone.0141182
- Shini S, Kaiser P. Effects of stress, mimicked by administration of corticosterone in drinking water, on the expression of chicken cytokine and chemokine genes in lymphocytes. Stress 2009; 12:388-99; PMID:19006006; http://dx.doi.org/10.1080/10253890802526894
- Shini S, Shini A, Kaiser P. Cytokine and chemokine gene expression profiles in heterophils from chickens treated with corticosterone. Stress 2010; 13:185-94; PMID:19958164; http://dx.doi.org/10.3109/10253890903144639
- Corrier DE, Elissalde MH, Ziprin RL, DeLoach JR. 1991. Effect of immunosuppression with cyclophosphamide, cyclosporin, or dexamethasone on Salmonella colonization of broiler chicks. Avian Dis 1991; 35:40-50; PMID:2029260; http://dx.doi.org/10.2307/1591292
- Ivanov II, Littman DR. Modulation of immune homeostasis by commensal bacteria. Curr Opin Microbiol 2011; 14:106-14; PMID:21215684; http://dx.doi.org/10.1016/j.mib.2010.12.003
- Cox NA, Richardson LJ, Buhr RJ, Bailey JS, Wilson JL, Hiett KL. 2006. Detection of Campylobacter jejuni in various lymphoid organs of broiler breeder hens after oral or intravaginal inoculation. Poult Sci 2006; 85:1378-82; PMID:16903467; http://dx.doi.org/10.1093/ps/85.8.1378
- Richardson LJ, Cox NA, Buhr RJ, Harrison MA. Isolation of Campylobacter from circulating blood of commercial broilers. Avian Dis 2011; 55:375-78; PMID:22017033; http://dx.doi.org/10.1637/9613-121310-Reg.1
- Boivin MA, Ye D, Kennedy JC, Al-Sadi R, Shepela C, Ma TY. Mechanism of glucocorticoid regulation of the intestinal tight junction barrier. Am J Physiol Gastrointest Liver Physiol 2007; 292:G590-98; PMID:17068119; http://dx.doi.org/10.1152/ajpgi.00252.2006
- Fischer A, Gluth M, Weege F, Pape UF, Wiedenmann B, Baumgart DC, Theuring F. Glucocorticoids regulate barrier function and claudin expression in intestinal epithelial cells via MKP-1. Am J Physiol Gastrointest Liver Physiol 2014; 306:G218-28; PMID:24309183; http://dx.doi.org/10.1152/ajpgi.00095.2013
- Shaughnessy RG, Meade KG, Cahalane S, Allan B, Reiman C, Callanan JJ, O'Farrelly C. Innate immune gene expression differentiates the early avian intestinal response between Salmonella and Campylobacter. Vet Immunol Immunopathol 2009; 132:191-98; PMID:19632728; http://dx.doi.org/10.1016/j.vetimm.2009.06.007
- Meade KG, Narciandi F, Cahalane S, Reiman C, Allan B, O'Farrelly C. Comparative in vivo infection models yield insights on early host immune response to Campylobacter in chickens. Immunogenetics 2009; 61:101-10; PMID:19082824; http://dx.doi.org/10.1007/s00251-008-0346-7
- Li X, Swaggerty CL, Kogut MH, Chiang HI, Wang Y, Genovese KJ, He H, Zhou H. Gene expression profiling of the local cecal response of genetic chicken lines that differ in their susceptibility to Campylobacter jejuni colonization. PLoS One 2010; 5:e11827; PMID:20676366; http://dx.doi.org/10.1371/journal.pone.0011827
- Smith CK, Kaiser P, Rothwell L, Humphrey T, Barrow PA, Jones MA. Campylobacter jejuni-induced cytokine responses in avian cells. Infect Immun 2005; 73:2094-2100; PMID:15784550; http://dx.doi.org/10.1128/IAI.73.4.2094-2100.2005
- Li YP, Ingmer H, Madsen M, Bang DD. Cytokine responses in primary chicken embryo intestinal cells infected with Campylobacter jejuni strains of human and chicken origin and the expression of bacterial virulence-associated genes. BMC Microbiol 2008; 8:107; PMID:18588667; http://dx.doi.org/10.1186/1471-2180-8-107
- Larson CL, Shah DH, Dhillon AS, Call DR, Ahn S, Haldorson GJ, Davitt C, Konkel ME. Campylobacter jejuni invade chicken LMH cells inefficiently and stimulate differential expression of the chicken CXCLi1 and CXCLi2 cytokines. Microbiology 2008; 154:3835-47; PMID:19047751; http://dx.doi.org/10.1099/mic.0.2008/021279-0
- Moynagh PN. Toll-like receptor signalling pathways as key targets for mediating the anti-inflammatory and immunosuppressive effects of glucocorticoids. J Endocrinol 2003; 179:139-44; PMID:14596665; http://dx.doi.org/10.1677/joe.0.1790139
- Chinenov Y, Rogatsky I. Glucocorticoids and the innate immune system: crosstalk with the Toll-like receptor signaling network. Mol Cell Endocrinol 2007; 275:30-42; PMID:17576036; http://dx.doi.org/10.1016/j.mce.2007.04.014
- Mansfield LS, Bell JA, Wilson DL, Murphy AJ, Elsheikha HM, Rathinam VA, Fierro BR, Linz JE, Young VB. 2007. C57BL/6 and congenic interleukin-10-deficient mice can serve as models of Campylobacter jejuni colonization and enteritis. Infect Immun 2007; 75:1099-1115; PMID:17130251; http://dx.doi.org/10.1128/IAI.00833-06
- Bereswill S, Fischer A, Plickert R, Haag LM, Otto B, Kühl AA, Dasti JI, Zautner AE, Muñoz M, Loddenkemper C, Gross U, Göbel UB, Heimesaat MM. Novel murine infection models provide deep insights into the “ménage à trois” of Campylobacter jejuni, microbiota and host innate immunity. PLoS One 2011; 6:e20953; PMID:21698299; http://dx.doi.org/10.1371/journal.pone.0020953
- Stahl M, Ries J, Vermeulen J, Yang H, Sham HP, Crowley SM, Badayeva Y, Turvey SE, Gaynor EC, Li X, Vallance BA. A novel mouse model of Campylobacter jejuni gastroenteritis reveals key pro-inflammatory and tissue protective roles for Toll-like receptor signaling during infection. PLoS Pathog 2014; 10:e1004264; PMID:25033044; http://dx.doi.org/10.1371/journal.ppat.1004264
- Pearson BM, Gaskin DJ, Segers RP, Wells JM, Nuijten PJ, van Vliet AH. The complete genome sequence of Campylobacter jejuni strain 81116 (NCTC11828). J Bacteriol 2007; 189:8402-3; PMID:17873037; http://dx.doi.org/10.1128/JB.01404-07
- Manning G, Duim B, Wassenaar T, Wagenaar JA, Ridley A, Newell DG. Evidence for a genetically stable strain of Campylobacter jejuni. Appl Environ Microbiol 2001; 67:1185-9; PMID:11229909; http://dx.doi.org/10.1128/AEM.67.3.1185-1189.2001
- Beug H, von Kirchbach A, Döderlein G, Conscience JF, Graf T. Chicken hematopoietic cells transformed by seven strains of defective avian leukemia viruses display three distinct phenotypes of differentiation. Cell 1979; 18:375-90; PMID:227607; http://dx.doi.org/10.1016/0092-8674(79)90057-6
- Qureshi MA, Miller L, Lillehoj HS, Ficken MD. Establishment and characterization of a chicken mononuclear cell line. Vet Immunol Immunopathol 1990; 26:237-50; PMID:2176014; http://dx.doi.org/10.1016/0165-2427(90)90094-9
- van Aubel RA, Keestra AM, Krooshoop DJ, van Eden W, van Putten JP. Ligand-induced differential cross-regulation of Toll-like receptors 2, 4 and 5 in intestinal epithelial cells. Mol Immunol 2007; 44:3702-14; PMID:17493681; http://dx.doi.org/10.1016/j.molimm.2007.04.001
- Schmittgen TD, Livak KJ. Analyzing real-time PCR data by the comparative C(T) method. Nat Protoc 2008; 3:1101-8; PMID:18546601; http://dx.doi.org/10.1038/nprot.2008.73