ABSTRACT
Pseudomonas aeruginosa is among the most common pathogens responsible for both acute and chronic infections of high incidence and severity. Additionally, P. aeruginosa resistance to conventional antimicrobials has increased rapidly over the past decade. Therefore, it is crucial to explore new therapeutic options, particularly options that specifically target the pathogenic mechanisms of this microbe. The ability of a pathogenic bacterium to cause disease is dependent upon the production of agents termed ‘virulence factors’, and approaches to mitigate these agents have gained increasing attention as new antibacterial strategies. Although blue light irradiation is a promising alternative approach, only limited and preliminary studies have described its effect on virulence factors. The current study aimed to investigate the effects of lethal and sub-lethal doses of blue light treatment (BLT) on P. aeruginosa virulence factors. We analyzed the inhibitory effects of blue light irradiation on the production/activity of several virulence factors. Lethal BLT inhibited the activity of pyocyanin, staphylolysin, pseudolysin and other proteases, but sub-lethal BLT did not affect the production/expression of proteases, phospholipases, and flagella- or type IV pili-associated motility. Moreover, a eukaryotic cytotoxicity test confirmed the decreased toxicity of blue light-treated extracellular P. aeruginosa fractions. Finally, the increased antimicrobial susceptibility of P. aeruginosa treated with sequential doses of sub-lethal BLT was demonstrated with a checkerboard test. Thus, this work provides evidence-based proof of the susceptibility of drug-resistant P. aeruginosa to BLT-mediated killing, accompanied by virulence factor reduction, and describes the synergy between antibiotics and sub-lethal BLT.
Introduction
Pseudomonas aeruginosa is a Gram-negative bacterium that thrives in most natural and man-made environments. It is found in diverse habitats, including soil, water, plants and animals, and infects multiple hosts.Citation1 P. aeruginosa causes a wide variety of acute (i.e., short duration and typically severe) and chronic (i.e., long duration, often refractory to treatment, and of variable severity depending on the pathogen) human infections, including in patients with severe burn wounds, urinary tract infections, acquired immune deficiency syndrome (AIDS), lung cancer, chronic obstructive pulmonary disease, bronchiectasis and cystic fibrosis (CF).Citation2-5 Adding to the problems caused by its high incidence and the severity of infection, the resistance of P. aeruginosa to conventional antimicrobial treatments has increased over the past decade.Citation6 Despite their wide recognition, the high incidence, severity and resistance of P. aeruginosa persist, and various strategies to address these problems have been proposed, such as (i) virulence and pathogenicity factors as therapeutic targets (for a review see ref. CitationSeven); (ii) secretion systems as therapeutic targets (for a review see ref. CitationEight); (iii) cell adhesion and biofilm formation as therapeutic targets (for a review see ref. CitationNine); or (iv) quorum sensing as a therapeutic target (for a review see ref. CitationTen), as well as many others (for a review see ref. CitationEleven)Citation7-11. Therefore, it is crucial to explore new therapeutic options for P. aeruginosa infections, which may be achieved by specifically targeting its pathogenic mechanisms. The ability of a pathogenic bacterium to cause disease depends on the production of agents termed ‘virulence factors’, such as toxins and adhesion molecules, which actively damage host tissues. Targeting these virulence factors (e.g., inhibiting their production, delivery or function) has gained increasing attention as a potential new antibacterial strategy; in principle this would ‘disarm’ a pathogen and allow the host immune system a better chance of clearing the infection before the pathogen causes too much tissue damage. Its metabolic versatility, intrinsic and acquired antibiotic resistance, biofilm formation and the production of multiple virulence (disease-causing) factors make P. aeruginosa a formidable pathogen. The virulence machinery of P. aeruginosa comprises both cell-associated determinants (such as lipopolysaccharides, pili, and flagella) and numerous secreted factors (such as elastases, proteases, exotoxins, pyocyanin (PCN), and extracellular polysaccharides).
P. aeruginosa pathogenicity is strongly associated with its ability to move. The following 3 types of movement have been identified: (1) flagella-related swimming and (2) swarming and (3) type IV pili-dependent twitching. Another significant factor contributing to the virulence of P. aeruginosa is PCN (1-hydroxy-5-methylphenazine), a cytotoxic pigment secreted by the bacterium. Moreover, P. aeruginosa virulence is augmented by secreted factors that allow the bacterium to destroy host tissue, modulate the immune system, create biofilms and initiate the colonization process. LasA (staphylolysin) and LasB (pseudolysin) elastases are proteases secreted by P. aeruginosa.Citation12 Protease IV is a Pseudomonas serine protease that has been shown to degrade components of the immune system as well as fibrinogen, plasmin, and plasminogen. In addition, alkaline protease is involved in the extracellular processing of proteases; it has a fairly broad substrate range and is thought to act synergistically with other proteases.Citation13 Finally, P. aeruginosa virulence factors include hemolysins, which lyse red blood cells and release free iron from heme, allowing the bacteria to grow rapidly and leading to greater colonization of the host tissue. P. aeruginosa produces 2 elements with hemolytic properties: heat-resistant glycolipids and heat-labile phospholipase C.Citation14,15
The severity and increasing incidence of infections involving multidrug-resistant (MDR) P. aeruginosa have driven research strategies targeting virulence factors that lead to both bacterial eradication and the reduction of bacterial virulence. Major research efforts have been initiated to identify an alternative antimicrobial approach to combat bacteria without easily facilitating the development of resistance. In a recent paper published in Nature Reviews Microbiology, Karen Bush and a group of 30 scientists from academia and industry noted, “The investigation of novel non-antibiotic approaches for the prevention of and protection against infectious diseases should be encouraged, and such approaches must be high-priority research and development projects.”Citation16 The development of light-based antimicrobial therapies, including antimicrobial photodynamic therapy (aPDT) (for a review see refs. Seventeen-18) and ultraviolet C radiation (UVC) (for a review see refs. Seventeen and 20), presents an alternative to antibiotic approaches.Citation17-20 Light is part of the electromagnetic spectrum, which is composed of wavelengths ranging from radio waves to gamma rays. Electromagnetic radiation waves are electric and magnetic field fluctuations that transport energy from one location to another. Visible light (wavelengths ranging from 390 to 700 nm) is not inherently different from the other parts of the electromagnetic spectrum with the exception that the human eye can detect visible light waves. Electromagnetic radiation is also described as a photon stream of massless particles, each traveling at the speed of light and possessing wavelike properties. A photon is the smallest quantum of energy that can be transported.Citation21 Light-based antimicrobial approaches have an extremely important advantage in that they demonstrate equal bactericidal effectiveness for both MDR and wildtype (drug-sensitive) isolates.Citation22 However, the aPDT approach combining a photosensitizer (PS) and light presents several challenges, such as the introducing of a photosensitizer in close proximity to bacterial cells and/or the attachment of a PS to the cell membrane and the development of strategies leading to increased photosensitizer selectivity for microbial cells instead of the host tissue. The use of UVC irradiation also has several limitations, primarily attributable to its harmful effects on eukaryotic cells and the possibility for host tissue damage, including carcinogenesis.Citation23 Currently, a novel light-based approach, blue light therapy (BLT), is considered an attractive alternative. BLT exerts bactericidal (> 3 log10 units reduction in viable counts, up to bacterial eradication) effects without requiring the addition of exogenous photosensitizer.Citation24,25 Moreover, BLT is considered safer for host tissue because it has fewer detrimental effects on mammalian cells than UV irradiation.Citation23 Blue light has already been used clinically to treat inflammatory acne.Citation26 Moreover, the efficacy of blue light for wound infections has demonstrated.Citation24,27 The lethal and mutagenic effects of visible light radiation have been extensively investigated in bacteria. High doses of visible light lead to lethal effects primarily via the production of reactive oxygen species (ROS), which oxidatively damage proteins and lipids with a consequent loss of cell viability.Citation28 The exact mechanism underlying the antibacterial effects of blue light is not fully understood. One generally accepted hypothesis proposes that blue light leads to the excitation of intracellular photosensitizing compounds, i.e., porphyrins, which act as photosensitizers in classical aPDT. The absorption of a photon leads to the transfer of energy and the ultimate production of highly cytotoxic reactive oxygen species, particularly singlet oxygen.Citation24 In our previous study examining the S. aureus wild-type reference strain NCTC 8325–4, which is capable of endogenous porphyrin production, and an isogenic knockout mutant (8325–4 ΔhemB) in which endogenous porphyrin production was blocked, we demonstrated the requirement for endogenous porphyrins for the bactericidal activity of a 405-nm light treatment.Citation29
The bactericidal effects of blue light radiation have been studied in many organisms, including Propionibacterium acnes, Helicobacter pylori, Escherichia coli, Staphylococcus aureus and P. aeruginosa.Citation24,28,30,31 Bactericidal effects have also been observed for black-pigmented oral bacteria such as Porphyromonas gingivalis and Prevotella spp. (for a review see ref. Citation32) as well as Aggregatibacter actinomycetemcomitans.Citation32,33 Maclean et al. examined the bactericidal effects of 405-nm light against specific bacterial species, including Gram-positives Staphylococcus epidermidis, Enterococcus faecalis, Streptococcus pyogenes, and Clostridium perfringens and Gram-negative Acinetobacter baumannii, Proteus vulgaris, and Klebsiella pneumoniae, which are associated with hospital-acquired infections. The majority of Gram-positive and Gram-negative species are inactivated by light treatment.Citation34 To use blue light to treat infectious diseases, it is important to assess whether blue light causes damage to the host cells. However, to date only a few studies have assessed such damage. Recent clinical trials conducted by Kleinpenning et al. showed no significant effects of blue light on human skin.Citation35 Blue light irradiation resulted in no observed changes in studied parameters. Therefore, the use of blue light to treat skin is considered safe. Furthermore, Wang et al. did not detect apoptotic cells in blue light-irradiated mouse skin up to 24 hours after exposure, confirming its safety for use on mammalian tissue. In addition, a group from the Wellman Center for Photomedicine (WCP) investigated the potential development of P. aeruginosa tolerance to BLT. They also tested the effectiveness of antimicrobial blue light in a mouse model of wound infection caused by P. aeruginosa. P. aeruginosa did not develop tolerance to antimicrobial blue light after 10 cycles of sub-lethal inactivation, and BLT eradicated infection in a mouse model.Citation36 Moreover, in agreement with our previous studies, the WCP group implicated endogenous porphyrins, specifically coproporphyrin III, in the photodynamic effects of blue light.Citation36 The most recent paper by Halstead et al. described the antibacterial efficacy of blue light against a panel of 34 bacterial isolates (clinical and type strains), demonstrating the high susceptibility of both planktonic phase bacteria and bacterial biofilms to blue light and suggesting blue light as a novel decontamination strategy for nosocomial environments and infections.Citation27
Thus, this study sought analyze the effects of blue light irradiation on P. aeruginosa in the absence of an exogenously administered PS in the context of 2 major cell surface virulence factors (flagella and pili), secreted virulence factors (such as PCN, elastases, lipases, and hemolysins), and proteolytic activity. Phototherapy primarily acts by inducing the production of reactive oxygen species and leads to the photodestruction of target cells; thus, we hypothesized that the oxidative-related destruction of bacterial cells would significantly decrease the activity of virulence factors as well as motility-mediated structures such as flagella or pili and mitigate P. aeruginosa pathogenicity. This paper describes the high bactericidal effectiveness of BLT and its inactivation of numerous P. aeruginosa virulence factors.
Results
Blue light irradiation exerts strong bactericidal effects against P. aeruginosa strains
We subjected 15 strains (3 wild-type reference strains and 12 clinical isolates), including both wild-type (drug-sensitive) and MDR P. aeruginosa, to blue light irradiation. Phototreatment responses of the different strains were heterogeneous, ranging from 5.2 to 8 log10-unit reductions in viable counts (colony-forming units (CFU)/ml) under similar experimental conditions (at a fluence of 50 J/cm2) (). Regardless of the level of antimicrobial strain resistance, BLT reduced microbial viability by ≥ 5 log10 units, suggesting blue light inactivation is an alternative bactericidal approach in the fight against MDR and extensively drug-resistant (XDR) P. aeruginosa isolates (). To investigate the effects of BLT on the production and/or activity of virulence factors, lethal and sub-lethal doses of blue light irradiation were evaluated because only experiments using live microbial cells (treated with sub-lethal BLT doses) permit the effects of BLT on virulence factors production to be determined. Lethal and sub-lethal conditions are defined based on whether direct damage is sufficient or insufficient to destroy the majority of a bacterial populationCitation37. In this study, lethal and sub-lethal damage were respectively defined as viable count reductions of ≥ 3 log10 and 0.5–2 log10 unit. BLT sub-lethally inactivated P. aeruginosa strains at a fluence of up to 10 J/cm2 ().
Figure 1. In vitro BLT efficacy against P. aeruginosa. Planktonic microbial cell suspensions (108 CFU/ml) were illuminated with blue light fluences ranging from 0 to 50 J/cm2 (15.7 mW/cm2; λ 405 nm; duration of irradiation: 0 to 3184 sec). Fluences up to 10 J/cm2 reveal sub-lethal efficacy against P. aeruginosa isolates. (A) BLT efficacy against reference wild-type P. aeruginosa strains; (B) BLT activity against drug-sensitive clinical P. aeruginosa isolates; (C) BLT activity against MDR clinical P. aeruginosa isolates. MDR, multidrug-resistant; XDR, extensively drug-resistant. The values are the means of 3 separate experiments (3 independent experiments with 3 repetitions per sample), and the bars represent the log relative error according to http://faculty.washington.edu/stuve/log_error.pdf. The test was performed for reference P. aeruginosa isolates PAO1, PA14, and PAK and clinical isolates no. 1959/o, 3146/s, 3404/p, 133/K, 23/K, 3752/sz, 153/s, 2284/p, 556/K, 4190/pA, 3109/o, and 143/p.
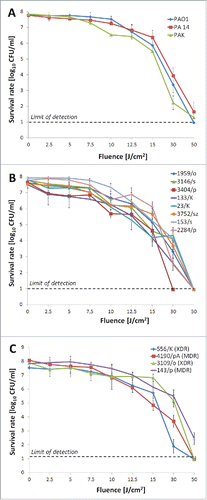
Lethal BLT
Lethal BLT affects PCN activity
PCN was analyzed and quantified in all P. aeruginosa isolates as well as light treated supernatants from bacterial cultures to investigate the impact of BLT on PCN activity. PCN activity was significantly reduced by BLT (). Mutant strains that were unable to produce PCN demonstrated higher susceptibility to blue light irradiation than the PCN-producing parent strain ().
Figure 2. PCN activity during BLT. (A) PCN detection and quantification during BLT. PCN activity in P. aeruginosa extracellular fractions was examined before and upon light irradiation (fluence 50 J/cm2; 15.7 mW/cm2; λ 405 nm; duration of irradiation: 3184 sec). The values are the means of 3 separate experiments (the assay was performed for 3 independent experiments with 3 repetitions per sample), and the bars represent the standard error (SE) according to Cumming et al.Citation81 The test was performed for the PCN-producing strains: PAO1 and PA14 (reference strains) as well as 1959/o, 3404/p, 133/K, 23/K, 153/s, 2284/p, 556/K, and 4190/pA (clinical isolates). (B) Photo protective effects of PCN. Planktonic cultures of P. aeruginosa isogenic strains (108 CFU/ml) were illuminated with blue light fluences ranging from 0 to 50 J/cm2 (15.7 mW/cm2; λ 405 nm; duration of irradiation: 0 to 3184 sec). The solid line indicates the wild-type P. aeruginosa reference strain, and the dotted lines indicate isogenic derivatives expressing different phenazines (detailed description in ). The values are the means of 3 separate experiments (3 independent experiments with 3 repetitions per sample), and the bars represent the log relative error according to http://faculty.washington.edu/stuve/log_error.pdf.
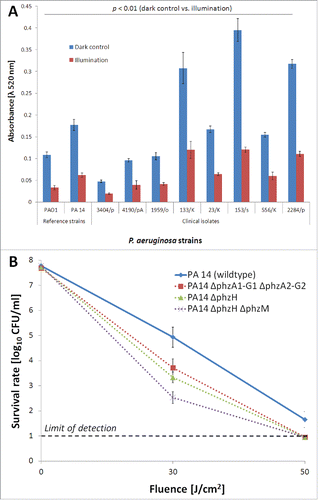
Table 1. Reference P. aeruginosa strains used in the study.
Elastase A activity
An S. aureus-based assay was employed to investigate the effects of lethal BLT on elastase A activity. All of the isolates studied yielded similar results (). Based on absorbance measurements at λ 600 nm, the time required for elastase A to lyse the same amount of heat-inactivated S. aureus cells was longer for blue light-treated extracellular fractions of a P. aeruginosa culture; thus lethal BLT decreased elastase A activity. Because a wide range of delta-t values was obtained, only the endpoints for all strains are shown in . The time point at which approximately 80% of the maximum activity (absorbance = 0.2) was observed for non-irradiated supernatants is shown for all strains in a bar chart. Time-curves are included in the Supplementary Data. The symbol Δt indicates the change in time required for the comparable lysis of S. aureus cells for BLT-treated and non-illuminated elastase A. Although each P. aeruginosa isolate demonstrated a different ratio for elastolytic activity, longer amounts of time, ranging from from 30 to 85 min, were observed for all strains (Supplementary Data).
Figure 3. Elastase activity during BLT. (A) Elastase (A)activity during BLT. The staphylolysin activity of elastase A was determined using a reference heat-inactivated S. aureus Newman strain treated with P. aeruginosa extracellular fractions (“dark control:” kept in the dark; “illumination:” BLT; fluence 50 J/cm2; 15.7 mW/cm2; λ 405 nm; duration of irradiation: 3184 sec), and OD600 values were measured every 5 min during incubation (37°C). The figure represents the time at which the dark control supernatants reached 80% of maximum elastolytic activity. The values are the means of 3 separate experiments (the assay was performed for 3 independent experiments with one repetition per sample), and the bars represent the SE. The maximum value of absorbance was set as 1, and all other measurements were normalized as a ratio of 1. (B) Elastase (B)activity during BLT. The pseudolysin activity of elastase B was analyzed by performing and ECR assay. The reaction buffer containing ECR was treated with P. aeruginosa extracellular fractions (“dark control:” kept in the dark; “illumination:” BLT; fluence 50 J/cm2; 15.7 mW/cm2; λ 405 nm; duration of irradiation: 3184 sec), and Congo Red release was quantified by measuring the absorbance at 495 nm, which is indicative of elastase B activity. The values are the means of 3 separate experiments (the assay was performed for 3 independent experiments with one repetition per sample), and the bars represent the SE. The test was performed for the elastase B-producing strains PAO1 and PAK (reference strains) as well as 1959/o, 3146/s, 3404/p, 3752/sz, 556/K, and 143/p (clinical isolates). *P < 0.05, **P < 0.01 (dark control vs. illumination).
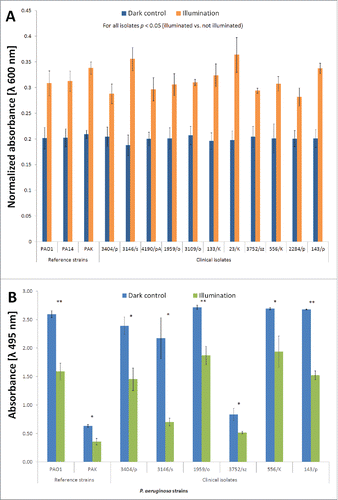
Elastase B activity
An Elastin Congo Red (ECR) assay revealed the inactivating effects of elastase B by BLT. Optical density measurements of the irradiated and non-irradiated extracellular fractions in the presence of ECR at λ 495 nm revealed decreased elastase B activity following BLT (). The production of elastase B was heterogeneous in the P. aeruginosa population, and light-dependent reduction in elastase B activity was observed regardless of LasB levels ().
Overall proteolytic activity
A modified skim milk assay was performed to investigate the effects of BLT on the total proteolytic activity of the strains by measuring turbidity changes in milk during incubation with extracellular fractions of P. aeruginosa isolates. A significant decrease in proteolytic activity was observed for BLT supernatants, indicating the ability of lethal BLT to inactivate bacterial proteases. Similar results were observed for all P. aeruginosa strains (). The bar graph shows the endpoint results for all strains at 80% maximum activity (absorbance = 0.2) in the non-irradiated supernatants. The endpoint for 40% maximum activity is shown for strain 3109/o because 80% was not reached (). Time-curves showing changes in the time required for proteolytic agents to lyse the same amount of skim milk proteins are presented in the Supplementary Data. Although each P. aeruginosa isolate demonstrated a different ratio for proteolytic activity, longer amounts of time, ranging from 10 to 110 min, were observed for all strains (Supplementary Data).
Figure 4. Proteolytic activity during BLT. The overall proteolytic activity of P. aeruginosa was determined by measuring the changes in milk turbidity during incubation with bacterial extracellular fractions (“dark control:” kept in the dark; “illumination:” BLT; fluence 50 J/cm2; 15.7 mW/cm2; λ 405 nm; duration of irradiation: 3184 sec). OD600 values were measured every 5 min during incubation (37°C and 200 rpm). The figure represents the time at which the dark control supernatants reached 80% of the maximum proteolytic activity. The values are the means of 3 separate experiments (the assay was performed for 3 independent experiments with one repetition per sample), and the bars represent the SE. The maximum value of absorbance was set as 1, and all other measurements were normalized as the ratio of 1. # indicates strains for which 80% proteolytic activity was not achieved.
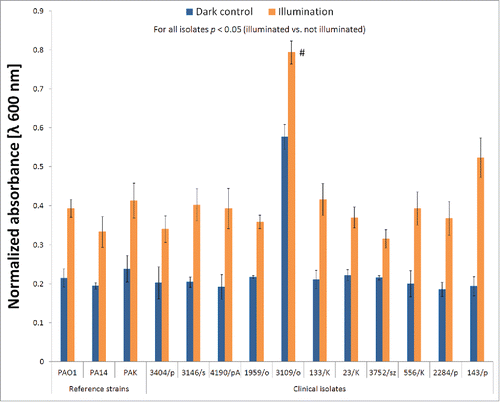
Hemolytic activity
We used fresh sheep red blood cells (RBC) to identify and quantify hemolytic agents (i.e., phospholipase C) in all P. aeruginosa isolates. The extracellular fractions of strains that demonstrated high production of hemolytic agents were subsequently irradiated with a lethal dose of blue light to investigate the effects of BLT on hemolysin activity, after which the hemolytic activity of the irradiated supernatants was measured in an RBC assay (). Hemolysin production by P. aeruginosa was heterogeneous, with strains exhibiting various levels of hemolytic agents. However, BLT did not affect the hemolytic activity of the strains; thus, P. aeruginosa hemolysins were not inactivated by blue light irradiation up to a fluence of 50 J/cm2.
Figure 5. Hemolytic activity during BLT. The hemolytic activity of P. aeruginosa was evaluated using fresh sheep RBCs treated with P. aeruginosa extracellular fractions (“dark control:” kept in the dark; “illumination:” BLT; fluence 50 J/cm2; 15.7 mW/cm2; λ 405 nm; duration of irradiation: 3184 sec). The amount of hemoglobin released was assessed by measuring the absorbance at λ 540 nm. The percentage (%) of lysed cells was calculated as follows: % = [(X-B)/(T-B)] × 100 (where B (baseline) is a negative control corresponding to the RBCs incubated with sterile medium, and T is a positive control corresponding to the total lysis obtained by incubating RBCs with a medium supplemented with 0.1% SDS). The values are the means of 3 separate experiments (the assay was performed for 3 independent experiments with one repetition per sample), and the bars represent the SE. The test was performed for all hemolysin-producing P. aeruginosa isolates: PAO1 (reference strain) and clinical isolates no. 3146/s, 4190/pA, 1959/o, 133/K, 23/K, 153/s, 556/K, 2284/p, and 143/p.
![Figure 5. Hemolytic activity during BLT. The hemolytic activity of P. aeruginosa was evaluated using fresh sheep RBCs treated with P. aeruginosa extracellular fractions (“dark control:” kept in the dark; “illumination:” BLT; fluence 50 J/cm2; 15.7 mW/cm2; λ 405 nm; duration of irradiation: 3184 sec). The amount of hemoglobin released was assessed by measuring the absorbance at λ 540 nm. The percentage (%) of lysed cells was calculated as follows: % = [(X-B)/(T-B)] × 100 (where B (baseline) is a negative control corresponding to the RBCs incubated with sterile medium, and T is a positive control corresponding to the total lysis obtained by incubating RBCs with a medium supplemented with 0.1% SDS). The values are the means of 3 separate experiments (the assay was performed for 3 independent experiments with one repetition per sample), and the bars represent the SE. The test was performed for all hemolysin-producing P. aeruginosa isolates: PAO1 (reference strain) and clinical isolates no. 3146/s, 4190/pA, 1959/o, 133/K, 23/K, 153/s, 556/K, 2284/p, and 143/p.](/cms/asset/d37c7d9c-f216-40b0-9a40-48c332f6a6e0/kvir_a_1250995_f0005_oc.gif)
Cytotoxicity of illuminated bacterial suspensions
We analyzed the extracellular fractions of bacterial cultures for all P. aeruginosa isolates to confirm significant reductions in virulence factor activity by blue light irradiation. We treated human keratinocytes with filtered bacterial supernatants that had been illuminated with a lethal dose of BLT (50 J/cm2) or left untreated and measured the amounts of viable keratinocytes after 24 hrs (). With this experiment, we sought to confirm the ability of phototreatment with blue light to affect the potency of secreted bacterial virulence factors. P. aeruginosa isolates exhibited a range of virulence, but for all strains, the toxicity of the extracellular fractions to human dermal keratinocytes was significantly reduced by blue light illumination (). The viability of human cells treated with BLT-exposed P. aeruginosa supernatants increased because BLT is associated with reduced secreted virulence factor activity suggesting additional benefits derived from the use of a light-based antibacterial strategy to treat infectious diseases.
Figure 6. Toxicity of illuminated P. aeruginosa extracellular fractions to keratinocytes. The extracellular fractions from all P. aeruginosa isolates were collected by centrifugation, filtered through 0.22-μm syringe filters, illuminated with blue light (50 J/cm2; 15.7 mW/cm2; λ 405 nm; duration of irradiation: 3184 sec) and incubated with keratinocytes for 24 h at 37°C. Cell survival was determined by performing an MTT assay. The values are the means of 3 separate experiments (3 independent experiments with 3 repetitions for each sample), and the bars represent the SE. The test was performed for the reference P. aeruginosa isolates PAO1, PA14, and PAK and clinical isolates no. 1959/o, 3146/s, 3404/p, 133/K, 23/K, 3752/sz, 153/s, 2284/p, 556/K, 4190/pA, 3109/o, and 143/p.
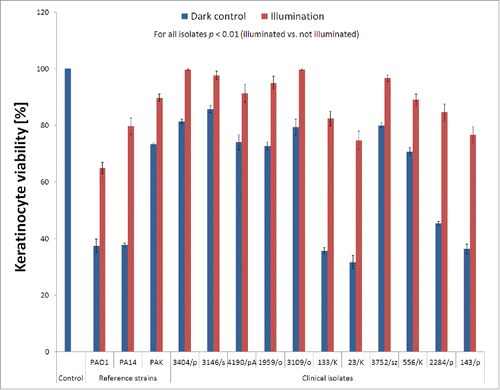
Sub-lethal BLT
Sub-lethal BLT does not affect virulence factor production/activity
The application of sub-lethal BLT to all P. aeruginosa isolates permitted us to identify its effects on the production and/or activity of virulence factors including (i) proteolytic enzymes (i.e., elastases A and B, serine protease IV, and alkaline protease), (ii) lipolytic agents (phospholipase C and lipases), and (iii) bacterial motility (swimming, swarming, and twitching). Sub-lethal BLT demonstrated no effects on the production/activity of these virulence factors. The experiments were performed for all strains, but shows an example of the results for isolate no. 133/K, which possesses all of the virulence factors mentioned. Semi-quantitative measurement (halo diameter) indicated no changes in virulence factor activity after BLT. For the dark control and illuminated samples, we obtained the following halo measurements: 20 mm ± 1 (for proteolytic agents); 20 mm ± 2 (for lipolytic agents); 24 mm ± 2 (for swimming motility); 19 mm ± 2 (for swarming motility), and 14 mm ± 3 (for twitching motility).
Figure 7. Sub-lethal BLT does not affect virulence factor production/activity. By applying sub-lethal BLT to P. aeruginosa isolates, we analyzed the effects of phototreatment on the production and/or activity of virulence factors. (upper panel) Proteolytic enzymes (i.e., elastases A and B, serine protease IV, and alkaline protease). Skim milk agar plates were employed to analyze the overall proteolytic activity of the bacteria. P. aeruginosa cultures (“dark control:” kept in the dark; “illumination:” sub-lethal BLT; fluence 10 J/cm2; 15.7 mW/cm2; λ 405 nm; duration of irradiation: 637 sec) were transferred onto agar plates and incubated for 18 hrs at 37°C; clear zones around the colonies were indicative of total proteolytic activity. The test was performed for all P. aeruginosa strains employed in the study, but the results for only one strain (no. 133/K) are shown in the figure. (middle panel) Lipolytic agents (phospholipase C and lipases). Lipolytic activity was analyzed using egg yolk agar plates. Bacterial cultures (“dark control:” kept in the dark; “illumination:” sub-lethal BLT; fluence 10 J/cm2; 15.7 mW/cm2; λ 405 nm; duration of irradiation: 637 sec) were transferred onto agar plates and incubated for 48 hrs at 37°C. After 24 hrs, white precipitate halos around the colonies were indicative of phospholipase and lipase activity. The test was performed for all P. aeruginosa strains employed in the study, but the results for only one strain (no. 133/K) are shown in the figure. (lower panels) Bacterial motility (swimming, swarming, and twitching). Swimming and swarming motility assays were performed using M8 agar plates, and twitching motility was analyzed on LB agar plates. After incubation, bacterial growth zones were measured. The motility test was performed for all P. aeruginosa strains used in the study, but the results for only one strain (no. 133/K) are shown in the figure. Each motility assay was performed for 3 independent experiments, with one repetition per sample.
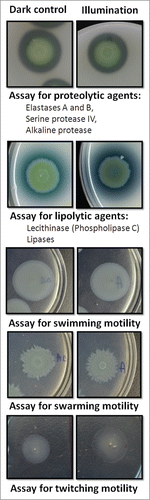
Sub-lethal BLT renders drug-resistant P. aeruginosa susceptible to antimicrobials
A checkerboard assay confirmed the increased susceptibility of P. aeruginosa isolates (clinical strain no. 3404/p, 556/K, 23/K and 3752/sz) pre-treated with sub-lethal BLT to the antimicrobial agents gentamycin, ceftazidime and meropenem (). Sequential blue light irradiation and antibiotic administration controlled bacterial growth at minimum inhibitory concentration (MIC) values that were 2-, 8-, and even 6four-fold lower. The most pronounced effects occurred for ceftazidime () preceded by irradiation with a blue light fluence of 12.5 J/cm2 resulting in a 6four-fold decrease in the MIC value required for P. aeruginosa. In addition, the minimal bactericidal concentrations (MBCs) of antimicrobials acting synergistically with BLT were lower for blue light pre-treated P. aeruginosa strains (). Moreover, no bacterial re-growth was observed at 24 hrs after the combination treatment.
Figure 8. BLT renders P. aeruginosa susceptible to gentamycin, ceftazidime and meropenem. A checkerboard titration method was employed to analyze the effects of sequential BLT combined with the application of antimicrobial agents. We combined various concentrations of antimicrobial agents with a range of sub-lethal doses of blue light irradiation; antibiotics were added to P. aeruginosa cultures after BLT). The plates were incubated overnight at 37°C in the dark. After incubation, OD600 values were measured to evaluate MIC changes. The values are the means of 3 separate experiments. Raw data indicating SD and SE are included in the Supplementary Data. Panels represent results for various strains: (A) strain no. 3404/p, (B) strain no. 556/K, (C) strain no. Twenty-three/K, and (D) strain no. 3752/sz.
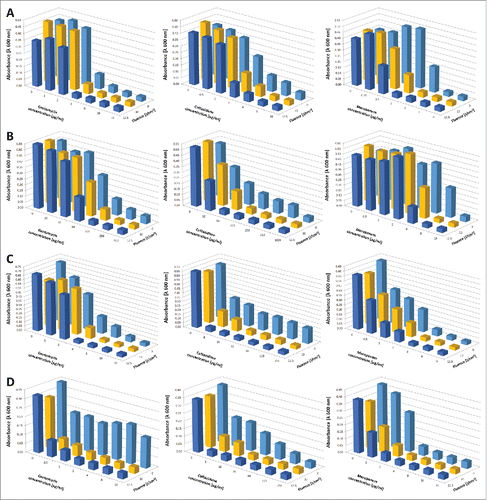
Table 2. Clinical P. aeruginosa isolates used in the study.
Table 3. Minimal Bactericidal Concentration change upon blue light treatment.
Discussion
In this study, we tested the effects of BLT on several reference and clinical isolates. Notably, all of the strains were highly susceptible to BLT and demonstrated reductions in survival above 5 log10 CFU/ml and as high as 8 log10 units. Thus, according to the American Society for Microbiology, this new approach warrants the designation “antimicrobial.“Citation38 Moreover, high bactericidal effectiveness was achieved in both wild-type and MDR isolates, including XDR strains. This is the first report demonstrating the bactericidal effectiveness of phototherapy against XDR P. aeruginosa isolates, indicating the high potential of the BLT approach for the development of light-mediated therapeutic options to treat Pseudomonas infections.
P. aeruginosa secretes a number of cytotoxic factors that exert deleterious effects in cells. One of these factors is PCN (1-hydroxy-5-methylphenazine), a blue pigment with redox properties that is important for bacterial virulence and survival. PCN has deleterious effects on a variety of host cell types, including epithelial and endothelial cells and leukocytes.Citation39 Few effective methods to inactivate this cytotoxin are available. The exposure of PCN to Rose Bengal (RB) or riboflavin and visible light causes irreversible bleaching concomitant with partial inactivation of the pigment. Based on the observed inhibition of PCN oxidation by azide, 1O2 has been proposed as a major oxidant.Citation40 In animal models of acute and chronic lung infection, PCN is essential to P. aeruginosa virulence. Additionally, due to its known oxidoreductive properties, PCN oxidizes glutathione, inactivates catalase in respiratory epithelial cells and thus participates in oxidative-stress related damage.Citation41 Although no therapeutic strategies directly target PCN, several antioxidant therapies have proven useful. An antioxidant-supplemented regimen involving the delivery of glutathione via aerosolization improved the lung function of cystic fibrosis patients after 8 weeks.Citation42 Recently, the inhibition of PCN synthesis has been suggested as a therapeutic strategy, although studies have not yet been carried out.Citation43,44 Our results demonstrate the ability of BLT to effectively inactivate PCN ; thus, BLT represents a potential therapeutic approach to target this virulence factor, ultimately leading to reductions in P. aeruginosa virulence and the diminished severity of infection outcomes.
PCN is synthesized and secreted by P. aeruginosa and appears to be a primary target for photodynamic degradation. PCN is an intensely colored blue pigment in pH neutral and alkaline solutions (λmax 660 nm) and pink in acidic buffers (λmax 520 nm).Citation45 Thus, we hypothesized that PCN protects P. aeruginosa against photooxidative injury by absorbing incident light in the visible region of the spectrum and scavenging bactericidal species generated by photosensitization, such as 1O2; both of these processes may affect the efficacy of photodynamic treatment. Successful killing of P. aeruginosa and the suppression of its virulence may also require the elimination of PCN. A recent study unambiguously confirmed the interaction of PCN and 1O2 with a high rate constant suggesting the ability of PCN to initially photoprotect P. aeruginosa against photodynamic eradication by scavenging this toxic agent.Citation40 However, the same reaction causes irreversible pigment bleaching implying the degradation of the phenazine chromophore. Based on the results of this study, PCN may indeed photoprotect bacteria against light-based treatment by lowering the susceptibility of P. aeruginosa to BLT. The bactericidal effectiveness of BLT for isogenic mutants lacking PCN was higher than for the wild-type (PCN-producing) strain. Thus, photo-based inactivation of PCN may suppress bacterial virulence and appears to be a useful photochemical/pharmacological approach to eradicate P. aeruginosa from infected tissue.Citation40
Additionally, the pathogenicity of P. aeruginosa depends on enzymatic activity. Secreted virulence factors allow the bacteria to destroy host tissue by cleaving proteins, affecting the immune system, creating biofilms and colonizing tissue. P. aeruginosa produces numerous extracellular substances that play roles in its pathogenesis. The elastases LasB and LasA are among the most commonly secreted P. aeruginosa proteases and are classic metalloproteases.Citation12,46 However, the total proteolytic activity of P. aeruginosa arises not only from LasA and LasB but also other secreted proteases such as protease IV and alkaline proteases.Citation13 This study reveals the ability of BLT to significantly inhibit the proteolytic activity of P. aeruginosa, potentially reducing its virulence and lessening the severity of an infection.
Motility, arguably one of the most impressive features of microbial physiology, facilitates rapid colonization of the airway. Cellular structures responsible for bacterial motility, such as flagella or pili, are crucial during the adhesion phase of colonization and bind to asialoGM1 in the epithelial cell membrane.Citation47,48 Furthermore, both pili-mediated adherence and twitching motility are critical to P. aeruginosa virulence. In an infant mouse model of lung infection, piliated strains of P. aeruginosa caused more severe and diffuse pneumonia than corresponding non-piliated mutants.Citation43 Unfortunately, based on the current study, BLT does not target flagella and type IV pili because it did not affect P. aeruginosa movement. However, this effect was analyzed using a sub-lethal dose of BLT, which does not exclude the possibility that lethal BLT targets the cellular structures responsible for bacterial movement.
The hemolytic activity of P. aeruginosa is another significant virulence factor. In combination with other extracellular toxins, hemolysins threaten human life by causing considerable cytopathology at the site of an infection. P. aeruginosa produces and secretes hemolytic substances such as heat-resistant glycolipids and heat-labile phospholipase C.Citation14 Unfortunately, in this study, BLT did not inactivate the agents responsible for P. aeruginosa hemolytic activity.
Notably, this paper is the first report to describe the inactivation of toxic bacterial exoproducts and virulence factors of a clinically relevant pathogen by BLT. Interestingly, the effects of blue light on bacterial virulence and motility have been observed in the plant pathogen Pseudomonas syringae.Citation49 A small number of studies in P. aeruginosa have proposed the photoinactivation of secreted proteases and phospholipase C via photodynamic treatment with exogenously administered sensitizers.Citation50,51 Proteases were significantly reduced by irradiation with red light in the presence of the photosensitizer toluidine blue O (TBO) in a dose-dependent manner, in terms of the light energy dose and the TBO concentration. Similarly, only a few studies have described the inactivating effects of aPDT in combination with exogenously administered PSs in other bacterial species. Tubby et al. reported the inactivation of staphylococcal virulence factors using a light-activated antimicrobial agent. Protease, α-hemolysin and sphingomyelinase activities were inhibited in a dose-dependent manner following exposure to a laser light in the presence of methylene blue.Citation52 Recently, Bartolomeu et al. the effects of photodynamic treatment using the exogenous sensitizer 5,-10,-15,-20-tetrakis(1-methylpyridinium-4-yl)porphyrin tetra-iodide (Tetra-Py(+)-Me) on catalase activity, β hemolysis, lipases, thermonuclease, enterotoxins, and coagulase production in S. aureus.Citation53 Kato et al. described the antimicrobial photodynamic inactivation of Candida albicans virulence factors and a reduction in the in vivo pathogenicity of this microbe. Mice systemically infected with C. albicans pre-treated with aPDT demonstrated significantly increased survival compared to mice infected with untreated yeast.Citation54 Thus, photodynamic therapy in combination with exogenously administered sensitizers reduces the harmful impacts of preformed virulence factors on the host. The current study is the first report of virulence factor inactivation by blue light alone. The blue light-mediated inactivation of virulence factors likely results from the ROS activity generated during photodynamic processes. ROS easily interacts with various biomolecules, leading to their destruction. The ROS-mediated inactivation of 2 virulence factors was observed for aPDT in the presence of an exogenous photosensitizer.Citation50 However, even after the successful inactivation of an infecting microorganism, virulence factors may still be present and cause significant damage to host tissues. Moreover, a number of in vitro and in vivo studies have shown an increase in endotoxins and hydrolytic enzymes (proteases, alkaline phosphatase, phospholipase C, and peptidoglycan hydrolase) released after exposure to different antibiotics.Citation55,56 These virulence factors exert deleterious effects even after the pathogen has been eradicated.Citation57,58 BLT has potential for the treatment of topical infections; thus, if it also inactivates microbial virulence factors, it has an advantage over conventional antibiotic therapy.
Notably, all experiments were performed with stationary-phase P. aeruginosa cultures in which all relevant virulence factors were already expressed. Thus, the rationale for using cell-free supernatants to analyze the BLT-mediated inactivation of virulence factors may be called into question. During photodynamic processes, bacterial inactivation occurs via the loss of cell-membrane integrity and cell lysis, and all cytoplasmic components leak into the supernatant. If we would irradiate the cell culture and collect the supernatants after the treatment one could speculate that some other than photodynamic processes could occur and result in reduction of virulence factors activity. Moreover, photoinactivation utilizes the extracellular fractions of endogenous porphyrins in cell-free supernatants. Our results therefore suggest the realization of similar virulence factor photoinactivation, even in cell-free areas of infected sites in vivo.
Finally, we provide evidence of the ability of BLT to affect secreted virulence factors and decrease the toxicity of extracellular P. aeruginosa fractions to human cells, which is an additional benefit of using BLT to treat infectious diseases. We have not investigated all molecules and substances in the culture supernatant that are potentially destroyed, and thus we are unable to suggest specific molecules that are responsible for the reduced toxicity of irradiated supernatants; however, the general toxicity of the extracellular fractions was clearly reduced by photoinactivation.
A significant achievement of the current study was the observed synergism of sequential BLT and antibiotics, leading to the increased susceptibility of P. aeruginosa to antimicrobial agents. To our knowledge, this is the first report providing evidence of possible interactions between BLT and antimicrobial agents in P. aeruginosa. However, synergy between antibiotics and photodynamic inactivation with exogenously administered photosensitizers has been previously described in other bacterial species. Cassidy et al. observed the higher efficacy of photodynamic antimicrobial inactivation in combination with antibiotics for the treatment of a Burkholderia cepacia complex infection.Citation59 For Staphyloccocus, Dastgheyb et al. demonstrated the additive antimicrobial activity of an illuminated porphyrin sensitizer with chloramphenicol and tobramycin in S. aureus, as well as synergistic activity against MRSA (methicillin-resistant S. aureus) and Staphylococcus epidermidis.Citation60 Similarly, Ronqui et al. showed the synergistic antimicrobial effects of photodynamic therapy with methylene blue and ciprofloxacin against S. aureus and E. coli.Citation61 Furthermore, Chibebe et al. observed the ability of photodynamic and antibiotic therapy with vancomycin to impair the pathogenesis of Enterococcus faecium in a whole-animal insect model using Galleria mellonella.Citation62 Drug resistance is a significant virulence factor that facilitates bacterial spread and leads to infection. Based on our study, blue light irradiation decreases the resistance of P. aeruginosa to various antimicrobials, such as gentamycin, ceftazidime, and meropenem, and hypothetically to other antimicrobial agents as well. Notably, this effect was achieved with sub-lethal doses of BLT, implying realization of the same results in infection site areas for which lethal BLT is not feasible due to inadequate tissue penetration by the light.
Although there were several limitations to this study, such as its focus on a single bacterial species and on planktonic rather than biofilm cultures, as well as the relatively small number of virulence factors tested, it provides valuable insights into BLT and, in particular, demonstrates the potency of BLT for reducing virulence factor activity.
P. aeruginosa is an example of a bacterial pathogen whose resistance is an issue of public concern; however, the current study suggests BLT may easily eradicate this bacterium, regardless of its level of drug resistance. Moreover, BLT is not only an effective tool for bacterial inactivation, reducing cell viability up to 8 log10 units, but is also beneficial for targeting and reducing the activity of several P. aeruginosa virulence factors.
MDR P. aeruginosa still represents a significant fraction of circulating strains according to the latest Antimicrobial Resistance Surveillance Report. The development of new tools to treat chronic infections caused by biologically and clinically relevant strains resistant to classical antibiotics is of great importance for preventing the spread of these variants via, for example, nosocomial transmission, as well as in community-associated settings. Although one of the most pronounced limitations of this study is its focus on planktonic rather than biofilm growth, we remain convinced of the evidence-based proof that this study provides regarding BLT-mediated P. aeruginosa eradication and virulence factor reduction, leading to the development of a new non-antibiotic therapeutic approach to protect human health.
Materials and methods
Strains and culture conditions
The reference P. aeruginosa strains used are listed in . The 12 clinical P. aeruginosa isolates, were provided by Dr. Hab Julianna Kurlenda and isolated from patients hospitalized in the Provincial Hospital in Koszalin and a hospital in Gdansk (). The microorganisms were grown in Luria-Bertani (LB) broth or on LB agar (Oxoid, United Kingdom). The isolates were categorized as susceptible, intermediate, or resistant according to the Clinical and Laboratory Standards Institute (CLSI) breakpoint guidelines (for a review see ref. Citation63) and drug resistance patterns were determined according to the guidelines of the European Center for Disease Prevention and Control (ECDC) and the Centers for Disease Control and Prevention (CDC).Citation63,64
Light source
Illumination was provided with a single-emitter diode lamp (405 nm, 120 W; output light with a power of 11 W/12 mm2; full width at half maximum (FWHM) of 10 nm), equipped with a panel controller and PC software (SecureMedia, Poland).
Visible light irradiation studies
Bacterial cultures were grown overnight (16 hrs) at 37°C in LB broth and then diluted to 108/ml bacterial cells. The cells were then transferred to a 96-well microtiter plate (100 µl per well) and illuminated with the selected blue light fluence, ranging from 0 to 50 J/cm2 (15.7 mW/cm2; λ 405 nm; duration of irradiation: 0 to 3184 sec) at room temperature. Following illumination, 10-µl aliquots were obtained to determine the CFU. The contents of the wells were mixed before sampling, and the aliquots were serially diluted 10-fold in PBS (0.13 mM NaCl, 8.1 mM Na2HPO4, 2.68 mM KCl, 1.47 mM KH2PO4) to achieve final dilutions of 10−1 to 10−6, which were then streaked horizontally as previously described.Citation65 The control consisted of bacteria incubated in the dark for the same amount of time as the bacteria exposed to light. Each experiment was carried out 3 times (3 independent experiments with 3 repetitions per sample; the time interval between the 3 replicates was 24 hrs). The survival fraction was expressed as the ratio of the CFU of bacteria treated with blue light to the CFU of untreated bacteria. The detection limit was 10 CFU/ml.
Determination of lethal and sub-lethal doses
A sub-lethal dose was defined as a dose that did not eliminate the majority of the microbial population. The lethal dose was defined as the dose that eliminated ≥ 99.9% of the bacterial population.Citation66 In this study, the lethal dose was determined under photodynamic conditions as the dose that reduced the number of bacterial cells by ≥ 3 log10. A sub-lethal dose reduced the number of bacteria by 0.5 – 2 log10. Sub-lethal doses were defined according to previous publications.Citation36,37,67-69
Preparation of P. aeruginosa cultures supernatants
Bacterial supernatants were prepared to determine PCN concentrations and evaluate protease, elastase and hemolysin activities according to a previously published protocol.Citation70 Fresh overnight liquid bacterial cultures in LB were diluted to optical density (OD)600 = 1. A total of 0.25 ml of this dilution was transferred into 25 ml of fresh LB in a 250-ml glass flask and incubated overnight at 37°C at 150 rpm. After overnight incubation, the culture was transferred into Falcon tubes and centrifuged (10,000 rpm for 10 min), and the supernatant filtered through a 0.22-µm syringe filter. The supernatant was then transferred to a 24-well microtiter plate (600 µl per well) and illuminated with the selected blue light fluence at room temperature. Following irradiation, the supernatants were stored at −20°C for further analysis.
PCN detection and quantification
The concentration of PCN in the supernatant was determined before and after light irradiation. A total of 2.5 ml of supernatant and 1.5 ml of chloroform (Sigma-Aldrich, Germany) were mixed and centrifuged for 5 min at 4,500 rpm. The chloroform phase was transferred into a new tube and mixed with 0.5 ml of 0.2 M HCl (Avantor Performance Materials, Poland). The sample was then centrifuged at 4,500 rpm for 8 min. The pink-colored acidic phase was transferred to a 96-well plate, and the absorbance at λ 520 nm was measured.Citation70 The assay was performed for 3 independent experiments with 3 repetitions per sample. The detection limit was 2.5 μM.Citation71
Hemolytic activity
To analyze the hemolytic activity of the bacterial supernatant, fresh sheep RBC (Innovative Research, USA) were employed using a previously described technique.Citation15 The cells were washed 3 times in PBS (centrifuged for 5 min at 6000 rpm) and resuspended in RPMI-1640 medium (Roswell Park Memorial Institute, RPMI) (Sigma-Aldrich, Germany) to a final concentration of 2% (cell volume/medium volume). Next, 700 µl of the prepared RBC solution was mixed with 300 µl of the supernatant in an Eppendorf tube. The tubes were incubated horizontally at 37°C and 150 rpm for 25 min. After incubation, the suspension was centrifuged (10,000 rcf, 10 min, 4°C), and 240 µl of the sample was transferred into a 96 well plate. The released hemoglobin was determined by measuring the absorbance at λ 540 nm. The percentage (%) of lysed cells was calculated as follows: % = [(X-B)/(T-B)] × 100 (where B (baseline) is a negative control corresponding to RBCs incubated with 300 μl of sterile LB and T is a positive control corresponding to the total lysis obtained by incubating RBCs with 300 µl of LB supplemented with 0.1% SDS (Sigma-Aldrich, Germany)). The assay was performed for 3 independent experiments with one repetition per sample.
Elastolytic activity
Elastase A (staphylolysin) activity
Staphylococcus aureus cells were employed to analyze staphylolysin activity according to a previously described method.Citation72 A reaction buffer consisting of 0.02 M Tris-HCl (Sigma-Aldrich, Germany) at pH 8.5 was prepared and sterilized. One colony of the S. aureus Newman strain was transferred into 250 ml of LB broth and incubated overnight (37°C, 150 rpm). The bacteria were centrifuged, washed twice with reaction buffer and resuspended in 25 ml of buffer in a heat-resistant tube. The solution was placed in a water bath and heated to 100°C for 15 min to kill all of the bacteria, then stored at −20°C. Prior to experiments, the S. aureus cells were defrosted and diluted in reaction buffer to an OD595 of 0.8–0.9. Next, 900 µl of the prepared solution was transferred into 24-well plates, and 100 µl of P. aeruginosa extracellular fractions, both untreated and treated with BLT, were added. The plates were placed immediately in an EnVision plate reader. OD600 measurements were taken every 5 min during incubation (37°C, orbital shaking mode). The assay was performed for 3 independent experiments with one repetition per sample. The maximum absorbance value was set as 1, and all other measurements were normalized at a ratio of 1.
Elastase B (pseudolysin) activity
Pseudolysin activity was analyzed by performing an Elastin Congo Red assay. A reaction buffer containing 0.05 M Tris-HCl supplemented with 0.5 mM CaCl2 (Avantor Performance Materials, Poland) at pH 7.5 was prepared and sterilized. A total of 10 mg of Elastin Congo Red (Sigma-Aldrich, Germany) was transferred into an Eppendorf tube, and 900 µl of reaction buffer was added. Next, 100 µl of the analyzed P. aeruginosa extracellular fractions, both untreated and treated with BLT, were added, and the tubes were incubated horizontally at 37°C and 150 rpm for 3 and 24 hrs. The suspensions were centrifuged (10,000 rpm, 10 min, and 4°C), and 200 µl of each sample was transferred into 96-well plates. The released Congo Red was quantified by measuring the absorbance at 495 nm, which was indicative of elastase B activity.Citation72,73 Staphylolysin also exhibited a certain degree of activity in this assay, which enhanced the elastinolytic activity of pseudolysin by several fold, indicating synergistic action by staphylolysin and pseudolysin.Citation74 However, because the increased absorbance at 495 nm resulted from the liberation of soluble dye fragments due to pseudolysin action, this assay only measured elastase B activity.Citation72 The assay was performed for 3 independent experiments with one repetition per sample. The detection limit was 4.0 μg of elastase per mg of total protein.Citation75
Proteolytic activity
To analyze the overall proteolytic activity of the bacteria, skim milk agar plates were employed as previously described.Citation72 A regular LB 1.5% agar solution was sterilized, cooled to 50°C and then supplemented with 1.25% (w/v) sterile skim milk. A total of 20 ml of the solution was poured aseptically into a Petri dish to solidify at room temperature. A total of 2 µl of each of the analyzed cultures, both untreated and treated with a sub-lethal dose of BLT, were transferred onto the agar plates and incubated for 18 hrs at 37°C. The clear zones around the colonies were indicative of total proteolytic activity. For lethal BLT, total secreted proteases activity was determined using a modified skim milk assay based on the turbidity changes in the milk during protease incubation.Citation70 A total of 900 µl of 1.5% skim milk was transferred into a transparent 24-well plate. Next, 100 µl of the prepared supernatants were added, and the plate was analyzed using an EnVision plate reader. Finally, OD600 measurements were taken every 5 min during incubation (37°C and 200 rpm). The assay was performed for 3 independent experiments with one repetition per sample.
Lipolytic activity
Lipolytic activity was analyzed using egg yolk agar plates according to a previously described method.Citation76 To prepare the egg yolk emulsion, chicken eggs were washed carefully and left in 70% ethanol for 5 min. The shells were crushed, the albumen was separated, and the yolks were transferred into a sterile graduated cylinder and mixed with ddH2O at a ratio of 2:3. LB supplemented with agar (15 g/l) was autoclaved and cooled to 50°C. The medium was mixed thoroughly with a freshly prepared egg yolk emulsion (10% v/v). A total of 20 ml of solution was poured into appropriate plates to solidify for at least 20 min. A total of 2 µl of the analyzed P. aeruginosa cultures, both untreated and treated with BLT, were transferred onto the agar plates and incubated for 24 and 48 hrs at 37°C. After 24 hrs, a white precipitation halo around the colonies was observed as the result of phospholipase and lipase activity. The assay was performed for 3 independent experiments with one repetition per sample.
Motility assays
Swimming
Swimming motility assays were performed with M8 agar plates supplemented with glucose, casamino acids, and MgSO4 (Avantor Performance Materials, Poland) following a previously described method with modifications.Citation77 Five-fold concentrated M8 medium (30 g/l Na2HPO4; 15 g/l KH2PO4; and 2.5 g/l NaCl) was autoclaved. A 2% glucose solution, a 2% casamino acids solution and a 1 M MgSO4 solution were filtered separately using 0.22-µm syringe filters. Next, 3 g of agar was mixed with 765 ml of water, autoclaved and cooled to 50°C. Then, 200 ml of 5x M8 solution, 10 ml of 2% glucose, 25 ml of 2% casamino acids and 1 ml of 1 M MgSO4 solution were thoroughly mixed. A total of 25 ml of the prepared medium was poured into appropriate plates to solidify and dry for 2 hrs. The tips of sterile toothpicks were immersed in individual analyzed bacterial cultures, both untreated and treated with BLT, and then inserted into a swimming agar plate (avoiding piercing the agar completely down to the bottom of the Petri dish). The plates were incubated with the lids up for 18 hrs at 37°C, and bacterial growth zones were measured.
Swarming
Swarming motility assays were performed with M8 agar plates supplemented with glucose, casamino acids, and MgSO4 following a previously described method with modifications.Citation78 The 5x M8 medium previously utilized to analyze swimming motility was autoclaved. A 10% glucose solution, a 10% casamino acids solution and a 1 M MgSO4 solution were filtered separately through 0.22-µm syringe filters. Next, 8 g of agar was mixed with 765 ml of water, autoclaved and cooled to 50°C. Then, 200 ml of 5x M8 solution, 10 ml of 10% glucose, 25 ml of 10% casamino acids and 1 ml of 1 M MgSO4 solution were mixed thoroughly. A total of 25 ml of the prepared medium was poured into appropriate plates to solidify and dry for 2 hrs. A total of 2 µl of the analyzed bacterial cultures, both untreated and treated with BLT, were transferred onto the agar plates. The plates were incubated with the lids up for 18 hrs at 37°C, and bacterial growth zones were measured.
Twitching
Twitching motility was analyzed on LB agar plates according to a previously described method.Citation79 LB medium at 20 g/l and agar at 10 g/l were mixed and autoclaved. Ten milliliters of the prepared solution was poured into appropriate plates to solidify and dry for 24 hrs. The tips of sterile toothpicks were immersed in individual analyzed bacterial cultures, both untreated and treated with BLT, and inserted deep into the twitching agar plates to reach the bottom. The plates were placed with lids down in a humidifying chamber and incubated for 18 hrs at 37°C. To visualize the results, a TM solution (50% v/v methanol and 10% v/v glacial acetic acid) (Avantor Performance Materials, Poland) was dropped on top of the agar plate and incubated for at least 5 min. Then, the TM solution was carefully washed out, and bacterial growth zones were measured.
Each motility assay was performed for 3 independent experiments with one repetition per sample.
Checkerboard analysis of the interaction between gentamycin, ceftazidime, meropenem and blue light irradiation
To evaluate potential synergistic cooperation between antimicrobials and BLT, we analyzed various combinations of antibiotics and BLT using a checkerboard titration method.Citation80 We combined different concentrations of antimicrobials with a range of sub-lethal doses of blue light irradiation (the antibiotic was added to the P. aeruginosa culture after the BLT treatment). The plates were incubated overnight at 37°C in the dark. After incubation, OD600 values were measured to determine MIC changes. All measurements were carried out 3 times (3 independent experiments).
Eukaryotic cell culture
A human keratinocyte (HaCaT) cell line (purchased from Cell Line Services Eppelheim, Germany) was cultured in Dulbecco's modified Eagle's medium (DMEM) supplemented with 10% fetal bovine serum, 2 mM glutamine, 100 units/ml penicillin and 100 μg/ml streptomycin. The cultures were maintained in a humidified atmosphere containing 5% CO2 at 37°C.
Cytotoxicity assay with extracellular fractions
P. aeruginosa strain supernatants were collected as previously described. Keratinocytes (3 × 104) in a 200-μl volume were seeded into 96-well plates and allowed to adhere overnight. Bacterial cell supernatants (both illuminated and kept in dark), grown for 48 hrs and filtered, were then added to the medium in a volume of 10 µl, and cells were incubated for a further 24 hrs. Cell survival was determined by performing an MTT (3-(4,5-dimethylthiazol-2-yl)-2,5-diphenyltetrazolium bromide) assay. Briefly, MTT (Sigma-Aldrich, Germany) was added to a final concentration of 0.5 mg/ml and incubated for 3 hrs at 37°C. Cells were lysed with DMSO (Sigma-Aldrich, Germany) and the absorbance of the formazan solution was measured at 550 nm with a plate reader (Victor, 1420 multilabel counter). All measurements were carried out 3 times (3 independent experiments with 3 repetitions for each sample).
Statistical methods
Statistical analyses were performed using the statistical suite STATISTICA (data analysis software system) version 10.0 (StatSoft. Inc. (2011), www.statsoft.com) and Excel. Quantitative variables were characterized by the arithmetic mean of standard deviation. To test the homogeneity of the variances, the Leven (Brown-Forsythe) test was utilized. The statistical significance of differences between 2 groups was determined with Student's t-test. The significance level was P < 0.05.
Disclosure of potential conflicts of interest
No potential conflicts of interest were disclosed.
Author contributions
GF did the experimental work, participated in conception of the study and has been involved in drafting manuscript. AK performed studies concerning eukaryotic cells. MG participated in the experimental work, has been involved in the coordination, conception, and design of the study and wrote the manuscript. All of the authors have read and approved the final manuscript.
KVIR_S_1250995.zip
Download Zip (2.3 MB)Acknowledgments
The isogenic pairs of isolates with affected phenazines production were kindly provided by Lars Dietrich from Department of Biological Sciences Columbia University New York. P. aeruginosa strains with disrupted movement systems were provided by Joanne N. Engel from Department of Medicine, University of California San Francisco, United States of America. Wild-type strain PAK was kindly provided by Reuben Ramphal from Institut Pasteur Paris, France
Funding
The studies investigating blue light treatment of P. aeruginosa and its synergy with antimicrobials were supported by grant no. 2015/19/B/NZ7/02487 from the National Science Center (M.G.). Publication costs were covered by the EU FP7 project MOBI4Health, GA. 316094. The funders had no role in study design, data collection and analysis, decision to publish, or preparation of the manuscript.
Reference
- Balasubramanian D, Schneper L, Kumari H, Mathee K. A dynamic and intricate regulatory network determines Pseudomonas aeruginosa virulence. Nucleic Acids Res 2013; 41:1-20; PMID:23143271; http://dx.doi.org/10.1093/nar/gks1039
- Rybtke M, Hultqvist LD, Givskov M, Tolker-Nielsen T. Pseudomonas aeruginosa Biofilm Infections: Community Structure, Antimicrobial Tolerance and Immune Response. J Mol Biol 2015; 427:3628-45; PMID:26319792; http://dx.doi.org/10.1016/j.jmb.2015.08.016
- Serra R, Grande R, Butrico L, Rossi A, Settimio UF, Caroleo B, Amato B, Gallelli L, de FS. Chronic wound infections: the role of Pseudomonas aeruginosa and Staphylococcus aureus. Expert Rev Anti Infect Ther 2015; 13:605-13; PMID:25746414; http://dx.doi.org/10.1586/14787210.2015.1023291
- Mayaud C, Parrot A, Cadranel J. Pyogenic bacterial lower respiratory tract infection in human immunodeficiency virus-infected patients. Eur Respir J Suppl 2002; 36:28s-39s; PMID:12168745; http://dx.doi.org/10.1183/09031936.02.00400602
- Vento S, Cainelli F, Temesgen Z. Lung infections after cancer chemotherapy. Lancet Oncol 2008; 9:982-92; PMID:19071255; http://dx.doi.org/10.1016/S1470-2045(08)70255-9
- Potron A, Poirel L, Nordmann P. Emerging broad-spectrum resistance in Pseudomonas aeruginosa and Acinetobacter baumannii: Mechanisms and epidemiology. Int J Antimicrob Agents 2015; 45:568-85; PMID:25857949; http://dx.doi.org/10.1016/j.ijantimicag.2015.03.001
- Clatworthy AE, Pierson E, Hung DT. Targeting virulence: a new paradigm for antimicrobial therapy. Nat Chem Biol 2007; 3:541-8; PMID:17710100; http://dx.doi.org/10.1038/nchembio.2007.24
- Merrell DS, Falkow S. Frontal and stealth attack strategies in microbial pathogenesis. Nature 2004; 430:250-6; PMID:15241423; http://dx.doi.org/10.1038/nature02760
- Sommer R, Joachim I, Wagner S, Titz A. New approaches to control infections: anti-biofilm strategies against gram-negative bacteria. Chimia (Aarau) 2013; 67:286-90; PMID:23967708; http://dx.doi.org/10.2533/chimia.2013.286
- Rasmussen TB, Givskov M. Quorum-sensing inhibitors as anti-pathogenic drugs. Int J Med Microbiol 2006; 296:149-61; PMID:16503194; http://dx.doi.org/10.1016/j.ijmm.2006.02.005
- Wagner S, Sommer R, Hinsberger S, Lu C, Hartmann RW, Empting M, Titz A. Novel Strategies for the Treatment of Pseudomonas aeruginosa Infections. J Med Chem 2016; 59:5929-69; PMID:26804741; http://dx.doi.org/10.1021/acs.jmedchem.5b01698
- Kuang Z, Hao Y, Walling BE, Jeffries JL, Ohman DE, Lau GW. Pseudomonas aeruginosa elastase provides an escape from phagocytosis by degrading the pulmonary surfactant protein-A. PLoS One 2011; 6:e27091; PMID:22069491; http://dx.doi.org/10.1371/journal.pone.0027091
- Caballero AR, Moreau JM, Engel LS, Marquart ME, Hill JM, O'Callaghan RJ. Pseudomonas aeruginosa protease IV enzyme assays and comparison to other Pseudomonas proteases. Anal Biochem 2001; 290:330-7; PMID:11237336; http://dx.doi.org/10.1006/abio.2001.4999
- Berka RM, Vasil ML. Phospholipase C (heat-labile hemolysin) of Pseudomonas aeruginosa: purification and preliminary characterization. J Bacteriol 1982; 152:239-45; PMID:6811552
- Rossignol G, Merieau A, Guerillon J, Veron W, Lesouhaitier O, Feuilloley MG, Orange N. Involvement of a phospholipase C in the hemolytic activity of a clinical strain of Pseudomonas fluorescens. BMC Microbiol 2008; 8:189; PMID:18973676; http://dx.doi.org/10.1186/1471-2180-8-189
- Bush K, Courvalin P, Dantas G, Davies J, Eisenstein B, Huovinen P, Jacoby GA, Kishony R, Kreiswirth BN, Kutter E, et al. Tackling antibiotic resistance. Nat Rev Microbiol 2011; 9:894-6; PMID:22048738; http://dx.doi.org/10.1038/nrmicro2693
- Dai T, Tegos GP, Zhiyentayev T, Mylonakis E, Hamblin MR. Photodynamic therapy for methicillin-resistant Staphylococcus aureus infection in a mouse skin abrasion model. Lasers Surg Med 2010; 42:38-44; PMID:20077489; http://dx.doi.org/10.1002/lsm.20887
- Dai T, Tegos GP, Lu Z, Huang L, Zhiyentayev T, Franklin MJ, Baer DG, Hamblin MR. Photodynamic therapy for Acinetobacter baumannii burn infections in mice. Antimicrob Agents Chemother 2009; 53:3929-34; PMID:19564369; http://dx.doi.org/10.1128/AAC.00027-09
- Dai T, Garcia B, Murray CK, Vrahas MS, Hamblin MR. UVC light prophylaxis for cutaneous wound infections in mice. Antimicrob Agents Chemother 2012; 56:3841-8; PMID:22564833; http://dx.doi.org/10.1128/AAC.00161-12
- Dai T, Kharkwal GB, Zhao J, St Denis TG, Wu Q, Xia Y, Huang L, Sharma SK, d'Enfert C, Hamblin MR. Ultraviolet-C light for treatment of Candida albicans burn infection in mice. Photochem Photobiol 2011; 87:342-9; PMID:21208209; http://dx.doi.org/10.1111/j.1751-1097.2011.00886.x
- Waldman G. Introduction to light : the physics of light, vision, and color. 193. Mineola: Dover Publications, 2002
- Grinholc M, Rapacka-Zdonczyk A, Rybak B, Szabados F, Bielawski KP. Multiresistant Strains Are as Susceptible to Photodynamic Inactivation as Their Naive Counterparts: Protoporphyrin IX-Mediated Photoinactivation Reveals Differences Between Methicillin-Resistant and Methicillin-Sensitive Staphylococcus aureus Strains. Photomed Laser Surg 2014; 32:121-9; PMID:24527879; http://dx.doi.org/10.1089/pho.2013.3663
- Dai T, Vrahas MS, Murray CK, Hamblin MR. Ultraviolet C irradiation: an alternative antimicrobial approach to localized infections? Expert Rev Anti Infect Ther 2012; 10:185-95; PMID:22339192; http://dx.doi.org/10.1586/eri.11.166
- Dai T, Gupta A, Huang YY, Yin R, Murray CK, Vrahas MS, Sherwood ME, Tegos GP, Hamblin MR. Blue light rescues mice from potentially fatal Pseudomonas aeruginosa burn infection: efficacy, safety, and mechanism of action. Antimicrob Agents Chemother 2013; 57:1238-45; PMID:23262998; http://dx.doi.org/10.1128/AAC.01652-12
- Enwemeka CS, Williams D, Hollosi S, Yens D, Enwemeka SK. Visible 405 nm SLD light photo-destroys methicillin-resistant Staphylococcus aureus (MRSA) in vitro. Lasers Surg Med 2008; 40:734-7; PMID:19065556; http://dx.doi.org/10.1002/lsm.20724
- Wheeland RG, Dhawan S. Evaluation of self-treatment of mild-to-moderate facial acne with a blue light treatment system. J Drugs Dermatol 2011; 10:596-602; PMID:21637900
- Halstead FD, Thwaite JE, Burt R, Laws TR, Raguse M, Moeller R, Webber MA, Oppenheim BA. The antibacterial activity of blue light against nosocomial wound pathogens growing planktonically and as mature biofilms. Appl Environ Microbiol 2016; PMID:27129967
- Dai T, Gupta A, Murray CK, Vrahas MS, Tegos GP, Hamblin MR. Blue light for infectious diseases: Propionibacterium acnes, Helicobacter pylori, and beyond? Drug Resist Updat 2012; 15:223-36; PMID:22846406; http://dx.doi.org/10.1016/j.drup.2012.07.001
- Grinholc M, Rodziewicz A, Forys K, Rapacka-Zdonczyk A, Kawiak A, Domachowska A, Golunski G, Wolz C, Mesak L, Becker K, et al. Fine-tuning recA expression in Staphylococcus aureus for antimicrobial photoinactivation: importance of photo-induced DNA damage in the photoinactivation mechanism. Appl Microbiol Biotechnol 2015; 99:9161-76; PMID:26252968; http://dx.doi.org/10.1007/s00253-015-6863-z
- Dadras S, Mohajerani E, Eftekhar F, Hosseini M. Different photoresponses of Staphylococcus aureus and Pseudomonas aeruginosa to 514, 532, and 633 nm low level lasers in vitro. Curr Microbiol 2006; 53:282-6; PMID:16941244; http://dx.doi.org/10.1007/s00284-005-0490-3
- Kim S, Kim J, Lim W, Jeon S, Kim O, Koh JT, Kim CS, Choi H, Kim O. In vitro bactericidal effects of 625, 525, and 425 nm wavelength (red, green, and blue) light-emitting diode irradiation. Photomed Laser Surg 2013; 31:554-62; PMID:24138193; http://dx.doi.org/10.1089/pho.2012.3343
- Cieplik F, Spath A, Leibl C, Gollmer A, Regensburger J, Tabenski L, Hiller KA, Maisch T, Schmalz G. Blue light kills Aggregatibacter actinomycetemcomitans due to its endogenous photosensitizers. Clin Oral Investig 2014; 18:1763-9; PMID:24297656; http://dx.doi.org/10.1007/s00784-013-1151-8
- Soukos NS, Som S, Abernethy AD, Ruggiero K, Dunham J, Lee C, Doukas AG, Goodson JM. Phototargeting oral black-pigmented bacteria. Antimicrob Agents Chemother 2005; 49:1391-6; PMID:15793117; http://dx.doi.org/10.1128/AAC.49.4.1391-1396.2005
- Maclean M, McKenzie K, Anderson JG, Gettinby G, MacGregor SJ. 405 nm light technology for the inactivation of pathogens and its potential role for environmental disinfection and infection control. J Hosp Infect 2014; 88:1-11; PMID:25066049; http://dx.doi.org/10.1016/j.jhin.2014.06.004
- Kleinpenning MM, Smits T, Frunt MH, van Erp PE, van de Kerkhof PC, Gerritsen RM. Clinical and histological effects of blue light on normal skin. Photodermatol Photoimmunol Photomed 2010; 26:16-21; PMID:20070834; http://dx.doi.org/10.1111/j.1600-0781.2009.00474.x
- Amin RM, Bhayana B, Hamblin MR, Dai T. Antimicrobial blue light inactivation of Pseudomonas aeruginosa by photo-excitation of endogenous porphyrins: In vitro and in vivo studies. Lasers Surg Med 2016; PMID:26891084
- Dodd CER, Sharman RL, Bloomfield SF, Booth IR, Stewart GSAB. Inimical processes: Bacterial self-destruction and sub-lethal injury. Trends Food Sci Technol 1997; 8:238-41; http://dx.doi.org/10.1016/S0924-2244(97)01043-1
- ASM. Antimicrobial Agents and Chemotherapy, Instructions to Authors. http://aac.asm.org/site/misc/2016AprilAACITA.pdf. 2016
- Lau GW, Ran H, Kong F, Hassett DJ, Mavrodi D. Pseudomonas aeruginosa pyocyanin is critical for lung infection in mice. Infect Immun 2004; 72:4275-8; PMID:15213173; http://dx.doi.org/10.1128/IAI.72.7.4275-4278.2004
- Reszka KJ, Bilski PJ, Britigan BE. Quenching of singlet oxygen by pyocyanin and related phenazines. Photochem Photobiol 2010; 86:742-6; PMID:20408986; http://dx.doi.org/10.1111/j.1751-1097.2010.00728.x
- Hawdon NA, Aval PS, Barnes RJ, Gravelle SK, Rosengren J, Khan S, Ciofu O, Johansen HK, Hoiby N, Ulanova M. Cellular responses of A549 alveolar epithelial cells to serially collected Pseudomonas aeruginosa from cystic fibrosis patients at different stages of pulmonary infection. FEMS Immunol Med Microbiol 2010; 59:207-20; PMID:20528926; http://dx.doi.org/10.1111/j.1574-695X.2010.00693.x
- Griese M, Kappler M, Eismann C, Ballmann M, Junge S, Rietschel E, van Koningsbruggen-Rietschel S, Staab D, Rolinck-Werninghaus C, Mellies U, et al. Inhalation treatment with glutathione in patients with cystic fibrosis. A randomized clinical trial. Am J Respir Crit Care Med 2013; 188:83-9; PMID:23631796; http://dx.doi.org/10.1164/rccm.201303-0427OC
- Kipnis E, Sawa T, Wiener-Kronish J. Targeting mechanisms of Pseudomonas aeruginosa pathogenesis. Med Mal Infect 2006; 36:78-91; PMID:16427231; http://dx.doi.org/10.1016/j.medmal.2005.10.007
- Lau GW, Hassett DJ, Ran H, Kong F. The role of pyocyanin in Pseudomonas aeruginosa infection. Trends Mol Med 2004; 10:599-606; PMID:15567330; http://dx.doi.org/10.1016/j.molmed.2004.10.002
- Das T, Kutty SK, Kumar N, Manefield M. Pyocyanin facilitates extracellular DNA binding to Pseudomonas aeruginosa influencing cell surface properties and aggregation. PLoS One 2013; 8:e58299; PMID:23505483; http://dx.doi.org/10.1371/journal.pone.0058299
- Das MC, Sandhu P, Gupta P, Rudrapaul P, De UC, Tribedi P, Akhter Y, Bhattacharjee S. Attenuation of Pseudomonas aeruginosa biofilm formation by Vitexin: A combinatorial study with azithromycin and gentamicin. Sci Rep 2016; 6:23347; PMID:27000525; http://dx.doi.org/10.1038/srep23347
- Comolli JC, Waite LL, Mostov KE, Engel JN. Pili binding to asialo-GM1 on epithelial cells can mediate cytotoxicity or bacterial internalization by Pseudomonas aeruginosa. Infect Immun 1999; 67:3207-14; PMID:10377092
- de BS, Roger P, Dupuit F, Bajolet-Laudinat O, Fuchey C, Plotkowski MC, Puchelle E. Asialo GM1 is a receptor for Pseudomonas aeruginosa adherence to regenerating respiratory epithelial cells. Infect Immun 1996; 64:1582-8; PMID:8613364
- Rio-Alvarez I, Rodriguez-Herva JJ, Martinez PM, Gonzalez-Melendi P, Garcia-Casado G, Rodriguez-Palenzuela P, Lopez-Solanilla E. Light regulates motility, attachment and virulence in the plant pathogen Pseudomonas syringae pv tomato DC3000. Environ Microbiol 2014; 16:2072-85; PMID:24033935; http://dx.doi.org/10.1111/1462-2920.12240
- Komerik N, Wilson M, Poole S. The effect of photodynamic action on two virulence factors of gram-negative bacteria. Photochem Photobiol 2000; 72:676-80; PMID:11107854; http://dx.doi.org/10.1562/0031-8655(2000)072%3c0676:TEOPAO%3e2.0.CO;2
- Sharma M, Bansal H, Gupta PK. Virulence of Pseudomonas aeruginosa cells surviving photodynamic treatment with toluidine blue. Curr Microbiol 2005; 50:277-80; PMID:15886909; http://dx.doi.org/10.1007/s00284-005-4473-1
- Tubby S, Wilson M, Nair SP. Inactivation of staphylococcal virulence factors using a light-activated antimicrobial agent. BMC Microbiol 2009; 9:211; PMID:19804627; http://dx.doi.org/10.1186/1471-2180-9-211
- Bartolomeu M, Rocha S, Cunha A, Neves MG, Faustino MA, Almeida A. Effect of Photodynamic Therapy on the Virulence Factors of Staphylococcus aureus. Front Microbiol 2016; 7:267; PMID:27014198; http://dx.doi.org/10.3389/fmicb.2016.00267
- Kato IT, Prates RA, Sabino CP, Fuchs BB, Tegos GP, Mylonakis E, Hamblin MR, Ribeiro MS. Antimicrobial photodynamic inactivation inhibits Candida albicans virulence factors and reduces in vivo pathogenicity. Antimicrob Agents Chemother 2013; 57:445-51; PMID:23129051; http://dx.doi.org/10.1128/AAC.01451-12
- Kadurugamuwa JL, Beveridge TJ. Virulence factors are released from Pseudomonas aeruginosa in association with membrane vesicles during normal growth and exposure to gentamicin: a novel mechanism of enzyme secretion. J Bacteriol 1995; 177:3998-4008; PMID:7608073
- Kadurugamuwa JL, Beveridge TJ. Natural release of virulence factors in membrane vesicles by Pseudomonas aeruginosa and the effect of aminoglycoside antibiotics on their release. J Antimicrob Chemother 1997; 40:615-21; PMID:9421308; http://dx.doi.org/10.1093/jac/40.5.615
- Crosby HA, Bion JF, Penn CW, Elliott TS. Antibiotic-induced release of endotoxin from bacteria in vitro. J Med Microbiol 1994; 40:23-30; PMID:8289210; http://dx.doi.org/10.1099/00222615-40-1-23
- Prins JM, van Deventer SJ, Kuijper EJ, Speelman P. Clinical relevance of antibiotic-induced endotoxin release. Antimicrob Agents Chemother 1994; 38:1211-8; PMID:8092816; http://dx.doi.org/10.1128/AAC.38.6.1211
- Cassidy CM, Donnelly RF, Elborn JS, Magee ND, Tunney MM. Photodynamic Antimicrobial Chemotherapy (PACT) in combination with antibiotics for treatment of Burkholderia cepacia complex infection. J Photochem Photobiol B 2012; 106:95-100; PMID:22079165; http://dx.doi.org/10.1016/j.jphotobiol.2011.10.010
- Dastgheyb SS, Eckmann DM, Composto RJ, Hickok NJ. Photo-activated porphyrin in combination with antibiotics: therapies against Staphylococci. J Photochem Photobiol B 2013; 129:27-35; PMID:24148969; http://dx.doi.org/10.1016/j.jphotobiol.2013.09.006
- Ronqui MR, de Aguiar Coletti TM, de Freitas LM, Miranda ET, Fontana CR. Synergistic antimicrobial effect of photodynamic therapy and ciprofloxacin. J Photochem Photobiol B 2016; 158:122-9; PMID:26971277; http://dx.doi.org/10.1016/j.jphotobiol.2016.02.036
- Chibebe JJ, Fuchs BB, Sabino CP, Junqueira JC, Jorge AO, Ribeiro MS, Gilmore MS, Rice LB, Tegos GP, Hamblin MR, et al. Photodynamic and antibiotic therapy impair the pathogenesis of Enterococcus faecium in a whole animal insect model. PLoS One 2013; 8:e55926; PMID:23457486; http://dx.doi.org/10.1371/journal.pone.0055926
- Magiorakos AP, Srinivasan A, Carey RB, Carmeli Y, Falagas ME, Giske CG, Harbarth S, Hindler JF, Kahlmeter G, Olsson-Liljequist B, et al. Multidrug-resistant, extensively drug-resistant and pandrug-resistant bacteria: an international expert proposal for interim standard definitions for acquired resistance. Clin Microbiol Infect 2012; 18:268-281; PMID:21793988; http://dx.doi.org/10.1111/j.1469-0691.2011.03570.x
- CLSI. Performance standards for antimicrobial susceptibility testing. CLSI approved standard M100-S22. Wayne, PA: Clinical and Laboratory Standards Institute, 2012
- Jett BD, Hatter KL, Huycke MM, Gilmore MS. Simplified agar plate method for quantifying viable bacteria. Biotechniques 1997; 23:648-50; PMID:9343684
- Barry AL, Lasner RA. In-vitro methods for determining minimal lethal concentrations of antimicrobial agents. Am J Clin Pathol 1979; 71:88-92; PMID:105628; http://dx.doi.org/10.1093/ajcp/71.1.88
- Kohanski MA, DePristo MA, Collins JJ. Sublethal antibiotic treatment leads to multidrug resistance via radical-induced mutagenesis. Mol Cell 2010; 37:311-20; PMID:20159551; http://dx.doi.org/10.1016/j.molcel.2010.01.003
- Latimer J, Forbes S, McBain AJ. Attenuated virulence and biofilm formation in Staphylococcus aureus following sublethal exposure to triclosan. Antimicrob Agents Chemother 2012; 56:3092-100; PMID:22430975; http://dx.doi.org/10.1128/AAC.05904-11
- Andersson DI, Hughes D. Microbiological effects of sublethal levels of antibiotics. Nat Rev Microbiol 2014; 12:465-78; PMID:24861036; http://dx.doi.org/10.1038/nrmicro3270
- El-Mowafy SA, bd El Galil KH, El-Messery SM, Shaaban MI. Aspirin is an efficient inhibitor of quorum sensing, virulence and toxins in Pseudomonas aeruginosa. Microb Pathog 2014; 74:25-32; PMID:25088031; http://dx.doi.org/10.1016/j.micpath.2014.07.008
- Alatraktchi FA, Andersen SB, Johansen HK, Molin S, Svendsen WE. Fast Selective Detection of Pyocyanin Using Cyclic Voltammetry. Sensors (Basel) 2016; 16; PMID:27007376; http://dx.doi.org/10.3390/s16030408
- Kessler E, Safrin M. Elastinolytic and proteolytic enzymes. Filloux A, and Ramos J. L. 135-69. 2014. Humana Press. Pseudomonas. Methods and Protocols
- Ohman DE, Cryz SJ, Iglewski BH. Isolation and characterization of Pseudomonas aeruginosa PAO mutant that produces altered elastase. J Bacteriol 1980; 142:836-42; PMID:6769912
- Kessler E, Safrin M, Abrams WR, Rosenbloom J, Ohman DE. Inhibitors and specificity of Pseudomonas aeruginosa LasA. J Biol Chem 1997; 272:9884-9; PMID:9092525; http://dx.doi.org/10.1074/jbc.272.15.9884
- Hamood AN, Griswold J, Colmer J. Characterization of elastase-deficient clinical isolates of Pseudomonas aeruginosa. Infect Immun 1996; 64:3154-60; PMID:8757847
- Kashid SG, Ghosh JS. Production, Isolation and Characterization of Exotoxin Produced by Bacillus cereus NCIM-2156 and Bacillus licheniformis NCIM-5343. Br J Pharmacol Toxicol 2010; 1:50-5
- Ha D, Kuchma SL, O'Toole GA. Plate-Based assay for swimming motility in Pseudomonas aeruginosa. Filloux A, and Ramos J. L. 59-65. 2014. Humana Press. Pseudomonas. Methods and Protocols
- Ha D, Kuchma SL, O'Toole GA. Plate-Based assay for swarming motility in Pseudomonas aeruginosa. Filloux A, and Ramos J. L. 67-72. 2014. Humana Press. Pseudomonas. Methods and Protocols
- Turnbull L, Whitchurch CB. Motility assay: twitching motility. Filloux A, and Ramos J. L. 73-86. 2014. Humana Press. Pseudomonas. Methods and Protocols
- Thornsberry C. Antimicrobial susceptibility testing of anaerobic bacteria: review, comments, and opinions. Ann Otol Rhinol Laryngol Suppl 1991; 154:7-10; PMID:1952686
- Cumming G, Fidler F, Vaux DL. Error bars in experimental biology. J Cell Biol 2007; 177:7-11; PMID:17420288; http://dx.doi.org/10.1083/jcb.200611141
- Bellin DL, Sakhtah H, Rosenstein JK, Levine PM, Thimot J, Emmett K, Dietrich LE, Shepard KL. Integrated circuit-based electrochemical sensor for spatially resolved detection of redox-active metabolites in biofilms. Nat Commun 2014; 5:3256; PMID:24510163; http://dx.doi.org/10.1038/ncomms4256
- Bucior I, Pielage JF, Engel JN. Pseudomonas aeruginosa pili and flagella mediate distinct binding and signaling events at the apical and basolateral surface of airway epithelium. PLoS Pathog 2012; 8:e1002616; PMID:22496644; http://dx.doi.org/10.1371/journal.ppat.1002616
- Ramphal R, Balloy V, Jyot J, Verma A, Si-Tahar M, Chignard M. Control of Pseudomonas aeruginosa in the lung requires the recognition of either lipopolysaccharide or flagellin. J Immunol 2008; 181:586-92; PMID:18566425; http://dx.doi.org/10.4049/jimmunol.181.1.586