The larvae of the greater wax moth, Galleria mellonella (Lepidoptera: Pyralidae) has been used as a host and model system for many fungal and bacterial pathogens due to its reduced innate immune system [Citation1] and ease of handling and rearing. Organisms of interest can be injected into the haemolymph of Galleria where they can be maintained for up to 10 days in vivo and then recovered for purification or enumeration. However, in most virulence studies involving G. mellonella, pathogens are injected to determine their LD50 and/or to monitor production of antimicrobial peptides (AMPs) such as defensin and lysozyme [Citation2,Citation3]. Larval health after infection with various agents is evaluated by activity, cocoon formation, melanization and survival rate [Citation4].
The insect immune system is relatively more advanced than other invertebrates such as nematodes and thus can give more insights about mammalian infection processes [Citation5]. The G. mellonella immune system consists of the cellular and the humoral response [Citation1]. The cellular response to invading pathogens is a direct response by haemocytes which surround and immobilize them by phagocytosis [Citation2]. The humoral response consists of melanization, and production of opsonins and AMPs. The opsonin apolipophorin-III, which shows high affinity for bacterial lipopolysaccharide in insects, has high homology with mammalian apolipoprotein [Citation1]. G. mellonella haemolymph contains antimicrobial peptides (gallerimycin and galiomicin) which act to break down the cell walls of fungal or bacterial pathogens [Citation2]. Melanization synthesis and deposition serves to encapsulate pathogens at the wound site in insects [Citation1].
G. mellonella has been used as a host model to study a variety of bacteria including Gram-positive bacteria such as Enterococus faecalis, Enterococcus faecium, Staphylococcus aureus, Streptococcus pyogenes, S. pneumonia, and Listeria monocytogenes; and Gram-negative bacteria such as Escherichia coli, Pseudomonas aeruginosa, and Klebsiella pneumonia [Citation1]. In addition, G. mellonella larvae have been used to study several pathogenic fungi such as Candida albicans and Aspergillus fumigatus [Citation6] and as a model host for some trans-kingdom pathogens such as Fusarium oxysporum and Pseudomonas aeruginosa [Citation7]. The G. mellonella model was also used to assess the efficacy of bacteriophage therapy to treat pathogenic infections [Citation8].
Its readiness to infection and its ability to initiate a defense response makes G. mellonella an excellent infection model [Citation9]. Although many studies have been conducted to investigate the components of G. mellonella innate immune response system, the haemolymph of this interesting model has not been well investigated. To date, there are very few reports of the chemical composition of the primary and secondary metabolites of the haemolymph of wax worm [Citation10–13] and even fewer utilizing modern GC-MS techniques. A study of the changes in amino acid (AA) composition of the haemolymph in response to temperature acclimation found 14 different amino acids by liquid chromatography and showed an increase in AA content with decreasing temperatures [Citation13]. Earlier works focused on the lipid and lipoprotein fractions of the haemolymph by solvent extraction followed by GC-FID, and by centrifugation followed by thin layer chromatography [Citation12,Citation14], respectively. However, to our knowledge, no general characterization of the polar metabolites of G. mellonella haemolymph has been carried out which would help define its ability to host a wide range of fungal and bacterial pathogenic species.
Untargeted metabolomic profiling is extremely useful for simultaneous analysis of a broad range of metabolites within a cell type, tissue, or even whole organisms, including amino acids, organic acids, sugars, sugar alcohols and fatty acids. Analysis of small molecules which are often metabolic pathway intermediates can help identify subtle changes in complex metabolic systems and can be used comparatively. These studies can be achieved through a variety of analytical tools including liquid and gas chromatography, as well as by using different chemical derivatization techniques. Each method opens a small window of understanding into a particular metabolic pathway or the biochemistry underlying a particular condition or treatment effect. In the case of this study, we used three methods of derivatization 1) trimethylsilylation (TMS); 2) methyl chloroformate (MCF); and 3) boron trifluoride (BF3) to detect several classes of metabolites found in the haemolymph of G. mellonella for the purpose of ascertaining why it is useful as an infectious disease host.
Galleria mellonella larvae (waxworms) were received in sawdust, from Bassett's Cricket Ranch (Visalia, CA). Waxworm larvae were fed overnight on a mixture of wheat bran, honey and water, to reverse any dehydration and starvation effects from shipping. The following day, haemolymph was collected (A). The haemolymph was subject to the derivatization and GC-MS analyses (B). GC conditions and methods, derivatization, compound identification and quantification for insect haemolymph were performed as described in previously published protocols [Citation15].
Figure 1. Collection and chemical analyses of Galleria mellonella. A: Haemolymph was collected by first puncturing the larvae behind the first set of thoracic (true) legs, in the inter-spiracle space, with a 0.5 mL BD fine insulin syringe. For each replicate, haemolymph from five larvae was collected. From 5 to 7 µL of pure haemolymph was collected from each larvae using a 10 µL capillary tube and was ejected into a 0.2 ml tube containing 50 µL of solvent (8:1:1 methanol:chloroform:water) to prevent melanization. Portions from the replicates were derivatized using TMS, MCF, or BF3 prior to the GC-MS analysis. B: GC-MS total ion chromatogram of G. mellonela haemolymph after TMS derivatization. The numbers above the peaks refer to the numbers listed in Is: internal standard used to calculate the absolute amount of each compounds.
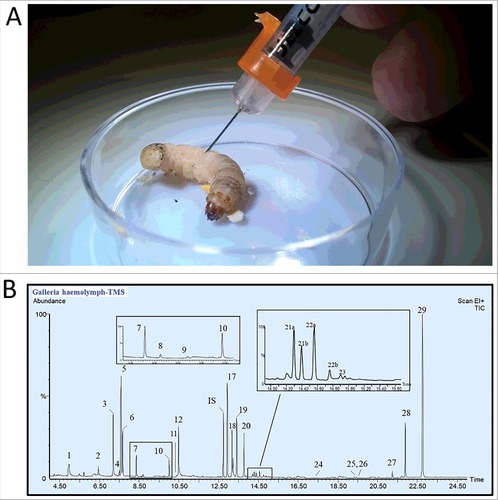
Twenty-nine and 31 metabolites were detected in the haemolymph of G. mellonella after chemical derivatization with TMS or MCF, respectively, followed by GC-MS analysis. and show the distribution of metabolites detected by the first two methods. Fatty acid results from the boron BF3 experiments were included in . The mean total metabolites concentration constituted 156.2 ± 74.3 mM by TMS and 108.9 ± 25.6 mM by MCF derivatization.
Table 1. Concentration (mM) of metabolites of Galleria mellonella haemolymph derivatized by trimethylsilylation (TMS) and analyzed by GC-MS. Means ± standard deviation shown were generated from total ion chromatograms (TIC) peaks from duplicate injections from five biological samples (n = 10). Peak areas were converted to mM concentration by use of linear calibration curves of standard reference compounds treated the same as samples.
Table 2. Concentration (mM) of metabolites of Galleria mellonella haemolymph derivatized by methyl chloroformate (MCF) and analyzed by GC-MS. Means ± standard deviation shown were generated from total ion chromatograms (TIC) peaks from duplicate injections from five biological samples (n = 10). Peak areas were converted to mM concentration by use of linear calibration curves of standard reference compounds treated the same as samples.
Several non-proteinogenic amino acids were detected in the haemolymph of G. mellonella. Putrescine (1, 4-butadiamine) was detected only by TMS and was found to be 88.7±41.8 mM, the highest of any compound in the haemolymph of G. mellonella. Putrescine has also been found in the haemolymph of the corn earworm, Heliothis zea, at 375±25 µg/mL haemolymph by ninhydrin assay and thin layer chromatography [Citation16]. The primary role of putrescine is binding of ammonia waste for transport prior to excretion, as was found in other arthropods [Citation17,Citation18]. Other non-proteinogenic amines detected by TMS included pyroglutamic acid and β-alanine (). These metabolites were not detected by MCF. Interestingly, gamma-aminobutyric acid (GABA) was detected by HPLC in larvae just before metamorphosing into adults [Citation13], but we did not detect it in any of our analyses. This may have been due to differences in the age or storage conditions of our larvae as we routinely detect GABA in other biological samples using TMS derivatization [Citation15].
Among the proteinogenic amino acids, proline was the major amino acid detected by both methods, TMS (15.2±9.4 mM) and MCF (32.5±4.4 mM). Proline has been implicated in hymenopterans as an alternate energy source for flight muscles [Citation19], but its role in waxworm larvae may be more related to muscle movements associated with the wandering stage. In tarantula spider haemolymph, proline was also found to be the major amino acid [Citation20]. Glycine and serine made up 3.1 and 2.2% of the haemolymph composition, respectively for TMS-derivatized samples, while these were 4.7 and 5.1% of the composition, respectively by MCF. Other amino acids found by both methods included alanine, valine, glutamic acid, asparagine, threonine, aspartic acid, phenylalanine and isoleucine ( & ). For TMS method, other amino acids detected at low concentration included alanine, valine, isoleucine, threonine, glutamic acid, phenylalanine, asparagine, and aspartic acid. These ranged in concentration from < 0.2 mM to 1.25 mM. For MCF method, several other amino acids were found in significant concentrations including lysine, histidine, and tyrosine (). Interestingly, Grace's insect medium includes 16 mM of histidine, but only lesser amounts of most other amino acids (between 0.5 and 5 mM) (Cat#11595, Thermofisher Scientific, Waltham, PA). Compounds detected at low concentration (<1 mM) by MCF included tryptophan, glutamic acid, threonine, aspartic acid, methionine and cysteine. These were not detected by TMS derivatization. Hanzal and Jegorov [Citation13] reported that glutamine, alanine and glycine were the most abundant amino acids of control G. mellonella larvae, and levels of phenylalanine and lysine increased during cold acclimation of wax worms. In the same study, levels of GABA and valine increased with larval age, while glycine, alanine and glutamine decreased with increasing age [Citation13].
Citric and malic acids were the only two organic acids detected in G. mellonella haemolymph by TMS and these were also found in our study of the haemolymph of the Asian citrus psyllid, D. citri [Citation15]. The concentrations of citric and malic acids were 5.0 and 1.5 mM, respectively. In addition to citric and malic acids, quinic acid, and succinic acid were detected by MCF at levels of 5.1 mM and 0.7 mM respectively. Fumaric and maleic acids were also found in trace amounts, and these participate in the citric acid cycle. Grace's insect medium supplies malic acid at 5 mM, and fumaric and succinic acids at a concentration of 0.5 mM indicating that they are required nutritional elements for insects.
Numerous saturated fatty acids (FAs) were detected by BF3 including tetradecanoic (myristic) acid, pentadecanoic acid, hexadecanoic (palmitic) acid, and octadecanoic (stearic) acid. Unsaturated FAs detected were oleic (C18:1) and linoleic (C18:2). However, the relative abundance of free fatty acids was low in our larvae (1.67 mM, 0.36%) compared to Thomas (1979) [Citation12] who found 9.3% of the whole haemolymph of G. mellonella was composed of fatty acids. The distribution of fatty acids was similar to that reported by Yendol (1970) [Citation14] except that we also found C14 and C15 saturated FAs, but did not find linoelaidic acid (C18:3) or palmitoleic (C16:1). Tarantula haemolymph was similar in its FA content, consisting mostly of C16 and C18 saturated FAs with traces of C14 and C20 FAs [Citation20]. FAs play a variety of roles in insect metabolism, especially in energy storage and demand [Citation21], immunity [Citation22], cell membrane structures [Citation23] and hormone biosynthesis [Citation12].
Trehalose, a glucose-glucose disaccharide, was the most abundant carbohydrate detected in waxworm haemolymph (), followed by the monosaccharides fructose and glucose. Trehalose constituted about 7% of the peak area while all other sugars were less than 1% each and did not exceed 9% of the overall haemolymph chemical composition. In several lepidopterans, including G. mellonella, trehalose was the major blood sugar, up to 90% [Citation11]. Assuming our unknown disaccharides are truly sugars, trehalose accounts for 79.7% of the sugars found in our wax worm larvae haemolymph by TMS/GC-MS. Trehalose is commonly found in the haemolymph of many insects and serves several physiological functions including carbon storage [Citation24], direct utilization for glucose energy metabolism [Citation11], as a cryo-protectant [Citation25,Citation26], and for lowering osmotic pressure since large amounts can be stored in the fat body without toxic effects [Citation27,Citation28]. Levels of trehalose can vary dramatically by species, and based on ambient temperatures, activity, growth stage, and nutrition [Citation28]. In aphids, for example, 196 mM and 926 mM trehalose were reported for the chestnut aphid, Lachnus tropicalis and Aphis gossypii respectively [Citation29]. Recently, structural roles for trehalose were defined after RNA interference of trehalase enzymes showed deformities in chitin synthesis in larva of Colorado potato beetles [Citation30].
We detected both inorganic and organic phosphate () in the haemolymph of G. mellonella. Inorganic phosphate was quite high, about 6.7 mM, while the sugar phosphates, including glycerophosphate and ethanolamine phosphate, made up 8.2% of the haemolymph composition. The early work of Wyatt et al. (1956) in haemolymph characterization showed that inorganic phosphate found in the haemolymphs of Bombyx mori and G. mellonella ranged from 5 to 15 mg per 100 mL. Organic phosphate was reported to be 100–200 mg per 100 mL [Citation10]. Two sugar acids, 2-ketoglutaric acid and glucaric acid, were detected, as well. The former is an intermediate in the citric acid cycle, while the latter is an acidified side-product of glucose. Not surprisingly, Grace's insect medium supplies 2-ketoglutaric acid at a concentration of 2.5 mM (Cat# 11595, ThermoFisher Scientific, Waltham, MA). Finally, glucitol, a sugar alcohol, was detected in minor concentration (0.22 mM).
In summary, by compound class, TMS derivatization detected a composition of 60.1% non-proteinogenic amino acids; 18.1% amino acids; 8.2% phosphates; 4.2% organic acids; mono- and disaccharides; 0.7% sugar acids; and the sugar alcohol, glucitol, was 0.14%. Methyl chloroformate derivatization, which favors carboxylic compounds, detected metabolites from three metabolite classes: amino acids (92.5%), organic acids (14.7%), and fatty acids (1.7%). The knowledge of chemical composition of G. mellonella haemolymph sheds light why G. mellonella is a good infectious host model for many microorganisms. In addition to the reduced innate immune system, G. mellonella haemolymph provide these pathogens with the essentials nutrients needed to multiply.
Disclosure of potential conflicts of interest
No potential conflicts of interest were disclosed.
Acknowledgments
I acknowledge the lab member for the helpful discussion. I thank Shelley Jones for the technical assistance.
Additional information
Funding
References
- Tsai CJ-Y, Loh JMS, Proft T. Galleria mellonella infection models for the study of bacterial diseases and for antimicrobial drug testing. Virulence. 2016;7:214–229.
- Bergin D, Murphy L, Keenan J, et al. Pre-exposure to yeast protects larvae of Galleria mellonella from a subsequent lethal infection by Candida albicans and is mediated by the increased expression of antimicrobial peptides. Microbes Infect. 2006;8:2105–2112.
- Ramarao, N., C. Nielsen-Leroux, et al. The insect Galleria mellonella as a powerful infection model to investigate bacterial pathogenesis. J Vis Exp. 2012;70:e4392.
- Champion OL, Wagley S, Titball RW. Galleria mellonella as a model host for microbiological and toxin research. Virulence. 2016;7:840–845.
- Lemaitre B, Hoffmann J. The host defense of Drosophila melanogaster. Annu Rev Immunol. 2007;25:697–743.
- Harding CR, Schroeder GN, Collins JW, et al. Use of Galleria mellonella as a Model organism to study Legionella pneumophila infection. J Vis Exp. 2013;81:e50964.
- Navarro-Velasco GY, Prados-Rosales RC, Ortíz-Urquiza A, et al. Galleria mellonella as model host for the trans-kingdom pathogen Fusarium oxysporum. Fungal Genet Biol. 2011;48:1124mdash;1129.
- Seed KD, Dennis JJ. Experimental bacteriophage therapy increases survival of Galleria mellonella larvae infected with clinically relevant strains of the Burkholderia cepacia complex. Antimicrob Agents Chemother. 2009;53:2205–2208.
- Fuchs BB, O'Brien E, Khoury JB El, et al. Methods for using Galleria mellonella as a model host to study fungal pathogenesis. Virulence. 2010;1:475–482.
- Wyatt GR, Loughheed TC, Wyatt SS. The chemistry of insect hemolymph; organic components of the hemolymph of the silkworm, Bombyx mori, and two other species. J Gen Physiol. 1956;39:853–868.
- Wyatt GR, Kalf GF. The chemistry of insect hemolymph. II. Trehalose and other carbohydrates. J Gen Physiol. 1957;40:833–847.
- Thomas KK. Isolation and partial characterization of the haemolymph lipoproteins of the wax moth, Galleria mellonella. Insect Biochem. 1979;9:211–219.
- Hanzal R, Jegorov A. Changes in free amino acid composition in haemolymph of larvae of the wax moth, Galleria mellonella L., during cold acclimation. Comp Biochem Physiol Part A Physiol. 1991;100:957–962.
- Yendol WG. Fatty acid composition of Galleria larvae, hemolymph, and diet (Lepidoptera: Galleriidae). Ann Entomol Soc Am. 1970;63:339–341.
- Killiny N, Hijaz F, El-Shesheny I, et al. Metabolomic analyses of the haemolymph of the Asian citrus psyllid Diaphorina citri, the vector of huanglongbing. Physiol Entomol. 2017;42:134–145.
- Cheung PYK, Grula EA, Satayamurthy N, et al. Presence of free putrescine in the haemolymph of corn earworm (Heliothis zea) larvae. Insect Biochem. 1982;12:41–48.
- Silva ECC, Masui DC, Furriel RPM, et al. Regulation by the exogenous polyamine spermidine of Na,K-ATPase activity from the gills of the euryhaline swimming crab Callinectes danae (Brachyura, Portunidae). Comp Biochem Physiol Part B Biochem Mol Biol. 2008;149:622–629.
- Schneider BL, Hernandez VJ, Reitzer L. Putrescine catabolism is a metabolic response to several stresses in Escherichia coli. Mol Microbiol. 2013;88:537–350.
- Teulier L, Weber J-M, Crevier J, et al. Proline as a fuel for insect flight: enhancing carbohydrate oxidation in hymenopterans. Proceedings Biol Sci. 2016;283:20160333.
- Schartau W, Leidescher T. Composition of the hemolymph of the tarantula Eurypelma californicum. J Comp Physiol B. 1983;152:73–77.
- Visser B, Willett DS, Harvey JA, et al. Concurrence in the ability for lipid synthesis between life stages in insects. R Soc open Sci. 2017;4:160815.
- Stanley D, Haas E, Miller J. Eicosanoids: Exploiting insect immunity to improve biological control programs. Insects. 2012;3:492–510.
- Arrese EL, Soulages JL. Insect fat body: Energy, metabolism, and regulation. Annu Rev Entomol. 2010;55:207–225.
- Elbein AD, Pan YT, Pastuszak I, et al. New insights on trehalose: A multifunctional molecule. Glycobiology. 2003;13:17R–27R.
- Wang XH, Qi XL, Kang L. Geographic differences on accumulation of sugars and polyols in locust eggs in response to cold acclimation. J Insect Physiol. 2010;56:966–970.
- Saeidi F, Moharramipour S, Barzegar M. Seasonal patterns of cold hardiness and cryoprotectant profiles in Brevicoryne brassicae (Hemiptera: Aphididae). Environ Entomol. 2012;41:1638–1643.
- Douglas AE. Phloem-sap feeding by animals: Problems and solutions. J Exp Bot. 2006;57:747–754.
- Reyes-DelaTorre A, Peña-Rangel MT, Riesgo-Escovar JR. Carbohydrate metabolism in Drosophila: Reliance on the disaccharide trehalose. In: Chang, C-F (Ed). Carbohydrates – Comprehensive studies on glycobiology and glycotechnology. Rijeka: InTech; 2012. Ch 14:p. 317–338.
- Moriwaki N, Matsushita K, Nishina M, et al. High concentrations of trehalose in aphid hemolymph. Appl Entomol Zool. 2003;38:241–248.
- Shi J-F, Xu Q-Y, Sun Q-K, et al. Physiological roles of trehalose in Leptinotarsa larvae revealed by RNA interference of trehalose-6-phosphate synthase and trehalase genes. Insect Biochem Mol Biol. 2016;77:52–68.