ABSTRACT
Burkholderia pseudomallei: is the etiological agent of the disease melioidosis and is a Tier 1 select agent. It survives and replicates inside phagocytic cells by escaping from the endocytic vacuole, replicating in the cytosol, spreading to other cells via actin polymerization and promoting the fusion of infected and uninfected host cells to form multinucleated giant cells. In this study, we utilized a proteomics approach to identify bacterial proteins produced inside RAW264.7 murine macrophages and host proteins produced in response to B. pseudomallei infection. Cells infected with B. pseudomallei strain K96243 were lysed and the lysate proteins digested and analyzed using nanoflow reversed-phase liquid chromatography and tandem mass spectrometry. Approximately 160 bacterial proteins were identified in the infected macrophages, including BimA, TssA, TssB, Hcp1 and TssM. Several previously uncharacterized B. pseudomallei proteins were also identified, including BPSS1996 and BPSL2748. Mutations were constructed in the genes encoding these novel proteins and their relative virulence was assessed in BALB/c mice. The 50% lethal dose for the BPSS1996 mutant was approximately 55-fold higher than that of the wild type, suggesting that BPSS1996 is required for full virulence. Sera from B. pseudomallei-infected animals reacted with BPSS1996 and it was found to localize to the bacterial surface using indirect immunofluorescence. Finally, we identified 274 host proteins that were exclusively present or absent in infected RAW264.7 cells, including chemokines and cytokines involved in controlling the initial stages of infection.
Introduction
Melioidosis, an infection of humans and animals, is caused by the Gram-negative bacterium Burkholderia pseudomallei [Citation1,Citation2]. The disease occurs throughout the tropics, but is especially prevalent in Southeast Asia and northern Australia. The organism can be isolated from soil and water in endemic regions and infections occur following contact with environmental sources via inhalation, ingestion or cutaneous inoculation. Infections can be latent, chronic or acute and disease manifestation is largely dependent on the immune status of the host, the route of infection and the infectious dose. B. pseudomallei is designated as a Tier 1 Select Agent in the United States because of its potential for misuse as a biological weapon [Citation3]. No licensed melioidosis vaccine is currently available and antibiotic treatment can be challenging because the organism is naturally resistant to many antimicrobial agents [Citation4,Citation5].
B. pseudomallei possesses a relatively large genome (~7 Mb) consisting of two chromosomes and encoding nearly 6,000 proteins [Citation6,Citation7]. Numerous virulence determinants have been identified, but relatively little is known about the molecular pathogenesis of B. pseudomallei infection [Citation8]. The microbe is a facultative intracellular pathogen that can survive and replicate inside of host phagocytic cells [Citation2]. It employs multiple virulence factors that interfere with a macrophage’s innate ability to efficiently eliminate infection. The goal of this study was to identify bacterial proteins produced inside RAW264.7 murine macrophages and examine host cell proteins that are increased or decreased in response to B. pseudomallei infection.
Materials and methods
Bacterial strains, plasmids, and growth conditions
Escherichia coli and B. pseudomallei K96243 [Citation6] and MSHR668 [Citation9] were grown at 37°C on LB agar (Lennox formulation) or in Luria-Bertani broth (LB) (Lennox formulation), with 100 μg/ml adenine HCl and 5 μg/ml thiamine HCl for the purM select agent exempt strain Bp82 [Citation10]. When appropriate, antibiotics were added at the following concentrations: 25 µg/ml kanamycin (Km) and streptomycin (Sm) for E. coli and 25 µg/ml polymyxin B (Pm) and 500–1000 µg/ml Km for B. pseudomallei. For induction studies, isopropyl-ß-D-1-thiogalactopyranoside (IPTG) was added to a final concentration of 0.5 mM. A 20 mg/ml stock solution of the chromogenic indicator 5-bromo-4-chloro-3-indolyl-ß-D-galactopyranoside (X-Gal) was prepared in N,N-dimethylformamide, and 40 μl was spread onto the surface of plate medium for blue/white screening in E. coli TOP10 or E. cloni® 10 G chemically competent cells. All manipulations with B. pseudomallei select agent strains were carried out in a class II microbiological safety cabinet located in a designated biosafety level 3 (BSL-3) laboratory. Other strains were handled in in a class II microbiological safety cabinet located in a designated BSL-2 laboratory.
Sample preparation prior to mass spectrometric analysis
Proteins from control and infected cell lysates were extracted and quantitated using a BCA assay (ThermoFisher Scientific, Cat. No. 23225). Equal amounts of protein from samples prepared on two separate occasions were reduced, alkylated and trypsin digested overnight using an enzyme-to-protein ratio of 1:50. Tryptic peptides were further desalted using a C18 spin column. One twentieth of each sample was lyophilized and reconstituted in 0.1% trifluoroacetic acid (TFA) and analyzed in quadruplicate without fractionation for quantitation. The remainder of the tryptic peptides were lyophilized dissolved in 25% acetonitrile with 0.1% formic acid and further fractionated using strong cation-exchange (SCX) chromatography [Citation11]. The SCX fractions of the two samples were pooled into 10 fractions each, lyophilized and reconstituted in 0.1% TFA to be analyzed by liquid chromatography mass spectrometry (LCMS).
Nanobore reversed-phase liquid chromatography tandem MS (nanoRPLC-MS/MS)
NanoRPLC-MS/MS was performed using an Agilent 1200 nanoflow LC system coupled online with a LTQ Orbitrap Velos mass spectrometer. The RPLC column (75 µm i.d. x 10 cm) were slurry-packed in-house with 5 µm, 300 Å pore size C-18 stationary phase into fused silica capillaries with a flame pulled tip. After sample injection, the column was washed for 20 min with 98% mobile phase A (0.1% formic acid in water) at 0.5 µl min−1. Peptides were eluted using a linear gradient of 2% mobile phase B (0.1% formic acid in ACN) to 35% B in 100 minutes, then to 80% B over an additional 20 minutes. The column flow-rate was maintained at 0.25 µl min−1 throughout the separation gradient. The mass spectrometer was operated in a data-dependent mode in which each full MS scan was followed by ten MS/MS scans wherein the ten most abundant molecular ions were dynamically selected for collision-induced dissociation (CID) using a normalized collision energy of 35%.
Identification and quantification of proteins from nanoRPLC-MS/MS
The RPLC-MS/MS data were processed using MaxQuant software (version 1.2.2.5). MS/MS data were searched by the Andromeda search engine against a combined decoy database of mouse database and B. pseudomallei database containing both forward and reverse sequences. Dynamic modifications of methionine oxidation and N-terminal acetylation as well as fixed modification of carbamidomethyl cysteine were also included in the database search. Only tryptic peptides with up to two missed cleavage sites with a minimum peptide length of six amino acids were allowed. The false discovery rate (FDR) was set to 0.01 for both peptide and protein identifications. Mouse protein changes due to B. pseudomallei infection were analyzed using label-free quantification (LFQ) function of MaxQuant. Quantitation results were further analyzed using Perseus program (version 1.2.0.17). Significant protein changes were determined using a two sample t-test, protein intensity replicates for the two samples were grouped and the statistical test was performed with an FDR value of 0.001.
DNA manipulation
Restriction enzymes (Roche Molecular Biochemicals), Antarctic phosphatase (New England BioLabs, Cat. No. M0289S), and T4 DNA ligase (Roche Molecular Biochemicals, Cat. No. 10481220001) were used according to the manufacturer’s instructions. When necessary, the End-It DNA End-Repair Kit (Lucigen, Cat. No. ER0720) was used to convert 5ʹ or 3ʹ protruding ends to blunt-ended DNA. DNA fragments used in cloning procedures were excised from agarose gels and purified with a GENECLEAN Kit (MP Biomedicals, Cat. No. SKU 111001200). Bacterial genomic DNA was prepared by using a previously described protocol [Citation12]. Plasmids were purified from overnight E. coli cultures by using Wizard Plus SV Minipreps DNA Purification System (Promega, Cat. No. A1460).
PCR amplifications
PCR products were sized and isolated using agarose gel electrophoresis, cloned using the pCR2.1-TOPO TA cloning kit (Thermo Fisher Scientific, Cat. No. 450641), and transformed into chemically competent E. coli TOP10 or E. cloni® 10 G. PCR amplifications were performed in a final reaction volume of 50 μl containing FailSafe PCR System with 1X PreMix D (Lucigen, Cat. Nos. FS99100 and FSP995D-INCL), 1.25 U FailSafe PCR Enzyme Mix, 1 μM PCR primers and approximately 200 ng of genomic DNA. Colony PCR was utilized to screen for B. pseudomallei deletion mutants. Briefly, sucrose resistant and Km sensitive colonies were resuspended in 50 µl water and 5 µl was added to the PCR reaction rather than purified genomic DNA. PCR cycling was performed using a PTC-150 minicycler with a Hot Bonnet accessory (MJ Research, Inc.) and heated to 97°C for 5 min. This was followed by 30 cycles of a three-temperature cycling protocol (97°C for 30 s, 55°C for 30 s and 72°C for 1 min) and one cycle at 72°C for 10 min. For PCR products greater than 1 kb, an additional 1 min per kb was added to the extension time.
The primers used to PCR-amplify BPSS1996 (BURPS668_A2871) were 1996-up (5ʹ-GCTAGCCGATTCGATCCTCGTGCAAC-3) and 1996-dn (5ʹ-GCTAGCAGATGCTCAATCGCAAGCTG-3ʹ). BPSL2748 (BURPS668_3186) was PCR-amplified with 2748-up (5ʹ-GCTAGCGTGAACACGTACGAAGGTAC-3ʹ) and 2748-dn (5ʹ-GCTAGCACTTGTCCGGATGCGCGATG-3ʹ).
Production and purification of recombinant Burkholderia proteins
BPSS1996 and hcp1 (hemolysin-coregulated protein 1) [Citation13] were synthesized, cloned, expressed and the encoded proteins were purified commercially (Biomatik). The genes were synthesized with encoded C-terminal 6X His-tags and flanking enzyme restriction sites. Primers were designed and used to clone the PCR products into the NdeI and HindIII sites of plasmid pUC57 (GenBank Accession Y14837.1). E. coli clones harboring the target sequence were identified by blue/white screening. Recombinant plasmid DNA was extracted from the clones and Sanger sequencing done to confirm the plasmid construct. Expression and amplification of the recombinant proteins were performed by the supplier using standard procedures, and the proteins were purified on nickel Ni2+ His-tag affinity columns. The final proteins were shown to be >90% pure by Coomassie blue-stained SDS-PAGE and western blot using an anti-6X His antibody.
Polyacrylamide gel electrophoresis (PAGE) of purified Burkholderia proteins
Purified proteins were adjusted to 100 µg/ml and combined with Novex™ Tricine SDS Sample Buffer (Thermo Fisher Scientific, Cat. No. LC1676) before loading the wells of a Novex™ 16% Tricine Protein Gel (Thermo Fisher Scientific, Cat. No. EC6695BOX); the lanes contained 1.0–1.5 µg protein each. A lane with Novex™ Sharp Pre-stained Protein Standard (Thermo Fisher Scientific, Cat. No. LC5800) was included for size estimations. The gel was electrophoresed and stained with SimplyBlue™ SafeStain (Thermo Fisher Scientific, Cat. No. LC6060).
Detection of serum antibodies to BPSS1996 and Hcp1 in a B. pseudomallei-infected rhesus macaque
Enzyme-linked immunosorbent assay (ELISA) was performed by coating microtiter plate wells with 25 µg/ml of recombinant BPSS1996 and Hcp1. Irradiation-inactivated B. pseudomallei K96243 (BPK) [Citation14] served as a positive control and buffer as a negative control. The antigens were incubated with nonhuman primate (NHP) antiserum from a B. pseudomallei K96243-infected rhesus macaque and developed with a goat anti-rhesus IgG (H + L)-HRP secondary antibody (Southern Biotech, Cat. No. 6200–05). The NHP antiserum had an anti-BPK reciprocal titer of 12,150,000 by ELISA (data not shown).
BALB/c mouse challenge studies
Bacterial strains were grown overnight in LB broth, serially diluted in PBS, and aliquots were spread onto LB agar plates to determine the number of colony forming units (CFU) present. Six- to eight-week-old female BALB/c mice, 10 per group, were challenged by the intraperitoneal (i.p.) route with 101–104 CFU of B. pseudomallei MSHR668, 668 ΔS1996, and 668 ΔL2748. The animals were observed at least once daily and moribund animals were euthanized by CO2 exposure. On day 21, the surviving animals from each group were euthanized with CO2. A Bayesian probit analysis was performed for each strain to estimate the lethal dose response curve and the 50% lethal dose (LD50). Prior distributions for each parameter were assumed to be independent, weakly informative Cauchy distributions with center 0 and scale 10. Using samples from the posterior distributions of the slope and intercept parameters from the probit analysis, the median and 95% credible intervals of the range of dose responses were estimated.
Research was conducted under an IACUC approved protocol in compliance with the Animal Welfare Act, PHS Policy, and other Federal statutes and regulations relating to animals and experiments involving animals. The facility where this research was conducted, USAMRIID, is accredited by the Association for Assessment and Accreditation of Laboratory Animal Care, International and adheres to principles stated in the Guide for the Care and Use of Laboratory Animals, National Research Council, 2011.
Immunization of BALB/c mice with BPSS1996 and Hcp1 and challenge with B. pseudomallei K96243
BALB/c mice were immunized with a prime dose of protein solution and boosted twice, at day 21 and again at day 35 after the prime dose. The doses each contained 25 µg of purified protein (BPSS1996 or Hcp1) combined with 500 µg Alhydrogel (500 µg), 20 µg deoxyoligonucleotide CpG (ODN2006) (InvivoGen, Cat. No. tlrl-2006-5), and PBS in a total volume of 200 µl. Control mice received the Alhydrogel/ODN2006 solution in PBS alone. One group was administered a solution containing a combination of both proteins. Each animal group consisted of sixteen mice, six of which were euthanized just prior to the challenge date for collection of polyclonal antisera to BPSS1996 and Hcp1. The remaining ten mice were challenged via the i.p. route 30 days after the last vaccine dose with an equivalent to 5–10 LD50s of B. pseudomallei strain K96243. Endpoint antibody titers of the sera were determined by ELISA as described above.
Immunofluorescence microscopy
Bacteria were grown overnight in LB media with and without antibiotics. The cells were centrifuged (4000 g, 5 min), washed twice with PBS and resuspended in 750 µl of 4% paraformaldehyde for 15 min at room temperature to fix. The cells were washed three times with nanopure water containing 1% BSA and 30 µl aliquots were added to poly-L-lysine coated slides and allowed to air dry. Fifty microliters of a 1:1000 dilution of mouse polyclonal BPSS1996 antisera in 2% BSA was added to the cells and incubated for 1 h at room temperature. The slides were washed with 1% BSA and incubated with a 1:1000 dilution of Alexa®Fluor 488–tagged goat anti-mouse IgG (H + L) antibody (Invitrogen, Cat. No. A11001) for 30 min. The slides were washed with 1% BSA, allowed to air dry and a drop of mounting media was added. The slides were viewed using a Nikon eclipse 90i microscope with phase contrast (100x, oil immersion objective) and fluorescence microscopy.
Construction and complementation of B. pseudomallei mutants
Gene replacement experiments with B. pseudomallei were performed using the sacB-based vector pMo130, as previously described [Citation15–Citation17]. The 1043-bp 2748-up/2748-dn PCR product was cloned into pCR2.1-TOPO (pCR2.1-BPSL2748), digested with Eco47III and SalI and the ends were blunt-ended and ligated. The resulting plasmid, pCR2.1-∆BPSL2748, contains a 359-bp deletion of BPSL2748. The insert was released with NheI and cloned into the corresponding site of pMo130 and designated pMo130-∆BPSL2748. The 1370-bp 1996-up/1996-dn PCR product was cloned into pCR2.1-TOPO (pCR2.1-BPSS1996), digested with ClaI and BsiWI and the ends were blunt-ended and ligated. The resulting plasmid, pCR2.1-∆BPSS1996, contains a 341-bp deletion of BPSS1996. The insert was released with NheI and cloned into the corresponding site of pMo130 and designated pMo130-∆BPSS1996. The NheI insert from pCR2.1-BPSS1996 was also cloned into the corresponding site of pMo130, resulting in pMo130-BPSS1996. This construct was linearized with BsiWI (14-bp upstream of the BPSS1996 stop codon), blunt-ended with the End-It DNA End-Repair Kit and treated with Antarctic Phosphatase. A promoter-less green fluorescent protein (GFP) gene was generated by digesting pCR2.1-GFP [Citation18] with BamHI and XbaI and treating with the End-It DNA End-Repair Kit. The blunt-ended and promoter-less GFP gene was cloned into the repaired BsiWI site of pMo130-BPSS1996 so that the BPSS1996 and GFP genes were in the same orientation (pS1996-GFP). The recombinant derivatives of pMo130 were electroporated (12.25 kV/cm) into E. coli S17-1 [Citation19] and conjugated with B. pseudomallei MSHR668 and Bp82 for 8 h. Pm was used to counterselect E. coli S17-1. Optimal conditions for resolution of the sacB constructs were found to be LB agar lacking NaCl and containing 10% (w/v) sucrose, with incubation at 25°C for 4 days. B. pseudomallei deletion mutants were identified by colony PCR using the primers described above. The PCR products generated from the deletion mutant strains were smaller than those obtained from the wild-type strain, but the BPSS1996-GFP allele resulted in a larger PCR product than BPSS1996.
The BPSS1996 insert was released from pCR2.1-BPSS1996 with EcoRI and SalI and cloned into the corresponding sites of the broad-host-range plasmid pBHR2 [Citation18], creating pBHR2-BPSS1996. The purified plasmid was electroporated into Bp82 ∆BPSS1996. Briefly, the organism was grown overnight in 5 ml of LB supplemented with adenine and thiamine. The culture was centrifuged, the pellet was washed twice with 3 ml of ice-cold 10% glycerol and resuspended in 1 ml of 10% glycerol. Approximately 1 μg of pBHR2 and pBHR2-BPSS1996 were mixed with 40 μl of Bp82 ΔBPSS1996 and incubated on ice for 15 mins. The mixtures were transferred to 2 mm diameter cuvettes (Thermo Fisher Scientific, Cat. No. BTX620) and electroporated for 4 ms, 2.5kv/cm and a constant capacitance of 25μF. The electroporated mixtures were immediately resuspended in 1 ml of 50% recovery media (Lucigen, Cat. No. F98226-1) and 50% LB supplemented with adenine and thiamine, transferred to 15 ml snap-cap polypropylene tubes and incubated at 37°C for 4 hours with constant agitation (250 rpm). One hundred microliters of the mixture was spread onto LB agar plates containing adenine, thiamine and Km and incubated for three days at 37°C.
Gentamicin protection assays in RAW264.7 cells
RAW264.7 cells were grown at 37°C in the presence of 5% CO2 in Dulbecco’s Modified Eagle Medium with 10% (v/v) fetal bovine serum (DMEM-10). The cells were infected with B. pseudomallei at a multiplicity of infection (MOI) of 10 for 1.5 h at 37°C, 5% CO2 to allow bacterial uptake. The cell culture media was removed and the cells were washed twice with Hanks’ Balanced Salt Solution (HBSS). DMEM-10 containing 200 μg/ml gentamicin was added to the cells and incubation was continued for an additional 10–24 h depending on the assay. For the LC-MS/MS studies (), the media was removed at 12 h post-infection and the RAW cells were washed with PBS. The RAW264.7 cells were then lysed with 0.1% (v/v) triton X-100 in water, filtered to remove bacteria, and the lysates frozen at −70°C until they could be processed. Uninfected RAW264.7 cells served as a control for this experiment. For the intracellular replication and survival studies (), at 1.5, 6, 9, 12, and 24 h post-infection, the RAW264.7 cells were washed, lysed and intracellular bacterial numbers were quantitated by spreading serial dilutions of the lysates on LB plates and incubating at 37◦C for 24–48 h. The experiment was performed in triplicate and the numerical values represent the mean ± S.D.
Figure 1. Workflow diagram of the cell culture and proteomics procedures that were used in this study. RAW264.7 macrophages and macrophages infected with B. pseudomallei K96243 at a MOI of 10 were lysed and filtered at 12 hours after infection. The resulting protein samples were digested with trypsin, subject to nanoRPLC-MS/MS, identified and quantified.
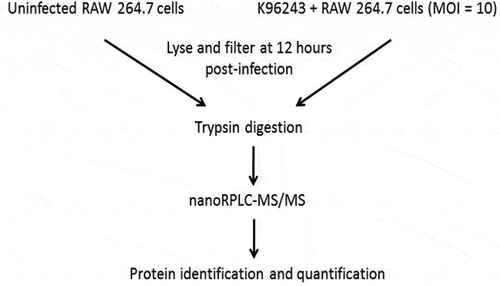
Statistical analyses
Significant protein changes identified with MaxQuant were determined using a two sample t-test; protein intensity replicates for the two samples were grouped and the statistical test was performed with an FDR value (expected proportion of false positives) of 0.001. The results of the ELISA assays for serum antibodies were evaluated by a two-way analysis of variance (ANOVA) with posthoc Tukey’s multiple pairwise comparisons tests, as performed using Prism GraphPad version 8.4.2. A Bayesian probit analysis was performed for each strain to estimate the lethal dose response curve and the LD50. Prior distributions for each parameter were assumed to be independent, weakly informative Cauchy distributions with center 0 and scale 10. Using samples from the posterior distributions of the slope and intercept parameters from the probit analysis, the median (and 95% credible intervals of the range of dose responses), and the probabilities of significant differences in LD50 were estimated. The viable count data obtained in the cell culture assay were evaluated with the Welch’s (unequal variance) t-test using the mean log10-transformed CFU/ml for each time-point.
Results
One hundred sixty B. pseudomallei proteins identified inside of RAW264.7 cells at 12 hours post-infection
Proteomics is a powerful tool for the study of proteins in complex mixtures including cells, body fluids, tissues, organisms and other complicated samples [Citation20]. Proteome analysis provides unique information about the modulation of host proteins during infection and can be used to identify bacterial proteins produced during infection. In this study, we utilized a proteomics approach to better understand the Burkholderia-macrophage interaction at the molecular level. Previous studies have shown that B. pseudomallei is present in the host cell cytosol in relatively large numbers by 12 h post-infection [Citation13] and we chose this time point for our proteomic analysis. RAW264.7 cells were infected with B. pseudomallei K96243 for 12 h and then washed, lysed and the lysate was filter-sterilized to remove intact bacteria (). The bacterial and host proteins were digested with trypsin and analyzed using nanoRPLC-MS/MS. One hundred sixty K96243 proteins were identified inside RAW264.7 cells at 12 h post-infection ( and S1), including some previously known to be produced only inside host cells (Hcp1, TssA, TssB, and BimA) [Citation13,Citation21–Citation24]. This result indicated that our methodology was technically sound and working as predicted. also shows some of the other B. pseudomallei K96243 proteins found inside RAW264.7 cells at 12 h post-infection. TssM, a known B. pseudomallei secreted effector protein with an N-terminal signal sequence, was among the proteins identified [Citation15,Citation24]. While our initial goal was to enrich for bacterial proteins secreted inside macrophages, many cytoplasmic and cell-associated bacterial proteins were also released inside the host cells by the inherent bactericidal activity of the RAW264.7 cells ( and S1). This result suggests that not all bacteria are fully intact at 12 h post-infection in RAW264.7 cells and the bacterial proteins in and S1 more accurately represent the cytoplasmic, cell-associated and secreted proteins that are abundantly produced when B. pseudomallei is grown intracellularly.
Table 1. Selected B. pseudomallei K96243 proteins identified inside RAW264.7 macrophages at 12 hours post-infection.
The B. pseudomallei proteins detected within RAW264.7 cells included those encoding stress-associated proteins (heat shock proteins and detoxifying enzymes), outer membrane proteins (OMPs), putative exported proteins and cytoplasmic elongation factors ( and S1). The bacterial heat shock proteins (HSPs) detected inside macrophages included the chaperones GroES, GroEL, HtpG, DnaK and GrpE (). These proteins are highly conserved proteins but have been shown to have important roles in infection, and in the immune response to and diagnosis of infection [Citation25–Citation33]. Additional intracellularly produced bacterial proteins associated with host-mediated nutritional and oxidative stress included the universal stress protein A (UspA), peroxiredoxin BPSL2748 and superoxide dismutase BPSL0880 (SodB). Three of the twelve OmpA family proteins encoded in the B. pseudomallei K96243 genome, BPSL0999, BPSL2765 and BPSL2522, were also detected inside RAW264.7 macrophages () [Citation34]. These proteins interact with peptidoglycan to maintain membrane and cell morphology and are known to be involved in bacterial virulence [Citation35,Citation36]. Another B. pseudomallei OMP induced inside macrophages, BamC, is part of the OMP assembly complex (BAM) involved in assembly and insertion of beta-barrel proteins into the outer membrane. It stabilizes the interaction between the essential proteins BamA and BamD [Citation37,Citation38]. Several of the proteins in are predicted to be putative exported proteins because they harbor N-terminal signal sequences, including the secreted effector TssM [Citation15,Citation24]. Finally, the cytoplasmic elongation factors EF-Tu, EF-Ts, and EF-G, which are essential to the process of translating RNA into proteins, were also identified inside the infected macrophage cells ( and S1). This result indicates that there was likely appreciable bacterial lysis in RAW264.7 cells by 12 h post-infection and some of the proteins in and S1 represent integral cytosolic bacterial proteins abundantly produced in the intracellular niche. The overall Burkholderia expression profile in cultured macrophages appears to resemble the B. pseudomallei gene expression pattern observed in human patients, as discussed below.
Two hundred seventy-four host proteins were exclusively present or absent in infected RAW264.7 cells at 12 h post-infection
We predict that B. pseudomallei growth inside macrophages will modulate the production of numerous components of the innate immune system and signaling pathways involved in the pathogenesis of infection. The proteomic analysis of uninfected and infected RAW264.7 cells yielded 274 host proteins that were exclusively present (58) or absent (216) in the infected cells ( and S2). Furthermore, there were >100 macrophage proteins that exhibited at least a 10-fold difference under these two experimental conditions (data not shown). Among the 15 host proteins most impacted by B. pseudomallei K96243 infection, nine were up-regulated and six were down-regulated compared to the protein levels in uninfected cells (). Two of the three most highly upregulated proteins were the cytokines interleukin 1α (IL-1α) and 1β (IL-1β), proinflammatory cytokines having pleiotropic activities. They play a central role in regulation of the immune response and inflammation and directly activate NF-κB, a global inflammatory response regulator. Several other cytokines/chemokines or their receptors displayed increased abundance, i.e., chemokine CXCL2 and C-C receptor CCR1 and granulocyte colony stimulating factor. G-CSF stimulates the bone marrow to produce polymorphonuclear leukocytes and their precursor stem cells and thus has an important role in the inflammatory response to infection. Similarly, elevated levels of complement protein C3, the most abundant protein of the complement system, signals a stimulated host response to B. pseudomallei infection.
Table 2. RAW 264.7 proteins exclusively present or absent inside Burkholderia-infected cells at 12 hours post-infection.
There were also significant changes in proteins involved in apoptotic cell-death functions. Three of the upregulated proteins (CD40, TNFRSF1B and TRAF1) are involved in the expression of tumor necrosis factor-alpha (TNF-α), a major stimulator of apoptosis. Nevertheless, the pleiotrophic TNF-α is also an important proinflammatory cytokine produced in higher abundance in individuals with melioidosis as compared with uninfected healthy individuals [Citation39]. Furthermore the only proteins typically associated with apoptosis and which were notably altered in expression were the enzyme caspase 6 and the caspase recruitment domain family protein CARD9. They were down-regulated. Finally, the six down-regulated proteins () also include entities involved in the down-regulation or reduced expression of the transcriptional activator NF-κB. Thus, all of the host proteins identified in as being strongly affected by infection may play important roles in the pathogenesis of B. pseudomallei in the host.
Construction of deletion mutations in two B. pseudomallei genes expressed in RAW267.4 cells
Bacterial proteins produced inside of host cells represent potential virulence determinants and several of the B. pseudomallei proteins we identified inside RAW264.7 cells are known virulence factors, including Hcp1, BimA and TssM (). Many of the K96243 proteins we identified in RAW264.7 at the 12 h time point are proteins that are novel or have no defined function. We selected two K96243 genes, BPSS1996 and BPSL2748 ( and ), for mutagenesis in the heterologous strain MSHR668 [Citation9]. This B. pseudomallei strain was selected for further studies because it is highly virulent for BALB/c mice by the i.p. route of infection (see below). Our K96243 isolate, on the other hand, is noticeably less virulent for BALB/c mice by the i.p. route of infection [Citation40]. BPSL2748 is predicted to be a peroxiredoxin, an antioxidant enzyme that uses thioredoxin to recharge after reducing hydrogen peroxide to water. The gene encoding this protein appears to be monocistronic (). BPSS1996 is a novel protein with a signal peptide and a Domain of Unknown Function designated DUF4148 (,). The gene encoding the DUF4148 protein is present immediately downstream of a predicted two-component signal transduction regulatory system (TCS) and may be part of a three gene operon (). The upstream TCS, BPSS1994-BPSS1995 (IrlR2-IrlS2), most closely resembles the B. pseudomallei IrlRS TCS that regulates genes involved in resistance to Cd2+ and Zn2+ [Citation41]. In fact, IrlR2 is 73% identical to IrlR and IrlS is 50% identical to IrlS [Citation41]. It is currently unknown if IrlR2S2 TCS is also involved in regulating resistance to heavy metals. Interestingly, a different DUF4148 gene (BPSS1038) is present immediately downstream of the irlR and irlS genes. No previous studies have identified any interaction of DUF4148 proteins with bacterial TCS and the close relationship of these genes in these two clusters may be coincidental.
Figure 2. Genomic context of the genes analyzed in this study and the domain organization of BPSS1996. a) B. pseudomallei K96243 chromosome 1 genes BPSL2747-BPSL2751 are shown as arrows and BPSL2748, a gene encoding a putative peroxiredoxin, AhpC-type, is shaded. b) B. pseudomallei K96243 chromosome 2 genes BPSS1993-BPSS1997 are shown as arrows and BPSS1996, a gene encoding a DUF4148 protein, is shaded. BPSS1993 (mprA) encodes metalloprotease A, a serine protease of the subtilisin family [Citation75]. BPSS1994 (irlR2) and BPSS1995 (irlS2) encode a two-component regulatory system and exhibit > 70% nucleotide identity with the B. pseudomallei 1026b invasion-related locus genes irlR and irlS (33). BPSS1997 (oxa) encodes a class D β-lactamase [Citation76]. A 1 kb scale for A) and B) is shown at the bottom of B). Open spaces between the genes represent non-coding intergenic regions. c) Domain organization of the 113 amino acid protein BPSS1996. The N-terminal 53 amino acids of BPSS1996 form a DUF4148 superfamily domain, which includes a 21 amino acid signal peptide [Citation77].** The C-terminal 60 amino acids do not share sequence similarity with any of the currently recognized protein domain families.
![Figure 2. Genomic context of the genes analyzed in this study and the domain organization of BPSS1996. a) B. pseudomallei K96243 chromosome 1 genes BPSL2747-BPSL2751 are shown as arrows and BPSL2748, a gene encoding a putative peroxiredoxin, AhpC-type, is shaded. b) B. pseudomallei K96243 chromosome 2 genes BPSS1993-BPSS1997 are shown as arrows and BPSS1996, a gene encoding a DUF4148 protein, is shaded. BPSS1993 (mprA) encodes metalloprotease A, a serine protease of the subtilisin family [Citation75]. BPSS1994 (irlR2) and BPSS1995 (irlS2) encode a two-component regulatory system and exhibit > 70% nucleotide identity with the B. pseudomallei 1026b invasion-related locus genes irlR and irlS (33). BPSS1997 (oxa) encodes a class D β-lactamase [Citation76]. A 1 kb scale for A) and B) is shown at the bottom of B). Open spaces between the genes represent non-coding intergenic regions. c) Domain organization of the 113 amino acid protein BPSS1996. The N-terminal 53 amino acids of BPSS1996 form a DUF4148 superfamily domain, which includes a 21 amino acid signal peptide [Citation77].** The C-terminal 60 amino acids do not share sequence similarity with any of the currently recognized protein domain families.](/cms/asset/e4046765-003b-414a-9190-c60a6b5ebe7b/kvir_a_1806675_f0002_b.gif)
B. pseudomallei 668 ΔS1996 is highly attenuated in BALB/c mice
In order to determine the relative virulence of the mutant strains, we infected BALB/c mice by the i.p. route with 10, 100, 1,000, and 10,000 CFU and monitored them for 21 days. B. pseudomallei 668 ∆S1996 (∆BPSS1996) was highly attenuated in BALB/c mice at all doses examined, but there was no significant difference in the virulence of 668 ∆L2748 (∆BPSL2748) and MSHR668 ( and S1). The 50% lethal dose (LD50) for 668 ∆S1996 in BALB/c mice was 7,240 CFU, ~ 55-fold higher than the LD50 for MSHR668 (134 CFU, p = 0.01). Our results demonstrate that BPSS1996 is an important B. pseudomallei virulence factor. BPSL2748, on the other hand, is not required for virulence in this animal model of infection. It was not attenuated in virulence and had an LD50 of 61 CFU, not significantly different from that of strain 668 ( and S1).
B. pseudomallei 668 ΔS1996 does not exhibit a growth deficiency in rich media or inside RAW264.7 cells
We next examined the growth of 668 ΔS1996 in LB broth to ensure that the virulence deficiency of this strain was not simply due to a growth defect. The growth of this strain and the parental strain, MSHR668, were indistinguishable in LB broth (Fig. S2). In addition, we constructed a single-copy BPSS1996-GFP transcriptional fusion on the chromosome to examine the expression of BPSS1996 in rich broth. shows that the strain possessing the BPSS1996-GFP allele, 668 S1996-GFP, exhibits green fluorescence when grown in LB broth, but MSHR668 does not. The results demonstrate that while BPSS1996 is expressed during growth in rich broth, the gene is not required for optimal growth in this medium ( and S2).
Figure 4. A BPSS1996-GFP transcriptional fusion is expressed when B. pseudomallei is grown in rich medium. a) MSHR668 and b) 668 S1996-GFP were grown in LB broth for 18 h, washed with PBS and viewed with a Nikon Eclipse 90i Fluorescent Microscope using the 100x oil immersion objective. The left panels show bacteria by differential interference contrast (DIC), the middle panels show green fluorescent protein (GFP) expression and the right panels present a merged image of the DIC and GFP images.
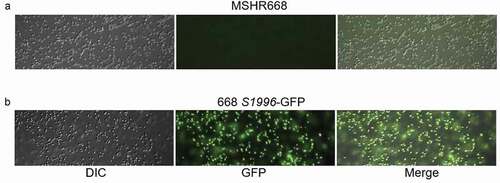
Since the BPSS1996 protein was identified inside K96243-infected macrophages (), we next examined if a mutation in the gene encoding this protein had an effect on the ability of B. pseudomallei to survive and replicate inside of macrophages. A gentamicin protection assay was performed to examine the ability of 668 ∆S1996 to survive and replicate inside RAW264.7 cells. demonstrates that 668 ∆S1996 does not display a survival or replication phenotype in RAW264.7 cells and suggests that the virulence phenotype of this strain in mice is unrelated to survival and replication in macrophages in vivo. The mean recovery of viable bacteria of the mutant and wild type strain at each of five time-points after infection did not differ significantly (p = 0.17, 0.57, 0.10, 0.40, and 0.10, respectively ().
Figure 5. Gentamicin protection assay in RAW264.7 cells. The cells were infected at a multiplicity of infection of ~10 with B. pseudomallei MSHR668 and 668 ∆S1996. At 1.5, 6, 9, 12, and 24 hours post-infection, the RAW264.7 cells were lysed and intracellular bacterial numbers were quantitated by spreading serial dilutions of the lysates on LB plates and incubating at 37◦C for 24–48 hours. The experiment was performed in triplicate and the numerical values represent the mean ± the standard deviation.
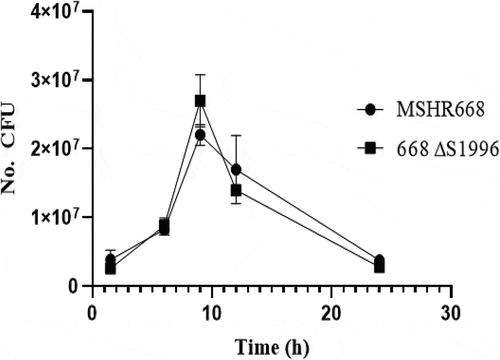
BPSS1996 is immunogenic in a rhesus macaque and BALB/c mice, but does not confer protection against a lethal B. pseudomallei challenge
The genes encoding BPSS1996 and Hcp1 were synthesized, cloned, expressed and purified commercially (Biomatik), as described in the Materials and Methods. Hcp1 is a critical component of the cluster 1 type six secretion system (T6SS-1) and was included in these studies as a known immunogenic virulence-associated protein of B. pseudomallei [Citation13]. To verify purity of the recombinant proteins, BPSS1996 and Hcp1 were subjected to PAGE analysis. As shown in , the migration of the proteins on a polyacrylamide gel stained with Coomasie blue agreed with the predicted sizes of 22 kDa for Hcp1 and 12 kDa for BPSS1996. Both proteins were detected by antisera collected from a B. pseudomallei-infected nonhuman primate (NHP), indicating that BPSS1996 is produced in vivo and stimulates the host immune system ().
Figure 6. Polyacrylamide gel electrophoresis of Burkholderia pseudomallei proteins. Purified proteins Hcp1 and BPSS1996 were electrophoresed to confirm their purity, mobility and size. The gel was run and stained with Coomassie blue and the samples were adjusted to 100 µg/ml before loading the wells. The bands sizes agreed with the predicted sizes of 12 kDa for BPSS1996 and 22 kDa for Hcp1. Lanes (left to right): 1, Protein ladder; 2, BPSS1996; 3, Hcp1. Protein Molecular Weight (http://www.cellbiol.com/sequence_manipulation_suite/protein_mw.php) was utilized to predict the molecular weights of Hcp1 and BPSS1996 using the corresponding amino acid sequences.
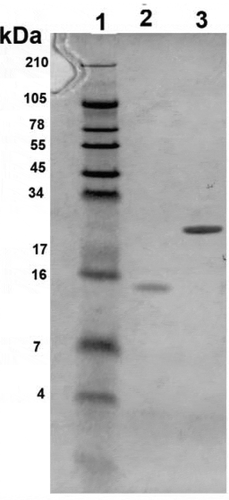
Figure 7. Immunoreactivity of Hcp1 and BPSS1996 with antiserum from a rhesus macaque infected with B. pseudomallei by ELISA. Microtiter plate wells were coated with 25 µg/ml of the antigens being tested (left to right): Hcp1, BPSS1996, killed whole cell B. pseudomallei strain K96243 (BPK) as positive control, or buffer alone. The control wells (blank) were incubated with buffer alone in the absence of an antigen. The proteins were detected with anti-Burkholderia antiserum from a rhesus macaque infected with B. pseudomallei strain K96243 as the primary antibody and developed with HRP conjugated goat-anti-monkey secondary antibody. All antigen-antibody samples were assayed in duplicate and the data were significantly different by ANOVA performed as described in the Materials and Methods. All 24 pairwise comparisons by dilution were significantly different with p < 0.0001 except for comparison of the reciprocal dilution 800 values of BPSS1996 and the blank sample, with the former being greater than the latter, p = 0.0002.
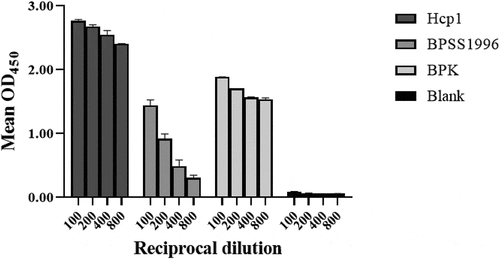
BALB/c mice were immunized three times subcutaneously with Hcp1, BPSS1996 and Hcp1 + BPSS1996 in a formulation containing Alhydrogel (adjuvant) and ODN2006 (immunostimulator). Control mice were vaccinated with Alhydrogel/ODN2006 in PBS alone. Each group consisted of sixteen mice, six of which were euthanized just prior to the challenge date for serum collection and analysis. The Hcp1- and BPSS1996-immunized mice generated strong IgG responses to the recombinant proteins, with mean reciprocal endpoint titers of 4,841,700 (Hcp1) and 720,000 (BPSS1996) (Table S3). The remaining ten mice in each group were challenged one month after the last vaccine dose by the i.p. route with 6.6 × 105 CFU of B. pseudomallei K96243 (~10 LD50s). All mice died by day 21 and the recombinant proteins, alone or in combination, did not extend the time to death (TTD) relative to the control group (). In fact, the TTD of the Hcp1 + BPSS1996 group was shorter than that of the mock-vaccinated group by nearly five days. Thus, the proteins alone or in combination were not protective and may possibly have exacerbated the infection and hastened the TTD compared to unvaccinated controls. These responses were not due to the absence of an immune response to the proteins as the level of IgG anti-Hcp1 and anti-BPSS1996 antibodies were substantial (Table S3). It is conceivable that the recombinant proteins induced a damaging host response referred to as a “cytokine storm”, a well-documented phenomenon which has been described in many other vaccination and infection scenarios [Citation42]. Alternately, although primarily observed in viral infections, antibody-dependent enhancement of infection (ADE) has been described for bacterial diseases [Citation43].
Table 3. Survival of BALB/c mice vaccinated with Hcp1 and/or BPSS1996 and challenged with a lethal i.p. dose of B. pseudomallei K96243.
Localization of BPSS1996 to the surface of B. pseudomallei
Previous studies have identified BPSS1996 as an OMP [Citation44] that elicits a humoral immune response in melioidosis patients [Citation45]. Immunofluorescence microscopy was utilized to detect the localization of BPSS1996 protein on the surface of bacterial cells (). Bp82, an attenuated derivative of B. pseudomallei 1026b [Citation10], was incubated with polyclonal mouse anti-BPSS1996 antiserum and stained with a fluorophore 488 tagged antibody. shows that green fluorescence was observed with Bp82, but was absent with Bp82 ∆BPSS1996, demonstrating that BPSSS1996 is localized on the bacterial surface. The BPSS1996 gene was cloned into the broad-host-range plasmid pBHR2 [Citation18] under the control of a constitutive promoter and transformed into Bp82 ∆BPSS1996. When reacted with the anti-BPSS1996 antisera and the fluorophore 488 tagged antibody, Bp82 ∆BPSS1996 (pBHR2-BPSS1996) exhibited green fluorescence while the vector only control, Bp82 ∆BPSS1996 (pBHR2), did not (). As a control, the bacteria were also incubated with BSA rather than the polyclonal BPSS1996 antisera and no florescence was detected following treatment with the secondary antibody (data not shown). The results demonstrate that the ∆BPSS1996 mutation can be complemented in trans and confirm that the BPSS1996 protein is present on the outer membrane of the bacterial cell.
Figure 8. Immunofluorescence staining of bacterial cells with polyclonal anti-BPSS1996 antisera. B. pseudomallei Bp82, Bp82 ΔBPSS1996, Bp82 ΔBPSS1996 (pBHR2-BPSS1996) and Bp82 ΔBPSS1996 (pBHR2) were reacted with a murine polyclonal anti-BPSS1996 primary antibody, washed and stained with an anti-mouse Alexa 488-conjugated secondary antibody. Top panel, phase contrast; bottom panel, green fluorescence. Bar 10 µm.
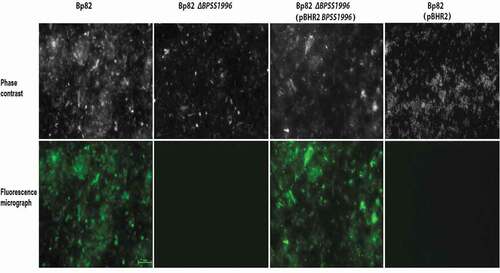
Discussion
NanoRPLC-MS/MS was utilized in this study to identify proteins produced by B. pseudomallei while inside murine macrophages. The intracellular protein profile closely resembled the gene expression of B. pseudomallei in vivo, especially as described for human immune responses characterized using sera from patients infected with B. pseudomallei [Citation25,Citation26,Citation28–Citation32,Citation45-Citation48]. We predicted that previously characterized Burkholderia proteins would be identified and serve as a positive control for our technical approach. One hundred sixty K96243 proteins were identified inside RAW264.7 cells at 12 h post-infection ( and Table S1), including some previously shown to be produced only inside host cells (Hcp1, TssM, TssA, TssB and BimA) [Citation13,Citation21–Citation24]. This result provided confidence that the bacterial proteins identified were actively produced intracellularly.
Several HSPs and OMPs were present within RAW264.7 cells at 12 h post-infection, suggesting that they may enhance pathogen survival and facilitate infection in this adverse host environment. HSPs, such as GroES, GroEL, HtpG, GrpE and DnaK, are often up-regulated under conditions of environmental stress are well represented in the B. pseudomallei intracellular proteome () [Citation25,Citation49]. These proteins are involved in the correct folding and assembly of polypeptides released from the ribosome, the transport of proteins to various locations in the cell and the degradation of aggregated or misfolded proteins. Several OMPs were also prominent in B. pseudomallei-infected macrophages (). As described above, BamC is a member of the BAM complex, an assembly of proteins involved in the assembly and insertion of beta-barrel proteins into the outer membrane. Although it is well recognized in E. coli and other bacteria [Citation37,Citation38], BamC was not demonstrated to be an abundant entity in previously reported Burkholderia proteomics studies [Citation26,Citation29,Citation31,Citation32,Citation44,Citation45,Citation47] and the significance of its enhanced production in the current study requires further investigation. Three OmpA family proteins (BPSL0999, BPSL2765 and BPSL2522) were also identified inside RAW264.7 macrophages infected with K96243 (). Previous studies demonstrated that BPSL2765 and BPSL2522 were immunogenic in B. pseudomallei-infected mice and in melioidosis patients and provided 50% protection against a lethal challenge when used as a vaccine candidate [Citation28,Citation34]. It is likely that HSPs and OMPs are released inside macrophages as a result of bacterial lysis by the 12 h timepoint, but it is also possible that bacterial OMPs are shed, or proteolytically released, while growing inside macrophages.
Several B. pseudomallei elongation factors and antioxidant enzymes were also prevalent inside RAW264.7 macrophages. The universal elongation factors EF-Tu, EF-G and EF-Ts play major roles during the elongation cycle of protein synthesis on the ribosome and they were well-represented inside infected macrophages ( and S1). Interestingly, EF-Tu also serves as a chaperone during times of environmental stress and participates in the folding of denatured proteins [Citation50]. The antioxidant enzymes peroxiredoxin (BPSL2748), FeSOD (BPSL0880), thiol peroxidase (BPSL2987) and AhpC (BPSL2096) were among the top K96243 proteins present inside macrophages ( and S1). These enzymes are involved in the detoxification of reactive oxygen species (ROS), such as hydrogen peroxide, alkyl hydroperoxides and superoxide, and promote the survival of pathogens exposed to antibacterial environments such as the host cell phagolysosome [Citation51,Citation52]. B. pseudomallei AhpC was found to be highly expressed in plasma from melioidosis survivors, but not in healthy controls [Citation31]. In addition, T cell mediated immunity to AhpC was associated with protection against disease and shown to be a correlate of survival [Citation46,Citation48] and it represents a melioidosis vaccine candidate [Citation30,Citation46,Citation48]. BPSL2748 is a putative AhpC-type peroxiredoxin, but it has not been as extensively studied as B. pseudomallei AhpC. In this study, we constructed a BPSL2748 mutant, 668 ∆L2748, and showed that it exhibited no overt decrease in virulence in a BALB/c mouse i.p. model of melioidosis ( and S1). This result implies that BPSL2748 may encode a redundant activity due to expression of other B. pseudomallei anti-oxidative enzymes ( and S1). Alternately, changes in virulence associated with the loss of BPSL2748 might be dependent on the route of infection.
The final group of B. pseudomallei proteins identified within infected RAW264.7 murine macrophages were putative exported proteins containing N-terminal signal sequences (). Proteins belonging to this group were BPSL1929, BPSS1996, BPSS1512 (TssM), BPSL2520 and BPSL1418. TssM is a deubiquitinase that is secreted by the Burkholderia type II secretion system (T2SS) [Citation15,Citation53]. It is induced inside of phagocytic cells where it downregulates the innate immune response by interfering with the ubiquitination of signaling intermediates involved in TLR-mediated NF-κB activation [Citation15,Citation24]. BPSL1929 is a hypothetical protein and BPSL1418 is a predicted peptidyl-prolyl cis-trans isomerase (PPIase), a superfamily of proteins associated with multiple biological activities and virulence [Citation54]. BPSS1996 and BPSL2520 are members of the Domains of Unknown Function (DUF) families DUF4148 and DUF2059, respectively. DUF proteins are highly conserved, but have no known biochemical activity or structural characterization [Citation55]. The BPSS1996 mutant strain constructed in this study, 668 ∆S1996, was highly attenuated in BALB/c mice infected by the i.p. route of infection (). This finding suggests that BPSS1996 is a novel virulence factor of B. pseudomallei. While this protein was identified within infected RAW264.7 cells (), it was not involved in intracellular replication and/or survival in macrophages (). This result was somewhat surprising and may indicate that BPSS1996 somehow alters the in vivo biology of macrophages [Citation56], perhaps by influencing antigen presentation capacity and/or cytokine and chemokine production, and thus hindering the host’s ability to adequately eliminate the infection. BPSS1996 is produced by B. pseudomallei in vitro () and in vivo as it is recognized by melioidosis antisera from infected NHPs () and humans [Citation45]. Schell et al. first characterized BPSS1996 as a B. pseudomallei OMP [Citation44] and fluorescence microscopy was used here to confirm that it is surface-associated ().
While DUF4148 proteins are found in both bacteria and eukaryotes, the overwhelming majority are present in members of the order Burkholderiales (Fig. S3). The B. pseudomallei K96243 genome encodes eight DUF4148 proteins (Fig. S4) and all the genes encoding these proteins are present on chromosome 2. Accessory genes, such as those involved in secondary metabolism, environmental survival and pathogenesis, are relatively common on chromosome 2 [Citation6]. Two closely related Burkholderia species, B. mallei and B. thailandensis [Citation8], possess five and eleven DUF4148 proteins, respectively (Fig. S4). B. thailandensis, an environmental saprophyte, contains a BPSS1996 ortholog (BTH_II0374), but the gene encoding this ortholog was lost by IS407A-mediated genome reduction in the host-adapted pathogen B. mallei [Citation57] (Fig. S4). The Burkholderia DUF4148 proteins are annotated as exported proteins with a ~ 21 amino acid signal peptide embedded within a conserved N-terminal domain of ~ 54 amino acids ( and S5). The B. pseudomallei DUF4148 proteins are relatively small, 90–113 amino acids, and contain C-terminal portions that display no similarities to proteins in the Pfam database [Citation58]. Very little has been published in the literature on DUF4148 proteins, but a recent publication found that the parasite Herpetomonas muscarum possesses a putative surface-exposed DUF4148 protein that is up-regulated in its fruit fly host [Citation59]. Further studies will be required to determine the function of the surface-exposed BPSS1996 protein and elucidate the role it plays in B. pseudomallei pathogenesis.
RAW264.7 proteins were also significantly impacted by the B. pseudomallei infection. We identified 274 host proteins that were either exclusively present (58 total) or absent (216 total) in K96243-infected cells, including a number of chemokines and cytokines. describes the 15 macrophage proteins most impacted by B. pseudomallei K96243 infection, nine of which are increased and six are decreased compared to the protein levels in uninfected cells. Since B. pseudomallei is an intracellular pathogen, innate immune responses of the host are involved in controlling the initial stages of infection. The mostly highly expressed factors shown in are innate immune signaling molecules including proinflammatory cytokines IL-1α, IL1-β, and G-CSF; TNF-α ligands; and chemokines. Elevated levels of these cytokines are commonly identified as major responders to B. pseudomallei infection in tissues from humans and mice infected with B. pseudomallei or stimulated with B. pseudomallei antigens [Citation60–Citation67].
In a RAW264.7 cell model of B. pseudomallei infection, Hseu et al. observed the increased production of many apoptosis-associated proteins to include several caspase enzymes and caspase-associated recruitment proteins (CARD) as well as numerous ligands of TNF, a major stimulator of apoptosis [Citation68]. Three of the nine highly expressed entities in are TNF receptor-associated proteins (encoded by TNFRSF1B, CD40, and TRAF1). However only one CARD family protein gene (Card9) and one caspase enzyme prominent in apoptosis (CASP6) are included, both of which were down-regulated (). Several other RAW264.7 proteins were down-regulated relative to uninfected cells (): IRAK1 (IL-1-receptor associated kinase), NKRF (NF-κB-repressing factor), COPS1 (COP9 signalosome complex subunit 1), and AP3B2 (AP-3 complex subunit beta-2). Since NF-κB has a central role in regulating the cellular response to infection and activates transcription of cytokines and survival functions, the down-regulation of NKRF in infected macrophages is a logical finding. Conversely, Wiersinga et al. showed that IRAK-1 transcription was downregulated at later times of infection in an experimental mouse model of melioidosis [Citation67]. This depletion of IRAK-1 mRNA agrees with studies in human volunteers injected with LPS [Citation69] and could reflect an early adaptation toward a state of immunotolerance during the course of melioidosis to dampen the overwhelming proinflammatory response. Furthermore, B. pseudomallei has mechanisms to evade the host response, e.g., relatively less reactogenic LPS compared to other gram negative pathogens, less stimulatory for TLR4 and lower acute cytokine responses [Citation61,Citation62,Citation67]. COPS1 and AP3B2 are constituents of protein complexes involved in assembling membrane proteins into transport vesicles for sorting to organelles [Citation70–Citation72]. AP3B2 is a subunit of the adaptor protein complex 3 (AP-3), which is required for sorting transmembrane proteins targeted to lysosomes and related organelles. The down regulation in expression of these proteins might disrupt the ability of a host cell to inactivate pathogens, such as B. pseudomallei, capable of intracellular survival and persistence [Citation70,Citation71,Citation73].
In addition to being a major stimulator of apoptosis, TNF-α together with IL-1β are proinflammatory cytokines expressed by PBMCs obtained from normal or B. pseudomallei -infected humans stimulated by infection or LPS, or in cells from B. pseudomallei infected mice [Citation60,Citation62,Citation63,Citation67,Citation68,Citation74]. Moreover, TNF-α, together with IL-1α and IL-18, are enhanced in expression during caspase-1 dependent macrophage death (pyroptosis) induced by B. pseudomallei [Citation64].
Thus the macrophage infection model appears to be capable of reproducing aspects of both early and late stages in B. pseudomallei infection, i.e., the early pro-inflammatory, host-protective immune responses and terminal indicators of immune suppression and cell death. In future studies we hope to determine how bacterial proteins produced inside macrophages, including BPSS1996, influence macrophage function and overall proteome content. The findings of the current study suggest that, in addition to basic questions of pathogenesis, the macrophage cell culture model of B. pseudomallei infection may be a useful supplemental tool to evaluate potential efficacy of antimicrobial countermeasures.
Supplemental Material
Download Zip (740.1 KB)Acknowledgments
We thank Zhaojing Meng and King Chan at the Laboratory of Proteomics and Analytical Technologies, Frederick National Laboratory for Cancer Research, for NanoRPLC-MS/MS technical support. We also thank David P. Fetterer, Steven Kern and Sarah Norris for statistical support. This work was supported by DTRA/JSTO-CBD under Grants CBCALL12-LS1-2-0070 and CBCALL14-AMD2-CBM-05-2-0015. Opinions, interpretations, conclusions, and recommendations are those of the authors and are not necessarily endorsed by the U.S. Army.
Disclosure statement
No potential conflicts of interest were reported by the authors.
Supplementary material
Supplemental data for this article can be accessed here.
Additional information
Funding
References
- Sprague LD, Neubauer H. Melioidosis in animals: a review on epizootiology, diagnosis and clinical presentation. J Vet Med B Infect Dis Vet Public Health. 2004;51: 305–320.
- Wiersinga WJ, Virk HS, Torres AG, et al. Melioidosis. Nat Rev Dis Primers. 2018;4:17107.
- Centers for disease control and prevention D. Possession, use, and transfer of select agents and toxins: biennial review. Final rule Fed Regist. 2012; 77(194):61083–61115.
- Johnson MM, Ainslie KM. Vaccines for the prevention of melioidosis and glanders. Curr Trop Med Rep. 2017;4(3):136–145.
- Schweizer HP. Mechanisms of antibiotic resistance in Burkholderia pseudomallei: implications for treatment of melioidosis. Future Microbiol. 2012;7(12):1389–1399.
- Holden MT, Titball RW, Peacock SJ, et al. Genomic plasticity of the causative agent of melioidosis, Burkholderia pseudomallei. Proc Natl Acad Sci. 2004;101:14240–14245.
- Ooi WF, Ong C, Nandi T, et al. The condition-dependent transcriptional landscape of Burkholderia pseudomallei. PLoS Genet. 2013;9(9):e1003795.
- Galyov EE, Brett PJ, DeShazer D. Molecular insights into Burkholderia pseudomallei and Burkholderia mallei pathogenesis. Annu Rev Microbiol. 2010;64:495–517.
- Tuanyok A, Leadem BR, Auerbach RK, et al. Genomic islands from five strains of Burkholderia pseudomallei. BMC Genomics. 2008;9:566.
- Propst KL, Mima T, Choi KH, et al. A Burkholderia pseudomallei ∆purM mutant is avirulent in immunocompetent and immunodeficient animals: candidate strain for exclusion from select-agent lists. Infect Immun. 2010;78(7):3136–3143.
- Chan KC, Issaq HJ. Fractionation of peptides by strong cation-exchange liquid chromatography. In: Zhou M, Veenstra T, editors. Proteomics for Biomarker Discovery. Totowa, NJ: Humana Press; 2013. p. 311–315.
- Wilson K. Preparation of genomic DNA from bacteria. In: Ausubel FMeditor. Current protocols in molecular biology. New York: John Wiley & Sons; 1987. p. 2.4.1–2.4.5.
- Burtnick MN, Brett PJ, Harding SV, et al. The cluster 1 type VI secretion system is a major virulence determinant in Burkholderia pseudomallei. Infect Immun. 2011;79(4):1512–1525.
- Amemiya K, Dankmeyer JL, Biryukov SS, et al. Deletion of two genes in Burkholderia pseudomallei MSHR668 that target essential amino acids protect acutely infected BALB/c mice and promote long term survival. Vaccines (Basel). 2019;7(4):196.
- Burtnick MN, Brett PJ, DeShazer D. Proteomic analysis of the Burkholderia pseudomallei type II secretome reveals hydrolytic enzymes, novel proteins, and the deubiquitinase TssM. Infect Immun. 2014;82(8):3214–3226.
- Hamad MA, Zajdowicz SL, Holmes RK, et al. An allelic exchange system for compliant genetic manipulation of the select agents Burkholderia pseudomallei and Burkholderia mallei. Gene. 2009;430:123–131.
- Logue C-A, Peak IR, Beacham IR. Facile construction of unmarked deletion mutants in Burkholderia pseudomallei using sacB counter-selection in sucrose-resistant and sucrose-sensitive isolates. J Microbiol Methods. 2009;76(3):320–323.
- Schell MA, Ulrich RL, Ribot WJ, et al. Type VI secretion is a major virulence determinant in Burkholderia mallei. Mol Microbiol. 2007;64(6):1466–1485.
- Simon R, Priefer U, Puhler A. A broad host range mobilization system for in vivo genetic engineering: tranposon mutagenesis in gram negative bacteria. Bio/Technology. 1983;1:784–791.
- Arthur JM. Proteomics. Curr Opin Nephrol Hypertens. 2003;12(4):423–430.
- Chieng S, Carreto L, Nathan S. Burkholderia pseudomallei transcriptional adaptation in macrophages. BMC Genomics. 2012;13:328.
- Shalom G, Shaw JG, Thomas MS. In vivo expression technology identifies a type VI secretion system locus in Burkholderia pseudomallei that is induced upon invasion of macrophages. Microbiology. 2007;153:2689–2699.
- Stevens MP, Stevens JM, Jeng RL, et al. Identification of a bacterial factor required for actin-based motility of Burkholderia pseudomallei. Mol Microbiol. 2005;56:40–53.
- Tan KS, Chen Y, Lim YC, et al. Suppression of host innate immune response by Burkholderia pseudomallei through the virulence factor TssM. J Immunol. 2010;184(9):5160–5171.
- Amemiya K, Meyers JL, DeShazer D, et al. Detection of the host immune response to Burkholderia mallei heat-shock proteins GroEL and DnaK in a glanders patient and infected mice. Diagn Microbiol Infect Dis. 2007;59(2):137–147.
- Felgner PL, Kayala MA, Vigil A, et al. A Burkholderia pseudomallei protein microarray reveals serodiagnostic and cross-reactive antigens. Proc Natl Acad Sci USA. 2009;106(32):13499.
- Grudniak AM, Markowska K, Wolska KI. Interactions of Escherichia coli molecular chaperone HtpG with DnaA replication initiator DNA. Cell Stress Chaperones. 2015;20(6):951–957.
- Kritsiriwuthinan K, Wajanarogana S, Choosang K, et al. Production and evaluation of recombinant Burkholderia pseudomallei GroEL and OmpA proteins for serodiagnosis of melioidosis. Acta Trop. 2018;178:333–339.
- Mariappan V, Vellasamy KM, Vadivelu J. Host-adaptation of Burkholderia pseudomallei alters metabolism and virulence: a global proteome analysis. Sci Rep. 2017;7(1):9015.
- Nithichanon A, Rinchai D, Buddhisa S, et al. Immune control of Burkholderia pseudomallei-Common, high-frequency T-Cell responses to a broad repertoire of immunoprevalent epitopes. Front Immunol. 2018;9(484). DOI:https://doi.org/10.3389/fimmu.2018.00484
- Suwannasaen D, Mahawantung J, Chaowagul W, et al. Human immune responses to Burkholderia pseudomallei characterized by protein microarray analysis. J Infect Dis. 2011;203(7):1002–1011.
- Varga JJ, Vigil A, DeShazer D, et al. Distinct human antibody response to the biological warfare agent Burkholderia mallei. Virulence. 2012;3(6):510–514.
- Woo PC, Leung PK, Wong SS, et al. groEL encodes a highly antigenic protein in Burkholderia pseudomallei. Clin Diagn Lab Immunol. 2001;8(4):832–836.
- Hara Y, Mohamed R, Immunogenic NS. Burkholderia pseudomallei outer membrane proteins as potential candidate vaccine targets. PloS One. 2009;4(8):e6496–e6496.
- Das M, Chopra AK, Cantu JM, et al. Antisera to selected outer membrane proteins of Vibrio cholerae protect against challenge with homologous and heterologous strains of V. cholerae. FEMS Immunol Med Microbiol. 1998;22(4):303–308.
- Weiser JN, Gotschlich EC. Outer membrane protein A (OmpA) contributes to serum resistance and pathogenicity of Escherichia coli K-1. Infect Immun. 1991;59(7):2252.
- Hagan CL, Kahne D. The reconstituted Escherichia coli Bam complex catalyzes multiple rounds of β-barrel assembly. Biochemistry. 2011;50(35):7444–7446.
- Webb CT, Heinz E, Lithgow T. Evolution of the β-barrel assembly machinery. Trends Microbiol. 2012;20(12):612–620.
- Kaewarpai T, Ekchariyawat P, Phunpang R, et al. Longitudinal profiling of plasma cytokines in melioidosis and their association with mortality: a prospective cohort study. Clin Microbiol Infect. 2020;26(6):783.e1-783.e8.
- Welkos SL, Klimko CP, Kern SJ, et al. Characterization of Burkholderia pseudomallei strains using a murine intraperitoneal infection model and in vitro macrophage assays. PLoS ONE. 2015;10(4):e0124667.
- Jones AL, DeShazer D, Woods DE. Identification and characterization of a two-component regulatory system involved in invasion of eukaryotic cells and heavy metal resistance in Burkholderia pseudomallei. Infect Immun. 1997;65:4972–4977.
- Teijaro JR. Cytokine storms in infectious diseases. Semin Immunopathol. 2017;39(5):501–503.
- Taylor A, Foo SS, Bruzzone R, et al. Fc receptors in antibody-dependent enhancement of viral infections. Immunol Rev. 2015;268(1):340–364.
- Schell MA, Zhao P, Wells L. Outer membrane proteome of Burkholderia pseudomallei and Burkholderia mallei from diverse growth conditions. J Proteome Res. 2011;10(5):2417–2424.
- Su YC, Wan KL, Mohamed R, et al. A genome level survey of Burkholderia pseudomallei immunome expressed during human infection. Microbes Infect. 2008;10(12):1335–1345.
- Dunachie SJ, Jenjaroen K, Reynolds CJ, et al. Infection with Burkholderia pseudomallei – immune correlates of survival in acute melioidosis. Sci Rep. 2017;7(1):12143.
- Kohler C, Dunachie SJ, Müller E, et al. Rapid and sensitive multiplex detection of Burkholderia pseudomallei-specific antibodies in melioidosis patients based on a protein microarray approach. PLoS Negl Trop Dis. 2016;10(7):e0004847.
- Reynolds C, Goudet A, Jenjaroen K, et al. T cell immunity to the alkyl hydroperoxide reductase of Burkholderia pseudomallei: a correlate of disease outcome in acute melioidosis. J Immunol. 2015;194(10):4814.
- Maleki F, Khosravi A, Nasser A, et al. Bacterial heat shock protein activity. J Clin Diagn Res. 2016;10(3):BE01–BE3.
- Caldas TD, Yaagoubi AE, Richarme G. Chaperone properties of bacterial elongation factor EF-Tu. J Biol Chem. 1998;273(19):11478–11482.
- Charoenlap N, Shen Z, McBee ME, et al. Alkyl hydroperoxide reductase is required for Helicobacter cinaedi intestinal colonization and survival under oxidative stress in BALB/c and BALB/c interleukin-10-/- mice. Infect Immun. 2012;80(3):921.
- Hofmann B, Hecht HJ, Flohé L.Peroxiredoxins. Biol Chem. 2002;383(3–4):347–364.
- Shanks J, Burtnick MN, Brett PJ, et al. Burkholderia mallei tssM encodes a putative deubiquitinase that is secreted and expressed inside infected RAW 264.7 murine macrophages. Infect Immun. 2009;77(4):1636–1648.
- Ünal CM, Steinert M. Microbial peptidyl-prolyl cis/trans isomerases (PPIases): virulence factors and potential alternative drug targets. Microbiol Mol Biol Rev. 2014;78(3):544–571.
- Mudgal R, Sandhya S, Chandra N, et al. De-DUFing the DUFs: deciphering distant evolutionary relationships of domains of unknown function using sensitive homology detection methods. Biol Direct. 2015;10:38.
- Shapouri-Moghaddam A, Mohammadian S, Vazini H, et al. Macrophage plasticity, polarization, and function in health and disease. J Cell Physiol. 2018;233(9):6425–6440.
- Song H, Hwang J, Yi H, et al. The early stage of bacterial genome-reductive evolution in the host. PLoS Pathog. 2010;6(5):e1000922–e1000922.
- El-Gebali S, Mistry J, Bateman A, et al. The Pfam protein families database in 2019. Nucleic Acids Res. 2018;47(D1):D427–D432.
- Sloan MA, Brooks K, Otto TD, et al. Transcriptional and genomic parallels between the monoxenous parasite Herpetomonas muscarum and Leishmania. PLoS Genet. 2019;15(11):e1008452–e1008452.
- Chantratita N, Tandhavanant S, Myers ND, et al. Survey of innate immune responses to Burkholderia pseudomallei in human blood identifies a central role for lipopolysaccharide. PLoS One. 2013;8(11):e81617.
- Gan YH. Interaction between Burkholderia pseudomallei and the host immune response: sleeping with the enemy?. J Infect Dis. 2005;192(10):1845–1850.
- Krishnananthasivam S, Sathkumara HD, Corea E, et al. Gene expression profile of human cytokines in response to Burkholderia pseudomallei infection. mSphere. 2017;2(2):e00121–17.
- Silva EB, Dow SW. Development of Burkholderia mallei and pseudomallei vaccines. Front Cell Infect Microbiol. 2013;3:10.
- Sun GW, Lu J, Pervaiz S, et al. Caspase-1 dependent macrophage death induced by Burkholderia pseudomallei. Cell Microbiol. 2005;7(10):1447–1458.
- Ulett GC, Ketheesan N, Hirst RG. Cytokine gene expression in innately susceptible BALB/c mice and relatively resistant C57BL/6 mice during infection with virulent Burkholderia pseudomallei. Infect Immun. 2000;68(4):2034.
- Ulett GC, Ketheesan N, Hirst RG. Proinflammatory cytokine mRNA responses in experimental Burkholderia pseudomallei infection in mice. Acta Trop. 2000;74(2):229–234.
- Wiersinga WJ, Dessing MC, van der Poll T. Gene-expression profiles in murine melioidosis. Microbes Infect. 2008;10(8):868–877.
- Hseu YC, Sung JC, Shieh BS, et al. Burkholderia pseudomallei infection induces the expression of apoptosis-related genes and proteins in mouse macrophages. J Microbiol Immunol Infect. 2014;47(5):394–398.
- van ‘T Veer C, PS VDP, MAD VZ, et al. Induction of IRAK-M is associated with lipopolysaccharide tolerance in a human endotoxemia model. J Immunol. 2007;179(10):7110.
- Dell’Angelica EC, Ohno H, Ooi CE, et al. AP-3: an adaptor-like protein complex with ubiquitous expression. Embo J. 1997;16(5):917–928.
- Odorizzi G, Cowles CR, Emr SD. The AP-3 complex: a coat of many colours. Trends Cell Biol. 1998;8(7):282–288.
- Wei N, Deng XW. Making sense of the COP9 signalosome: a regulatory protein complex conserved from Arabidopsis to human. Trends Genet. 1999;15(3):98–103.
- Ammann S, Schulz A, Krägeloh-Mann I, et al. Mutations in AP3D1 associated with immunodeficiency and seizures define a new type of Hermansky-Pudlak syndrome. Blood. 2016;127(8):997–1006.
- Lu R, Popov V, Patel J, et al. Burkholderia mallei and Burkholderia pseudomallei stimulate differential inflammatory responses from human alveolar type II cells (ATII) and macrophages. Front Cell Infect Microbiol. 2012;2:165.
- Lee MA, Liu Y. Sequencing and characterization of a novel serine metalloprotease from Burkholderia pseudomallei. FEMS Microbiol Lett. 2000;192:67–72.
- Keith KE, Oyston PC, Crossett B, et al. Functional characterization of OXA-57, a class D β-Lactamase from Burkholderia pseudomallei. Antimicrob Agents Chemother. 2005;49(4):1639.
- Almagro Armenteros JJ, Tsirigos KD, Sønderby CK, et al. Signal P 5.0 improves signal peptide predictions using deep neural networks. Nat Biotechnol. 2019;37(4):420–423.
- Dereeper A, Guignon V, Blanc G, et al. Phylogeny.fr: robust phylogenetic analysis for the non-specialist. Nucleic Acids Res. 2008;36(WebServer issue):W465–W469.
- Schuster-Böckler B, Schultz J, Rahmann S. HMM Logos for visualization of protein families. BMC Bioinformatics. 2004;5(1):7.