ABSTRACT
In eukaryotes, calcium not only is an essential mineral nutrient but also serves as an intracellular second messenger that is necessary for many physiological processes. Previous studies showed that the protein phosphatase-calcineurin protects fungi from toxicity caused by the extracellular calcium; however, little is known about how calcineurin mediates the cellular physiology process for this function. In this study, by monitoring intracellular calcium, particularly by tracking vacuolar calcium dynamics in living cells through a novel procedure using modified aequorin, we found that calcineurin dysfunction systematically caused abnormal intracellular calcium homeostasis in cytosol, mitochondria, and vacuole, leading to drastic autophagy, global organelle fragmentation accompanied with the increased expression of cell death-related enzymes, and cell death upon extracellular calcium stimuli. Notably, all detectable defective phenotypes seen with calcineurin mutants can be significantly suppressed by alleviating a cytosolic calcium overload or increasing vacuolar calcium storage capacity, suggesting toxicity of exogenous calcium to calcineurin mutants is tightly associated with abnormal cytosolic calcium accumulation and vacuolar calcium storage capacity deficiency. Our findings provide insights into how the original recognized antifungal drug target-calcineurin regulates intracellular calcium homeostasis for cell survival and may have important implications for antifungal therapy and clinical drug administration.
Introduction
Calcium (Ca2+), as an intracellular second messenger in eukaryotic cells, plays a critical role in the regulation of various cellular processes including synaptic transmission, secretion, and cytokinesis [Citation1,Citation2]. The intracellular Ca2+ concentration is strictly and precisely controlled by a sophisticated calcium homeostasis system, which is composed of various calcium channels, calcium pumps, and calcium antiporters [Citation2–4]. Overwhelming evidencesdemonstrate that calcineurin, the conserved Ca2+-calmodulin (CaM) activates protein phosphatase, is a master regulator of intracellular calcium homeostasis in fungi [Citation5]. Calcineurin is required for the regulation of cation homeostasis, morphogenesis, cell-wall integrity, and pathogenesis [Citation6]. However, the mechanism by which calcineurin regulates the optimal Ca2+ concentrations in the cytosol and in intracellular compartments such as the vacuole and mitochondria remains elusive.
When fungal cells encounter environmental stresses, a transient elevation of cytosolic calcium is induced primarily through calcium influx systems in the plasma membrane such as the high-affinity calcium uptake Cch1-Mid1 channel complex system [Citation4,Citation7]. Calcium-activated calcineurin then dephosphorylates the transcription factor Crz1, which induces its translocation to the nucleus to regulate gene expression. It has been shown that the free cytosolic calcium concentration ([Ca2+]c) must be maintained at a low, steady level within a narrow physiological range (50– 200 nM) after a sudden increase, peaking within seconds and then sharply decreasing within 30 seconds as the excess cytosolic calcium is transiently transferred to calcium stores including the endoplasmic reticulum (ER), vacuoles, and mitochondria by various calcium pumps and calcium antiporters [Citation4,Citation8–11]. In mammals, the ER, mitochondria, and lysosomes are physically and/or functionally linked [Citation12]. The role of Ca2+ signaling in mitochondria is to determine cellular survival by controlling basal mitochondrial bioenergetics and by regulating apoptosis. Moreover, the lysosomes can act as a Ca2+ store for Ca2+ release into the cytosol, thereby influencing Ca2+ homeostasis. Ca2+ signaling in lysosomes may regulate autophagy to respond to cell damage and cell stress [Citation13]. As a topological equivalent, the vacuole is the primary calcium storage organelle in fungi, containing more than 90% of total cellular calcium [Citation14]. Notably, until now there was no reliable system to probe or detect the Ca2+ concentration in fungal vacuole, probably due to its low internal pH. Vacuolar Ca2+ sequestration is performed by the collaboration of Ca2+/H+ exchangers in the Vcx family and the P-type ATPase Pmc family [Citation15,Citation16]. In contrast, vacuolar Ca2+ can be released into the cytosol through the mechanosensitive channel Yvc1 and/or transient receptor protein (TRP) channels [Citation7,Citation17–21]. Several lines of evidence in yeast-like and filamentous fungi indicate that the transcription of Pmc family members is dependent on an activated calcineurin-Crz1/CrzA complex, whereas Vcx1 activity is negatively regulated by the calcineurin complex [Citation7,Citation22–25].
In addition to being a calcium store, the fungal vacuole is responsible for the last step of autophagy. Autophagy is a highly conserved catabolic process that mediates the self-degradation of intracellular material, such as proteins, lipids, or even entire organelles. In the conserved autophagy pathway, the double-membrane autophagosome (AP) engulfs cellular components to be delivered for degradation in the lysosome/vacuole [Citation26,Citation27]. Autophagy exists at a basal level in all living cells, but it can be induced in response to various factors such as nitrogen starvation, oxidative stress, ER stress, and rapamycin drug treatment [Citation28–30]. In most of these situations, autophagy has both beneficial and harmful effects. It can function as a protective mechanism to promote cell survival in nutritional starvation and other stresses, but excessive autophagy also leads to damage to organelles and even cell death [Citation31,Citation32].
Aspergillus fumigatus is an opportunistic filamentous fungus that causes a wide spectrum of life-threatening diseases with poor treatment outcomes in immunocompromised individuals [Citation33]. Calcineurin is considered a potential drug target due to its essential roles in fungal growth and virulence [Citation34–36]. In this study, we observed the phenomenon that exogenous calcium is toxic to calcineurin-mutant fungi. By monitoring the calcium dynamics in the cytosol and intracellular calcium stores with modified forms of aequorin as a calcium reporter, we found that the calcineurin mutant exhibited abnormal calcium homeostasis and triggered autophagy and global fragmentation of the nucleus and organelles under a calcium stimulus. Additionally, the defective phenotypes of the calcineurin mutant can be significantly suppressed by alleviating cytosolic calcium overload through deletion of the Ca2+ channel cchA or by increasing vacuolar calcium storage capacity with overexpressed vacuolar P-type Ca2+-ATPase PmcA, highlighting the feedback relationship between calcineurin and CchA and the critical role of vacuoles in the detoxification of excess calcium for fungal survival. Our findings shed light on the underlying mechanism by which calcineurin regulates calcium homeostasis for cell survival, and these results provide a rationale for combination therapy of calcium and calcineurin inhibitors to combat human fungal pathogens.
Results
Extracellular calcium is toxic to A. fumigatus calcineurin mutants
Calcineurin is a highly conserved Ca2+-calmodulin-dependent protein phosphatase that plays a central role in morphological development and cation homeostasis in fungi [Citation37,Citation38]. To further characterize the functions of calcineurin in Ca2+ signaling transduction, we constructed deletion mutants of the catalytic subunit CnaA and the regulatory subunit CnaB by CRISPR-Cas9 technique in A. fumigatus (Figure S1). Morphological analysis in solid and liquid minimal media showed that the ΔcnaA mutant displayed defective morphological phenotypes including reduced hyphal growth and abnormal branching (). Notably, the abnormal hyphal phenotypes of the ΔcnaA mutant were exacerbated in the presence of 10 mM CaCl2, i.e., swollen hyphae tips, whereas no obvious differences were observed in the cnaAR complementary strain. Moreover, the ΔcnaA mutant showed a significant decrease in mycelial production compared to that of the wild-type at indicated time-points when cultured in media with or without 10 mM CaCl2 (). Interestingly, the calcineurin mutants ceased growth when incubated in the presence of calcium after 72 h, suggesting that the addition of calcium is toxic to the ΔcnaA mutant (). To confirm this toxicity, we cultured the wild-type and ΔcnaA strains in liquid minimal media (MM) with or without 10 mM CaCl2 for 96 h. Subsequently, the homogenized mycelia were inoculated into fresh liquid MM for another 48 h. The wild-type strains pretreated with or without calcium grew relatively well after transferring to fresh liquid MM. In contrast, the growth of the ΔcnaA mutant pretreated with calcium was dramatically decreased compared to that of the untreated ΔcnaA mutant ( and ), confirming that the relatively low levels of extracellular calcium significantly inhibited the growth of the calcineurin mutant. Moreover, the ΔcnaB mutant exhibited identical phenotypes to those of the ΔcnaA mutant under the conditions we tested (Figure S2). Taken together, these data suggest that extracellular calcium is toxic to A. fumigatus calcineurin mutants and that calcineurin is essential for the survival of A. fumigatus in response to calcium stimuli. Given that ΔcnaB displayed the identical phenotype to the ΔcnaA mutant in the presence of calcium and previous study showed that both of catalytic subunit CnaA and regulatory subunit CnaB are indispensable for the function of calcineurin [Citation39], we therefore chose the ΔcnaA mutant for subsequent experiment to investigate the role of calcineurin in mediating the cellular physiology process.
Figure 1. Calcium is toxic to calcineurin mutants
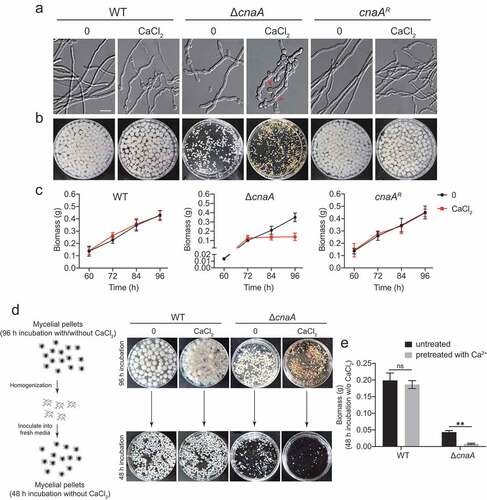
ΔcnaA mutant exhibits increased cytosolic and vacuolar calcium accumulation in response to extracellular calcium stimuli
Given that calcineurin is critical for regulating A. fumigatus survival in response to extracellular calcium, to further explore the mechanisms by which calcineurin regulates calcium homeostasis in A. fumigatus, we constructed wild-type and calcineurin mutants expressing codon-optimized aequorin to perform real-time monitoring of the dynamics of free Ca2+ concentration [Ca2+] in living hyphal cells [Citation40]. Upon treatment with 10 mM CaCl2, the [Ca2+]c (the free Ca2+ concentration in cytosol) in the wild-type strain transiently increased from a basal resting level of approximately 0.1 μM to a peak concentration of 0.63 μM and then gradually returned to a stable resting level (); thus, the amplitude of [Ca2+]c between the peak and the resting status was 0.55 μM. In comparison, the basal resting level of [Ca2+]c increased from 0.1 μM to 0.2 μM (~2-fold increase) and amplitude of [Ca2+]c increased from 0.55 μM to 0.73 μM (~33% increase) in the ΔcnaA mutant under the same conditions, suggesting that cytosolic calcium homeostasis was affected by the deletion of cnaA. Next, we wondered whether the calcium equilibrium in mitochondria would be affected by calcineurin mutants. To test this possibility, we compared the [Ca2+]mt (the free Ca2+ concentration in mitochondria) using a modified aequorin (Mt-Aeq) protein fused with a mitochondrial signal peptide. As shown in , the ΔcnaA mutant displayed a 20% increase in the basal resting level and a similar amplitude response of [Ca2+]mt compared to the wild-type and cnaA complementing strains, suggesting that the deletion of cnaA only has a minor effect on mitochondrial calcium homeostasis. Next, we wondered in fungi, the vacuole might be a major intracellular Ca2+ store that plays a role in the regulation of Ca2+ homeostasis. However, until now, no reliable detection approach has been available for the real-time monitoring of the vacuolar-free Ca2+ concentration ([Ca2+]vac) in fungi. To set up this system, we fused a guide sequence encoding the vacuolar lumen marker protein carboxypeptidase Y (CpyA) at the N-terminus of aequorin to generate the fusion protein CpyA-Aeq [Citation41]. To verify the localization of the guided protein CpyA in A. fumigatus, we tagged CpyA with GFP and RFP at the C-terminus under control of gpdA promoter, respectively. As shown in , the localization of CpyA-GFP fusion protein shows a vacuolar pattern. To further verify this localization, we tagged the previously reported vacuole membrane-localized protein PmcA (the homolog of yeast P-type Ca2+-ATPase Pmc1) with GFP in the CpyA-RFP background. Colocalization analysis showed that GFP-PmcA surrounded the CpyA-RFP (), demonstrating that CpyA acts as a typical vacuole lumen protein. Next, to further confirm the feasibility of the [Ca2+]vac measurement system, we generated the overexpression strains of the vacuolar P-ATPases PmcA and PmcB, which are responsible for calcium influx into the vacuole by consuming ATP, and assessed the calcium levels in the vacuole. As shown in Figure S3, overexpressing pmcA/B remarkably elevated the basal resting level of [Ca2+]vac and enhanced calcium influx into the vacuole compared with the wild-type strain, confirming the reliability of this vacuolar calcium measurement method. Thus, we employed the CpyA-Aeq system to monitor the free Ca2+ concentration of vacuoles. Interestingly, the basal resting [Ca2+] level in vacuoles (0.65 μM) prior to stimuli was significantly greater than that in the cytosol and mitochondria (), confirming that the vacuole is a major intracellular calcium store in A. fumigatus. Notably, in the ΔcnaA cells, the basal resting level of [Ca2+]vac was 0.8 μM (~23% increase) and the [Ca2+]vac amplitude was 4.6 μM (~5-fold increase) compared to that of in wild-type cells upon exposure to 10 mM Ca2+ (). Collectively, these data suggested that the ΔcnaA mutant exhibits a dramatic increase in vacuolar Ca2+ influxes and induces excessive vacuolar Ca2+ accumulation.
Figure 2. Deletion of cnaA affects intracellular calcium accumulation in response to extracellular calcium stimuli
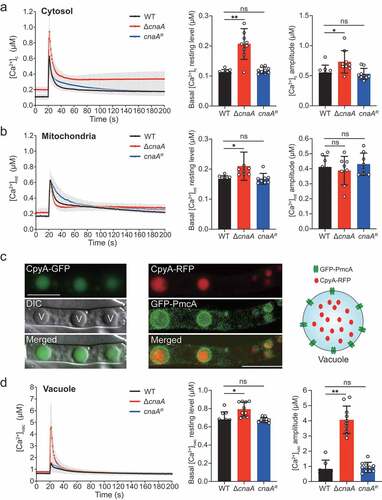
Extracellular calcium induces vacuolar fragmentation and autophagy in the ΔcnaA mutant
Next, we wondered whether the excessive vacuolar Ca2+ accumulation in the ΔcnaA mutant would cause any changes of vacuolar morphology. As shown in , fluorescence microscopy analysis showed that wild-type and ΔcnaA cells had similar oval-shaped mature vacuoles as indicated by CpyA-GFP when cultured in liquid minimal media for 36 h with or without the addition of calcium. Notably, when incubation time was prolonged to 60 h, the ΔcnaA mutant showed highly fragmented fluorescence patterns of vacuoles in the presence of calcium (), even at a calcium concentration as low as 0.1 mM (Figure S4A), suggesting that the addition of lower concentrations of calcium may result in calcium toxicity in the ΔcnaA mutant under in vitro laboratory condition. In comparison, in the absence of calcium treatment, the vacuolar morphologies of ΔcnaA mutants showed no detectable differences compared to the parental strain. These data indicate that significant vacuolar fragmentation occurs in the ΔcnaA mutant when grown in liquid minimal media containing extra calcium. Because the vacuole is the major site for macromolecular degradation through autophagy, to examine whether the deletion of cnaA would trigger autophagy under extracellular calcium condition, we generated double-labeled strains with the autophagy marker Atg8 tagged at its N-terminus with GFP and the vacuole marker CpyA fused with RFP and monitored these in the ΔcnaA and wild-type backgrounds. As shown in , GFP-Atg8 fusion proteins show a punctate pattern that is proximal to the CpyA-RFP-labeled vacuoles in the wild-type and ΔcnaA strains when incubated for 36 h in the presence or absence of calcium. However, when cultures were stimulated by the addition of CaCl2 for 60 h, the green fluorescence patterns of GFP-Atg8 overlapped with the CpyA-RFP-labeled vacuoles (). Furthermore, Western blotting showed that the ΔcnaA strain displayed increased cleavage of GFP from the GFP-Atg8 fusion protein compared to the wild-type cells when cultured for 60 h under the control of the constitutive gpdA promoter () or the native atg8 promoter (Figure S4B-S4C) in the presence of calcium. In addition, cleavage of GFP was not significantly exacerbated when media were supplemented with other divalent metal cations including magnesium, zinc, iron, and copper (Figure S4D), suggesting that the autophagy was specifically induced by calcium. Next, to examine the contribution of autophagy to Ca2+ toxicity in the ΔcnaA mutant, we deleted the classic autophagy-dependent gene atg2, which encodes a peripheral membrane protein for autophagic vesicle formation [Citation42,Citation43], in the ΔcnaA background. As shown in , GFP-Atg8 consistently exhibited a punctate pattern and did not co-localize with the vacuolar marker CpyA-RFP in the ΔcnaAΔatg2 mutant. Furthermore, Western blotting showed that no (or an undetectable level of) GFP was cleaved from GFP-Atg8 fusion protein in the ΔcnaAΔatg2 double mutant compared with the ΔcnaA mutant (), suggesting that autophagy was suppressed in the ΔcnaAΔatg2 mutant. Notably, as shown in , the ΔcnaAΔatg2 mutant displayed a delay in growth cessation (at 84 h) compared to that of the ΔcnaA mutant (at 72 h) under calcium treatment conditions (). However, the ΔcnaAΔatg2 mutant pretreated with or without calcium showed a comparable biomass production compared to that of the ΔcnaA mutant after homogenization and transfer to fresh liquid MM, suggesting that the deletion of atg2 did not prevent the cell death induced by a lack of cnaA after culture (), indicating that Atg2 is required for autophagy but not Ca2+ toxicity in the calcineurin-deficient mutant upon calcium stimuli. Taken together, these data suggest that a lack of CnaA results in specific calcium-induced autophagy in A. fumigatus.
Figure 3. The loss of CnaA causes vacuolar fragmentation and atg2-related autophagy in the presence of excess calcium
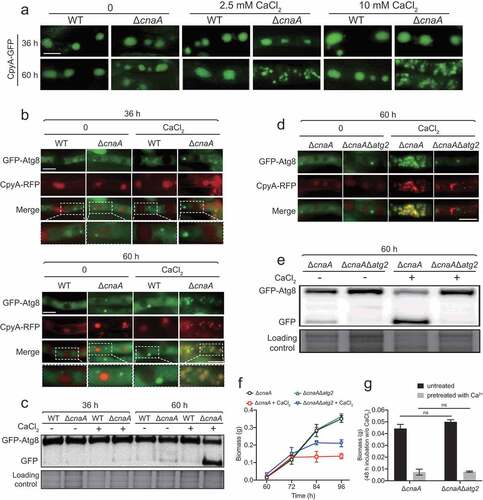
Lack of the calcium channel CchA alleviates the calcium toxicity-related phenotypes in the cnaA null mutant
Our previous studies in a model fungus A. nidulans indicated that calcineurin negatively regulates CchA (the voltage-gated Ca2+ channel component of high-affinity calcium influx system) upon calcium uptake in response to extracellular calcium; the deletion of cchA suppressed the hypersensitivity to external calcium in calcineurin mutants [Citation44]. We hypothesized that a similar situation would occur in A. fumigatus. As expected, cchA deletion partially suppressed the colony radial growth defects and hyperbranching polarity growths in cnaA deletion mutants with or without calcium treatment (Figure S5). Notably, a lack of CchA significantly rescued the defective hyphal growth of the cnaA mutant in calcium treatment conditions ( and ). The detection of calcium dynamics in living cells showed that the [Ca2+]vac amplitude and the basal resting [Ca2+]c of the ΔcnaAΔcchA mutant were approximately 53% and 20% lower, respectively, than those in the ΔcnaA mutant ( and ), suggesting that calcineurin mediates cytosolic and vacuolar calcium homeostasis by negatively regulating CchA. Accordingly, the colocalization of GFP-Atg8 and CpyA-RFP (vacuolar marker) and the vacuolar fragmentation were not observed in the ΔcnaAΔcchA mutant (). Moreover, Western blots showed that the ΔcnaAΔcchA mutant exhibited an autophagy level similar to that of the wild-type strain (), suggesting that autophagy in response to external calcium is inhibited when cchA is deleted in the ΔcnaA mutant. These data suggest that a lack of the calcium channel CchA alleviates the calcium toxicity-related phenotypes in the cnaA null mutant.
Figure 4. Deletion of cchA alleviates the calcium toxicity-related phenotypes in the ΔcnaA mutant
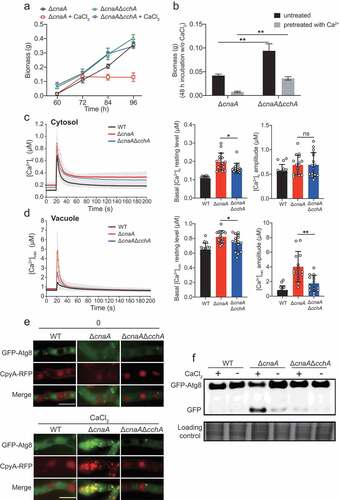
Lack of CchA did not restore the virulence of the ΔcnaA mutant in a mouse infection model
The aforementioned data demonstrated that the absence of CchA in the ΔcnaA mutant partially suppressed the growth defects of A. fumigatus in vitro. Next, we further tested whether the ΔcnaAΔcchA mutant would affect virulence in in vivo animal models. As shown in , immunocompromised ICR mice were infected with fresh conidia, homogenized hyphae, or luciferase-probed related strains by an intratracheal route. After two weeks, the mice infected with wild-type A. fumigatus display high mortality, whereas both the ΔcnaAΔcchA and ΔcnaA mutants caused low mortality (). Statistical analysis showed that there was no significant difference between ΔcnaAΔcchA and ΔcnaA in survival rates for either the conidia or the homogenized hyphal infection model. Histopathological examinations and live animal imaging showed that the lungs from mice inoculated with the wild-type fungus displayed aggressive fungal growth and luminescence signals around the lungs ( and ). In contrast, there was no detectable fungal growth or luminescence signals in the lungs of mice infected with the ΔcnaAΔcchA or ΔcnaA mutants, the differences in virulence are likely due to the different immune systems between these two infection models. Together, these data suggest that the lack of CnaA results in the loss of virulence in immunocompromised mice, and the deletion of cchA did not restore the virulence in the mouse model.
Figure 5. Deletion of cchA in the ΔcnaA mutant did not restore the virulence in a mouse infection model
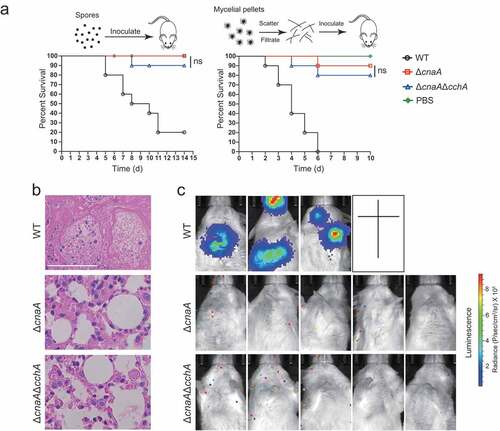
Overexpressing P-type Ca2+-ATPase PmcA significantly rescued the calcium toxicity-related phenotypes in the ΔcnaA mutant
In fungi, the vacuole serves as the major Ca2+ store and plays a critical role in the detoxification of cytoplasmic Ca2+. Vacuolar calcium equilibrium is maintained by the P-type Ca2+-ATPase Pmc family and the H+/Ca2+ exchanger Vcx family, which are regulated by calcineurin [Citation4]. In line with this information, the ΔcnaA mutant exhibited lower expression levels of pmcA and pmcC compared to those of the wild-type, whereas the transcript level of vacuolar H+/Ca2+ exchanger family members, especially vcxB, vcxD, and vcxE, was upregulated in A. fumigatus (Figure S6), demonstrating that calcineurin positively regulates the P-type Ca2+-ATPase Pmc family while negatively regulating the H+/Ca2+ exchanger Vcx family. Given the calcium toxicity-related phenotypes observed in the ΔcnaA mutant, we hypothesized that the upregulation of H+/Ca2+ exchanger Vcx family members in the ΔcnaA mutant would be insufficient to detoxify excess cytoplasmic calcium in response to external calcium, and thus a ΔcnaAOE::pmcA strain overexpressing PmcA, the yeast P-type Ca2+-ATPase Pmc1 homolog in A. fumigatus, under the control of the strong constitutive promoter PgpdA was used in the ΔcnaA background to test whether the defective phenotypes in the ΔcnaA mutant would be suppressed. qRT-PCR analysis confirmed that pmcA was highly overexpressed in the ΔcnaA mutant (Figure S3). Further live-cell detection of [Ca2+]vac showed that the ΔcnaAOE::pmcA strain displayed an elevated basal resting level of [Ca2+]vac and had an enhanced [Ca2+]vac amplitude compared to that of the ΔcnaA mutant (), indicating that calcium was effectively transported from the cytoplasm to vacuoles by PmcA. Additionally, autophagy assays showed that the ΔcnaAOE::pmcA strain displayed reduced autophagy compared to that of the ΔcnaA mutant in response to external calcium stimuli ( and ). Moreover, the overexpression of pmcA in the cnaA mutant markedly suppressed the growth cessation in calcium treatment conditions (). The ΔcnaAOE::pmcA strain pretreated with or without calcium (96 h) showed dramatically increased biomass production compared to that of the ΔcnaA mutant after homogenization and transfer to fresh liquid MM (). Thus, these data suggested that overexpressing P-type Ca2+-ATPase PmcA partially rescues calcium toxicity-related phenotypes in the ΔcnaA mutant.
Figure 6. Overexpressing P-type Ca2+-ATPase PmcA in the ΔcnaA mutant significantly rescues calcium toxicity-related phenotypes
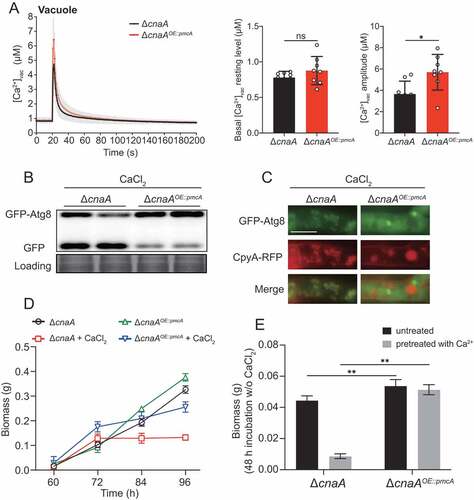
The ΔcnaA mutant exhibits global fragmentation of nuclei and organelles upon calcium stimuli
To further dissect the molecular mechanisms operating in the A. fumigatus ΔcnaA mutant upon in vitro calcium stimuli, we performed proteomic comparison analysis using tandem mass tags (TMTs) and liquid chromatography tandem MS (LC/MS-MS) and compared wild-type and ΔcnaA strains grown in the presence of extracellular calcium for 60 h. Proteins with change ratios significantly different from general protein variation (1.50 < ΔcnaA/wild-type < 0.50) were selected for gene ontology (GO) annotation and enrichment analysis. As shown in Table S3, the expression levels of 618 and 218 proteins were increased and decreased, respectively, in the ΔcnaA mutant compared to the wild-type strain. Most increased abundance of proteins were involved in metabolic processes of biomolecules, especially in catabolism and proteolysis (Figure S7A). In contrast, most of proteins with decreased abundance were associated with biosynthesis and/or the transport of biomolecules and inorganic ions (Figure S7B). Kyoto Encyclopedia of Genes and Genomes (KEGG) annotation revealed that the proteins with increased abundance were primarily associated with various amino acid metabolism pathways, especially tyrosine, beta-alanine, valine, and leucine (), whereas proteins with decreased abundance mainly focused on biosynthesis-related pathways including ribosome (protein synthesis), thiamine metabolism, and steroid biosynthesis. Of note, proteins with increased abundance in the ΔcnaA mutant included 23 peptidases, 18 hydrolases, 4 nucleases, 3 proteases, 3 autophagy-related proteins, and 1 metacaspase (), which may lead to global protein degradation and cause apoptosis/apoptotic-like programmed and/or autophagic cell death in ΔcnaA cells under calcium treatment. Thus, we hypothesized that CnaA-involved cellular calcium equilibrium may regulate whole-cell organelle networks. To further assess whether a lack of CnaA would induce abnormal organelle and nuclear morphology with calcium treatment, we probed the nucleus (RFP-H2A), endoplasmic reticulum (Erg11A-GFP), Golgi apparatus (RFP-PHOSBP), and mitochondria (MrsA-RFP). As predicted, fluorescence observation showed that these organelles displayed fragmentation that was comparable to that of the vacuole in the ΔcnaA mutant after calcium treatment for 60 h, suggesting that the apoptosis/apoptotic-like and/or autophagic cell death may occur (). A similar phenomenon was observed in A. fumigatus cells treated with the calcineurin inhibitor FK506 (). The biomass production of the wild-type strain pretreated with FK506 and calcium (96 h) was markedly compared to that of the wild-type strain merely pretreated with FK506 after homogenization and transfer to fresh liquid MM (). Taken together, these data suggested that global nuclear and organelle fragmentation may contribute to calcium toxicity in mutants lacking functional calcineurin.
Figure 7. ΔcnaA mutant shows global fragmentation of nuclei and organelles upon calcium stimuli
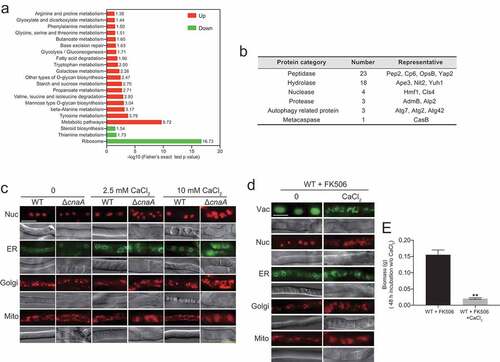
Materials and methods
Strains, media, and culture conditions
A. fumigatus strains used in this study was listed in the supplementary data Table S1. Strains were routinely grown in the following media: minimal media (MM) containing 1% glucose, 2% agar, trace elements and 20 × salt solution, as described previously [Citation45]. MM supplemented with 5 mM uridine and 10 mM uracil were used for uracil and uridine auxotroph strains. The recipe for liquid glucose minimal media is identical to that for MM, except without agar. All strains were cultured at 37°C.
Quantification of biomass production
105 conidia were incubated in 100 ml of MM with or without 10 mM CaCl2 at 37°C in a rotary shaker at 200 rpm for 60, 72, 84, and 96 h. Biomass curves were drawn after mycelial pellets were collected, dried, and weighed.
Assessment of hyphal survival
The related strains were grown in liquid MM with or without 10 mM CaCl2 with 220 rpm shaking at 37°C for 96 h. Subsequently, the mycelium pellets of each strain were crushed and homogenized into hyphae fragments using tissue grinding and filtered with lens-cleaning paper in sterile ddH2O. The optical density at 600 nm (OD600) of each group was finally adjusted to 0.2. Then, 100 μl of suspension containing hyphae fragments was inoculated into liquid MM for 48 h via shake cultivation, and the biomass was weighed and compared.
Constructions for plasmids
For the construction of complementary cnaA/cnaB, p-zero-hph-cnaA plasmid was generated as follows: the selectable marker hph from PAN7-1 was amplified by PCR using the primers hph-F and hph-R and then cloned into the pEASY-Blunt vector (TransGen Biotech), generating the plasmid p-zero-hph. The primers cnaA/cnaB-notI-F and cnaA/cnaB-notI-R were used to generate a fragment that includes the promoter sequence, the complete ORF, and the 3ʹUTR of cnaA/cnaB. This fragment was then cloned into the NotI site of p-zero-hph to generate the plasmid p-zero-hph-cnaA/cnaB.
For measuring the Ca2+ level in the cytosol and mitochondria, the plasmids pAMA1-PgpdA-Aeq and pAMA1-PgpdA-mt-Aeq were generated as follows: PCR was performed using the primers Ama1-BamHI-gpd-F and Ama1-BamHI-trpC-R to amplify PgpdA-Aeq-Ttrp and PgpdA-mt-Aeq-TtrpC fragments from the existing plasmids pAEQS1-15 and pAEQm [Citation40,Citation46,Citation47]. The fragments were subcloned into the BamHI site of the plasmid prg3-AMAI-NotI to generate pAMA1-PgpdA-Aeq and pAMA1-PgpdA-mt-Aeq, respectively.
For monitoring the calcium level in the vacuole, the plasmid pAMA1-PgpdA-CpyA-Aeq was generated as follows: PCR was performed using the primers ClaI-cpyA-R and ClaI-cpyA-F to generate a cpyA’ ORF fragment. The fragment was subcloned into the ClaI site of pBARGPE, which has a PgpdA promoter in front of its ClaI site, to generate the plasmid pBARGPE-PgpdA-cpyA. The PgpdA-cpyA fragment was amplified from the pBARGPE-PgpdA-cpyA with Ama1-BamHI-gpd-F and CpyA-linker-R primers and then fused with Aeq-TrpC fragment generated using Linker-Aeq-F and Ama1-BamHI-trpC-R to yield fusion fragment PgpdA-cpyA-Aeq. The pAMA1-PgpdA-CpyA-Aeq plasmid was generated by ligating PgpdA-cpyA-Aeq into prg3-AMAI-NotI.
pAMA1-PgpdA-CpyA-GFP/RFP was generated as follows: Fusion PCR using primers Ama1-BamHI-gpd-F and Ama1-BamHI-GFP/RFP-R, was performed to fuse the PgpdA-cpyA with GFP/RFP fragment amplified by primers Linker-GFP/RFP-F and Ama1-BamHI-GFP/RFP-R. Then, the fusion fragment was ligated into the BamHI site of the plasmid prg3-AMAI-NotI, generating pAMA1-PgpdA-CpyA-GFP/RFP.
For indicating the vacuole, the plasmid pAMA1-PgpdA-CpyA-GFP/RFP was generated as follows: Fusion PCR using primers Ama1-BamHI-gpd-F and Ama1-BamHI-GFP/RFP-R, was performed to fuse the PgpdA-cpyA with GFP/RFP fragment amplified by primers Linker-GFP/RFP-F and Ama1-BamHI-GFP/RFP-R. Then, the fusion fragment was ligated into the BamHI site of the plasmid prg3-AMAI-NotI to generate pAMA1-PgpdA-CpyA-GFP/RFP.
For visualization of cell nucleus, the pAMA1-PgpdA-RFP-H2A was generated as follows: The PgpdA-RFP-H2A fragment was amplified from the pBARGPE-PgpdA-RFP-H2A with primers Ama1-BamHI-gpd-F and Ama1-BamHI-trpC-R and then was subcloned into the BamHI site of the plasmid prg3-AMAI-NotI to generate pAMA1-PgpdA-RFP-H2A.
For visualization of ER, the plasmid pAMA1-PgpdA-erg11A-GFP was generated as follows: using primers Ama1-BamHI-gpd-F and Erg11A-R, the PgpdA-erg11A fragment was amplified from the CX23 [Citation48]. Using primers Erg11A-GFP-F and Ama1-BamHI-GFP-R, the GFP was amplified. Then, PgpdA-erg11A and GFP were fused together with primers Ama1-BamHI-gpd-F and Ama1-BamHI-GFP-R. Subsequently, the fusion fragment was subcloned into the BamHI site of the plasmid prg3-AMAI-NotI to generate pAMA1-PgpdA-erg11A-GFP.
For visualization of mitochondria, the plasmid pAMA1-MrsA-RFP was generated as follows: Using primers Ama1-BamHI-MrsA-F and MrsA-R, the MrsA fragment containing mrsA promoter and ORF without stop codon was amplified from the A. fumigatus genomic DNA (gDNA). The RFP was amplified with primers MrsA-RFP-F and Ama1-BamHI-RFP-R. Then, MrsA fragment and RFP were fused together with primers Ama1-BamHI-MrsA-F and Ama1-BamHI-RFP-R. Subsequently, the fusion fragment was subcloned into the BamHI site of the plasmid prg3-AMAI-NotI to generate pAMA1-MrsA-RFP.
For visualization of Golgi apparatus, pAMA1-PgpdA-mRFP-PHOSBP was generated as follows: The pleckstrin homology domain of the human oxysterol binding protein (PHOSBP) was PCR-amplified with primers gpdA-RFP-F and BamHI-PHOSBP-R and then fused with PgpdA promoter (PCR-amplified with Ama1-BamHI-gpd-F and Gpd-R) using primers Ama1-BamHI-gpd-F and BamHI-PHOSBP-R. The fusion fragment was subcloned into BamHI site of the plasmid prg3-AMAI-NotI to pAMA1-PgpdA-mRFP-PHOSBP.
For measuring the level of autophagy, plasmid pAMA1-PgpdA-GFP-Atg8 overexpressing GFP-Atg8 was generated as follows: PCR, using the joint primers Ama1-BamHI-gpd-F/Gpd-GFP-F/Atg8-F and Gpd-R/Atg8-GFP-R/Ama1-BamHI-Atg8-R, were performed to generate PgpdA promoter/GFP/Atg8(ORF) fragments. Next, the fusion fragments PgpdA-GFP-Atg8 were generated by fusion PCR with primers Ama1-BamHI-gpd-F and Ama1-BamHI-Atg8-R. The fusion fragments were subcloned into the BamHI site of the plasmid prg3-AMAI-NotI to generate pAMA1-PgpdA-GFP-Atg8. The plasmid pAMA1-Patg8-GFP-Atg8 expressing GFP-Atg8 under atg8 native promoter was generated as follows: PCR, using the joint primers Ama1-Bam1-atg8(p)-F/Atg8(p)-GFP-F/and Atg8-promoter-R/Ama1-BamHI-Atg8-R, was used to generate atg8 promoter/GFP-Atg8 fragments. Next, the two fragments were fused together with primers with primers Ama1-Bam1-atg8(p)-F and Ama1-BamHI-Atg8-R. The fusion fragment was subcloned into the BamHI site of prg3-AMAI-NotI to generate pAMA1-Patg8-GFP-Atg8.
For assessing the co-localization of CpyA-RFP and GFP-Atg8, the plasmid pAMA1-PgpdA-CpyA-RFP-PgpdA-GFP-Atg8 was generated as follows: The fragment PgpdA-CpyA-RFP/PgpdA-GFP-Atg8 was amplified with primers pairs Ama1-BamHI-gpd-F and TAA-RFP-R/RFP-gpdA-F and Ama1-BamHI-Atg8-R. Next, the two fragments were subcloned into prg3-AMAI-NotI with the One Step Cloning Kit (Vazyme, C113) forming pAMA1-PgpdA-CpyA-RFP-PgpdA-GFP-Atg8.
For overexpressing pmcA/pmcB, plasmid pBARGPE-hph-PgpdA-pmcA/pmcB overexpressing pmcA/pmcB were generated as follows: PCR, using the joint primers ClaI-pmcA/pmcB-F and ClaI-pmcA/pmcB-R, were used to generate pmcA/pmcB fragment that include the complete ORF and 3ʹUTR of the indicated genes. Next, the three fragments were, respectively, subcloned into the ClaI site of the plasmid to generate overexpression plasmids pBARGPE-hph-PgpdA-pmcA/pmcB, which contain a selective marker gene hph to resist hygromycin.
For amplifying the repair template gfp-hph, the plasmid p-zero-gfp-hph was generated as follows: the selectable marker hph was amplified by PCR using the primers HPH-F and HPH-R, and then cloned into the pEASY-Blunt vector, generating a plasmid p-zero-hph. Primers NOTI-gfp-F and NOTI-gfp-R were used to generate a GFP fragment. This fragment was then cloned into the NotI site of p-zero-hph to generate p-zero-gfp-hph.
For amplifying the repair template ptrA-PgpdA/ptrA-PgpdA-gfp, the plasmid p-zero-ptrA-PgpdA-gfp was generated as follows: the selectable marker ptrA was amplified by PCR using the primers ptrA-F and ptrA-R, and then cloned into the pEASY-Blunt vector, generating a plasmid p-zero-ptrA. Next, the plasmid was linearized with primer zero-F and zero-R. PCR, using primers zero-PgpdA-F/PgpdA-GFP-F and PgpdA-R/zero-GFP-R, were used to amplify PgpdA promoter/gfp fragments. The two fragments were fused with primers zero-PgpdA-F and zero-GFP-R, generating PgpdA-gfp fragment. Finally, the fusion fragment was subcloned into the linearizing p-zero-ptrA, yielding the plasmid p-zero-ptrA-PgpdA-gfp.
For amplifying the repair template hph-PgpdA, the plasmid p-hph-PgpdA was generated as follows: PCR, using primer NotI-PgpdA-F and NotI-TtrpC-R, was used to amplify gpdA promoter (PgpdA). Then, the gpdA promoter was ligated into the NotI site of p-zero-HPH, generating p-hph-PgpdA.
For expressing the N-terminus (300 aa) of CchA, the plasmid pAMA1-PgpdA-GFP-CchAN300 was generated as follows: The PgpdA-GFP fragment was obtained by PCR with primers, Ama1-BamHI-gpd-F and CchA-GFP-R from p-zero-ptrA-PgpdA-gfp; The CchAN300 fragment containing 900 bp of cchA ORF 5ʹ was amplified by PCR with primer CchA-F and Ama1-BamHI-CchAN300-R. Then, the two fragments were subcloned into prg3-AMAI-NotI with the One Step Cloning Kit (Vazyme, C113), generating the plasmid pAMA1-PgpdA-GFP-CchAN300.
For live animal bioluminescence imaging, the plasmid pBARGPE-pyr4-PgpdA-Luc expressing Luciferase was generated as follows: PCR, using the joint primers ClaI-Luc-F and ClaI-Luc-R, were used to generate luc (Luciferase gene) ORF. Next, the Luc fragments was subcloned into the ClaI site of the plasmid pBARGPE-pyr4, yielding pBARGPE-pyr4-PgpdA-Luc.
All the primers and primer annotations were given in supplementary data Table S2.
Gene-editing with MMEJ-CRISPR in A. fumigatus, transformation and strain verification
For editing the A. fumigatus gene, the MMEJ-CRISPR system was used as described in our previous published papers [Citation45,Citation49]. sgRNA targeted to the appropriate site of the target gene was synthesized in vitro using the MEGAscript T7 Kit (Life Technologies, AM1333). The corresponding repair template (DNA) with microhomology arms was amplified by PCR. Then, the repair template fragments and sgRNA were cotransformed into a Cas9-expressing A. fumigatus recipient strain. The primers and annotations for sgRNAs and repair templates are listed in Table S2. Transformation procedures were performed as previously described. Transformants were selected in medium lacking uridine or uracil or in the presence of 150 μg ml−1 hygromycin B (Sangon) or 0.1 μg ml−1 pyrithiamine (Sigma). For the recycling usage of the selectable marker pyr4, 1 mg ml−1 5-FOA was used for screening recipient strains. All primers used are listed in the supplementary data Table S2. All transformant isolates were verified by diagnostic PCR analysis using mycelia as the source of DNA. Primers were designed to probe upstream and downstream of the expected cleavage sites as labeled in Figure S1D.
Microscopy observation
For microscopic observation of hyphae morphology, fresh conidia were inoculated onto sterile glass coverslips overlaid with 1 ml of liquid glucose media with or without 10 mM CaCl2. Strains were cultivated on the coverslips at 37°C for 14 h before observation. The coverslips with hyphae were gently washed with PBS three times. Differential interference contrast (DIC) and green/red fluorescent images of the cells were collected with a Zeiss Axio Imager A1 microscope (Zeiss, Jena, Germany). To observe the fluorescence localization of GFP/RFP-tagged proteins, strains were grown in liquid medium at 37°C with shaking at 200 rpm for indicated time, and then the mycelium pellet sandwiched between the microscope slide and coverslips was observed directly by fluorescence microscopy.
Measurement of the free Ca2+ concentration ([Ca2+])
Measurement of the free Ca2+ concentration was performed as described previously with some modifications [Citation40,Citation50]. The strains expressing Aeq/Mt-Aeq/CpyA-Aeq were cultured for 2 days to form fresh spores. The spores were filtered through nylon cloth and washed 10 times in distilled deionized water. One million (106) spores in 100 μl liquid MM were inoculated into each well of a 96-well microtiter plate (Thermo Fischer). The plate was incubated at 37°C for 24 h. The medium was then removed gently with a pipette, and the cells in each well were washed twice with 150 µl PGM (20 mM PIPES pH 6.7, 50 mM glucose, 1 mM MgCl2). Aequorin was reconstituted by incubating mycelia in 100 μl PGM containing native coelenterazine (2.5 μM) (Sigma-Aldrich, C-7001) at 4°C for 4 h in the dark. Then, mycelia were washed twice with 150 µl PGM and allowed to recover to room temperature for 1 h. Luminescence was measured with an LB 960 Microplate Luminometer (Berthold Technologies, Germany), which was controlled by a dedicated computer running the MikroWin 2000 software. At the 20-s time point of luminescence reading, 10 mM CaCl2 was applied as a stimulant. At the end of each experiment, the active aequorin was completely discharged by permeabilizing the cells with 20% (v/v) ethanol in the presence of an excess of calcium (3 M CaCl2) to determine the total aequorin luminescence of each culture. The conversion of luminescence (relative light units [RLU]) into [Ca2+] was performed with using Excel 2019 software (Microsoft). Input data were converted using the following empirically derived calibration formula: pCa = 0.332588 (-log k) + 5.5593, where k is luminescence (in RLU) s−1/total luminescence (in RLU).
In vitro phosphorylation analysis
GFP-CchAN300-expressing A. fumigatus were grown in liquid MM for 36 with shaking at 200 rpm. Then, the mild buffer (10 mM Tris-HCl, pH 7.5, 150 mM NaCl, 0.5 mM EDTA, 0.01% Triton X-100, 1 mM DTT, 1 mM PMSF, and 1:100 protease inhibitor cocktail) was used to extract the total proteins. The GFP-CchA were enriched with a GFP-Trap kit (ChromoTek). After enrichment, samples were separated on 10% SDS-PAGE. The gel bands corresponding to the targeted protein GFP-CchA were excised from the gel. The gel pieces were destained 3 times with 125 mM NH4HCO3/50% acetonitrile. Then, proteins were reduced with 10 mM DTT/125 mM NH4HCO3 at 56°C for 30 min and alkylated with 55 mM iodoacetamide/125 mM NH4HCO3 for 30 min in the dark. After washing twice with 50% acetonitrile, the gel pieces were dried in a vacuum centrifuge. Then, 200 ng trypsin in 25 mM NH4HCO3 was added, and the digestion was maintained at 37°C overnight. After digestion, 0.5% formic acid/50% acetonitrile was added to extract peptides from the gel pieces. The extraction procedure was repeated, and the three extracts were combined and dried by vacuum centrifugation. The peptide samples were dissolved in 2% acetonitrile/0.1% formic acid and analyzed using a TripleTOF 5600+ mass spectrometer coupled with the Eksigent nanoLC System (SCIEX, USA). Peptide was loaded onto a C18 trap column (5 μm, 100 μm × 20 mm) and eluted at 300 nl min−1 onto a C18 analytical column (3 μm, 75 µm × 150 mm) over a 60-min gradient. The two mobile phases were buffer A (2% acetonitrile/0.1% formic acid/98% H2O) and buffer B (98% acetonitrile/0.1% formic acid/2% H2O). For information dependent acquisition (IDA), survey scans were acquired in 250 ms, and 40 product ion scans were collected in 50 ms/per scan. MS1 spectra were collected in the range 350–1500 m/z, and MS2 spectra were collected in the range of 100–1500 m/z. Precursor ions were excluded from reselection for 15 s. Phosphorylation site identification was achieved by searching the obtained MS spectra files using Mascot Daemon software.
Proteomics
The Proteomics experiment was performed at Hangzhou PTM Biolabs Co., Ltd. as a commercial service. In brief, lysis buffer (8 M urea, 1% Triton-100, 10 mM dithiothreitol, and 1% Protease Inhibitor Cocktail (Calbiochem)) was used to extract the total protein. Proteins were digested with trypsin (Promega) to be peptides and then processed according to the manufacturer’s protocol for TMT kit. The labeled tryptic peptides were fractionated by HPLC using a Thermo Betasil C18 column (5 μM particles, 10 mm diameter, 250 mm length). After fractioning, the tryptic peptides were analyzed with LC-MS/MS system. The MS/MS data processing was performed using Maxquant search engine (v.1.5.2.8). Tandem mass spectra were searched against Aspergillus UniProt database concatenated with reverse decoy database. Proteins with change ratios significantly different from general protein variation (ΔcnaA/wild-type > 1.50 or < 0.67) were analyzed for GO terms and KEGG pathway analysis using the UniProt-GOA database (http://www.ebi.ac.uk/GOA/) and the KEGG database, respectively.
Virulence assay
For mouse infection model challenged with A. fumigatus conidia, ICR mice (6– 8 weeks old, male, 25 ± 5 g) were purchased from Beijing Vital River Laboratory Animal Technology Co., Ltd. The mice were randomized into four groups (n = 10 each). Mice were immunosuppressed on day −3 and −1 with cyclophosphamide (150 mg kg−1) and on day −1 with hydrocortisone acetate (40 mg kg−1). On day 0, mice were anesthetized with 1% pentobarbital sodium (10 ml kg−1) and infected intratracheally with a 50-μl slurry containing 5 × 106 conidia or 50 μl of PBS as the control. Cyclophosphamide (150 mg kg−1) was injected every 3 days after infection to maintain immunosuppression. Survival was monitored two weeks after inoculation. Lung tissue was processed for histology, Grocott’s methenamine silver stain and periodic acid-Schiff (PAS) staining were performed. For mouse infection model challenged with A. fumigatus hyphae, the mycelia of each strain were homogenized into hyphae fragments using tissue grinding and filtered with lens-cleaning paper in sterile normal saline. The optical density at 600 nm of the 10-fold dilutions of each group were finally adjusted to 0.5. The ICR mice described as above (4 groups, n = 10 each) were immunosuppressed on day −3 and −1 with cyclophosphamide (150 mg kg−1). On day 0, mice were anesthetized with pentobarbital sodium and then infected intratracheally with a 50-µl hyphal suspension. Cyclophosphamide (150 mg kg−1) was injected every 3 days to maintain immunosuppression. Survival was monitored 10 days after inoculation. To directly monitor the infection status in the hyphal infection model, luciferase-expressing A. fumigatus strains were used to infect mice, and live mouse bioluminescence imaging was performed. The mice (4 groups, n = 5 each) were immunosuppressed and infected as in the above description. The infected mice were introduced intraperitoneally with 100 µl D-luciferin in PBS (33.3 mg ml−1) 10 min before bioluminescence imaging. Mice were anesthetized using 2.5% isoflurane with a constant flow and then placed in an IVIS Lumina XR system chamber. Scanning was performed on day 4. The region was cropped in the chest area from each animal, and the total bioluminescence signal intensity of the lung region was extracted and analyzed using Living Image 4.2 (Caliper Life Sciences).
Ethics statement
Animal infection experiments were performed following the Guide for the Care and Use of Laboratory Animals of the U.S. National Institutes of Health. The animal experimental protocol was approved by the Animal Care and Use Committee of Nanjing Normal University, China (permit no. LACUC-20,200,703) according to the governmental guidelines for animal care.
Statistics
Data were given as means ± SD. The SD was from at least three biological replicates. Statistical significance was estimated with Graphpad Prism 7 using Student’s t-test or log-rank test. P-values less than 0.05 were considered statistically significant.
Discussion
The critical role of calcineurin in calcium dysregulation-related human diseases has been recognized for decades [Citation51]. In fungi, calcineurin has been considered an attractive antifungal target because it is essential for stress survival, development, drug resistance, and virulence [Citation34]. Previous studies have reported that calcineurin protects Candida albicans from toxicity caused by the endogenous levels of calcium in serum [Citation52], which is consistent with our results showing that exogenous calcium is toxic when fungal cells lack functional calcineurin in A. fumigatus (). These findings suggest that the role of calcineurin in calcium stress survival mechanism is conserved in Candida and Aspergillus species. However, the underlying mechanism by which calcineurin orchestrates intracellular calcium dynamics remains elusive. Here, through the successful development of an intracellular calcium dynamics monitoring method, we demonstrated that calcineurin dysfunction disturbed calcium homeostasis in the cytosol, vacuole, and mitochondria, leading to a dramatic induction of autophagy and organelle fragmentation and ultimately resulting in significant cell growth inhibition and cell death in the presence of calcium stimuli.
Typically, the resting cytosolic Ca2+ concentration in A. fumigatus ranges from 0.05 to 0.1 μM. In the ΔcnaA mutant, the cytosolic resting Ca2+ level increased by two-fold compared to that of the wild-type prior to calcium stimuli, which is in line with our previous reports on A. nidulans. Moreover, when cells were challenged with 10 mM Ca2+, the ΔcnaA mutant displayed a 33% increase in [Ca2+]c amplitude compared to that of the wild-type. These data support the evolutionarily conserved role of calcineurin in maintaining cytosolic calcium homeostasis across fungal species. The sustained increased cytosolic calcium concentration led us to question whether calcium homeostasis in the intracellular calcium stores such as mitochondria and vacuole would be affected by a lack of calcineurin. We established a novel vacuolar Ca2+ detection approach by fusing the vacuole-targeted protein carboxypeptidase Y (CpyA) to the N-terminus of aequorin, and we found that in the presence of extracellular calcium stimuli, the basal resting level of [Ca2+]vac and the transient [Ca2+]vac in the ΔcnaA mutant were significantly increased, whereas the calcium signature in mitochondria was not significantly affected, implying that vacuoles may be the major site for the detoxification of excess cytosolic calcium in A. fumigatus. Collectively, these data demonstrated that calcineurin not only regulates the resting cytosolic calcium but also affects the vacuolar calcium transient response. Importantly, the increased cytosolic resting [Ca2+]c level and the [Ca2+]vac amplitude seen in the ΔcnaA mutant can be suppressed by deleting the calcium channel CchA, suggesting that calcineurin negatively regulates CchA when cells are exposed to calcium. Accordingly, the feedback relationship between calcineurin and the calcium channel CchA has been described in yeast and A. nidulans [Citation44,Citation53]. In yeast, it has been proposed that Cch1 is dephosphorylated by calcineurin in vitro [Citation54], leading to the inhibition of calcium channel activity, which is in accord with our phosphoproteomic analysis showing that Serine 55 in CchA could be directly dephosphorylated by calcineurin in A. fumigatus (Fig. S8).
In addition to the disordered intracellular calcium homeostasis, the ΔcnaA mutant exhibited the global fragmentation of organelles including the vacuole, the nucleus, ER, and mitochondria, and aggressive autophagy when grown in the presence of 10 mM calcium. Under calcium-treated condition, the growth cessation and cell death of the ΔcnaA mutant start to occur at 60 h and this phenomenon was exacerbated with prolonged incubation time, indicating that the relatively low concentration of calcium is toxic to calcineurin-deficient mutant A. fumigatus. It has been well established that the vacuole plays a key role in detoxifying the excess calcium when cells are exposed to external calcium. Thus, we hypothesized that the cytoplasmic calcium excess combined with deficient vacuolar detoxification capacity would give rise to the calcium-toxicity related phenotypes observed in the ΔcnaA mutant. This hypothesis was supported by the fact that alleviating cytosolic calcium overload by the deletion of cchA or increasing vacuolar calcium storage capacity by overexpressing of the P-type Ca2+-ATPase PmcA can significantly suppress the defective phenotypes of the ΔcnaA mutant. These results highlighted the important roles of calcineurin in mediating calcium homeostasis for fungal survival and the detoxification functions of the vacuoles. Of note, blocking CchA or the overexpression of PmcA is unable to completely suppress the vacuolar fragmentation, aggressive autophagy, and growth cessation in the ΔcnaA mutant in the presence of calcium stimuli, implying that there are other calcium pumps or transporters may also be co-operated with them and involved in the regulation of calcium homeostasis. Probably, examples include the mitochondrial Ca2+ uniporter McuA, the Golgi-localized P-type Ca2+/Mn2+-ATPase PmrA, and the ER-localized P-type ATPase SpfA [Citation47,Citation55,Citation56], all of which have been demonstrated to be required for mediating calcium homeostasis in response to environmental stresses. In addition, although our results suggested that autophagy triggered by the dysregulation of intracellular calcium homeostasis in the ΔcnaA mutant correlates with cell death because blocking autophagy by atg2 deletion could slow the cell death progress, we cannot eliminate the possibility that other “toxic factors” affect the viability of A. fumigatus calcineurin mutants in the presence of calcium. Proteomic analysis revealed that the abundance of various peptidases, nucleases, metacaspases CasA and CasB, and autophagy-related proteins were increased in the ΔcnaA mutant compared to the wild-type strain in calcium-treated conditions for 60 h, implying that apoptosis/apoptotic-like and/or autophagic cell death may occur. Taken together, these results suggested that the disrupted morphology of organelles and increased expression of cell death-related enzymes contribute to calcium toxicity in A. fumigatus calcineurin mutants.
Although cyclosporine A and FK506 (tacrolimus), calcineurin inhibitors, have been shown to possess in vitro antifungal activity against fungal pathogens including A. fumigatus, C. albicans, and C. neoformans, the clinical use of calcineurin inhibitors for antifungal therapy remains limited due to their immunosuppressive side effects [Citation19,Citation37,Citation57]. Because calcineurin inhibitors exhibit synergistic fungicidal activity with antifungal drugs, research on analogues of calcineurin inhibitors with antifungal activity but low immunosuppressive properties may be a promising direction. Encouragingly, APX879, the FK506 analogue, has been shown to exhibit reduced immunosuppressive activity while maintaining limited antifungal activity against pathogens in a murine infection model [Citation58]. Our findings showing that exogenous calcium is able to significantly inhibit the growth of calcineurin mutants highlights a possibility that the combination of calcium and nonimmunosuppressive calcineurin inhibitors could enhance therapeutic efficacy against human pathogens.
Author contributions
C.Z., Y.Z. and L.L., conceived the study; C.Z., Y.R., H.G., and L.G. performed the experiments; and C.Z., Y.Z. and L.L. analyzed and interpreted the data and wrote the manuscript with input from all authors.
Supplemental Material
Download Zip (15.5 MB)Acknowledgments
This work was financially supported by National Key Research and Development Program of China (2019YFA0904900), National Natural Science Foundation of China (31861133014 and 31770086), Program for Jiangsu Excellent Scientific and Technological Innovation team (17CXTD00014), Priority Academic Program Development of Jiangsu Higher Education Institutions to L.L. National Natural Science Foundation of China (31900404) and Natural Science Foundation of the Jiangsu Higher Education Institutions of China (19KJB180017) to Y.Z. We thank Gustavo H. Goldman (University of São Paulo) for kindly providing helpful comments on the manuscript.
Disclosure statement
No potential conflict of interest was reported by the authors.
Supplementary Material
Supplemental data for this article can be accessed here.
Additional information
Funding
References
- Islam MS. Calcium signaling: from basic to bedside. Adv Exp Med Biol. 2020;1131:1–6.
- Rosendo-Pineda MJ, Moreno CM, Vaca L. Role of ion channels during cell division. Cell Calcium. 2020;91:102258.
- Carafoli E. Intracellular calcium homeostasis. Annu Rev Biochem. 1987;56(1):395–433.
- Cyert MS, Philpott CC. Regulation of cation balance in Saccharomyces cerevisiae. Genetics. 2013;193:677–713.
- Blatzer M, Latge JP. Metal-homeostasis in the pathobiology of the opportunistic human fungal pathogen Aspergillus fumigatus. Cur Opin Microbiol. 2017;40: 152–159.
- Park HS, Lee SC, Cardenas ME, et al. Calcium-Calmodulin-Calcineurin signaling: a globally conserved virulence cascade in eukaryotic microbial pathogens. Cell Host Microbe. 2019;26(4):453–462.
- Thewes S. Calcineurin-Crz1 signaling in lower eukaryotes. Eukaryot Cell. 2014;13(6):694–705.
- Cunningham KW. Acidic calcium stores of Saccharomyces cerevisiae. Cell Calcium. 2011;50(2):129–1238.
- Miseta A, Fu L, Kellermayer R, et al. The golgi apparatus plays a significant role in the maintenance of Ca2+ Homeostasis in the vps33Δ Vacuolar biogenesis mutant of Saccharomyces cerevisiae. J Biol Chem. 1999a;274(9):5939–5947.
- Miseta A, Kellermayer R, Aiello DP, et al. The vacuolar Ca2+/H+ exchanger Vcx1p/Hum1p tightly controls cytosolic Ca2+ levels in S. cerevisiae. FEBS Lett. 1999b;451(2):132–136.
- Munoz A, Chu M, Marris PI, et al. Specific domains of plant defensins differentially disrupt colony initiation, cell fusion and calcium homeostasis in Neurospora crassa. Mol Microbiol. 2014;92(6):1357–1374.
- Dolgin E. How secret conversations inside cells are transforming biology. Nature. 2019;567(7747):162–164.
- La Rovere RM, Roest G, Bultynck G, et al. Intracellular Ca2+ signaling and Ca2+ microdomains in the control of cell survival, apoptosis and autophagy. Cell Calcium. 2016;60:74–87.
- Dunn T, Gable K, Beeler T. Regulation of cellular Ca2+ by yeast vacuoles. J Cell Biol. 1994;269:7273–7278.
- Forster C, Kane PM. Cytosolic Ca2+ homeostasis is a constitutive function of the V-ATPase in Saccharomyces cerevisiae. J Biol Chem. 2000;275(49):38245–38253.
- Kmetzsch L, Staats CC, Simon E, et al. The vacuolar Ca2+ exchanger Vcx1 is involved in Calcineurin-Dependent Ca2+ Tolerance and virulence in Cryptococcus neoformans. Eukaryot Cell. 2010a;9(11):1798–1805.
- Chang Y, Schlenstedt G, Flockerzi V, et al. Properties of the intracellular transient receptor potential (TRP) channel in yeast, Yvc1. FEBS Lett. 2010;584(10):2028–2032.
- Denis V, Cyert MS. Internal Ca2+ release in yeast is triggered by hypertonic shock and mediated by a TRP channel homologue. J Cell Biol. 2002;156(1):29–34.
- Hamamoto S, Mori Y, Yabe I, et al. In vitro and in vivo characterization of modulation of the vacuolar cation channel TRPY 1 from Saccharomyces cerevisiae. Febs J. 2018;285(6):1146–1161.
- Lange I, Yamamoto S, Partida-Sanchez S, et al. TRPM2+ functions as a lysosomal Ca2+ -release channel in beta cells. Sci Signal. 2009; (71):2. 10.1126/scisignal.2000278.
- Yu Q, Wang F, Zhao Q, et al. A novel role of the vacuolar calcium channel Yvc1 in stress response, morphogenesis and pathogenicity of candida albicans. Int J Med Microbiol. 2014;304(3–4):339–350.
- Cunningham KW, Fink GR. Calcineurin inhibits VCX1-dependent H+/Ca2+ exchange and induces Ca2+ ATPases in Saccharomyces cerevisiae. Mol Cell Biol. 1996;16(5):2226–2237.
- Dinamarco TM, Freitas FZ, Almeida RS, et al. Functional characterization of an Aspergillus fumigatus calcium transporter (PmcA) that is essential for fungal infection. PloS One. 2012;7(5):e37591.
- Ferreira RT, Silva ARC, Pimentel C, et al. Arsenic stress elicits cytosolic Ca2+ bursts and Crz1 activation in Saccharomyces cerevisiae. Microbiology-Sgm. 2012;158(9):2293–2302.
- Kmetzsch L, Staats CC, Simon E, et al. The vacuolar Ca2+ exchanger Vcx1 is involved in calcineurin-dependent Ca2+ tolerance and virulence in Cryptococcus neoformans. Eukaryot Cell. 2010b;9(11):1798–1805.
- Klionsky DJ. Cell biology – Autophagy as a regulated pathway of cellular degradation. Science. 2000;290(5497):1717–1721.
- Nakatogawa H. Mechanisms governing autophagosome biogenesis. Nat RevMol Cell Biol. 2020;21:439–458.
- Liu R, Li J, Zhang T, et al. Itraconazole suppresses the growth of glioblastoma through induction of autophagy: involvement of abnormal cholesterol trafficking. Autophagy. 2014;10(7):1241–1255.
- Wang Y, Zhang H. Regulation of autophagy by mTOR signaling pathway. Adv Exp Med Biol. 2019;1206:67–83.
- Yi C, Tong JJ, Yu L. Mitochondria: the hub of energy deprivation-induced autophagy. Autophagy. 2018;14:1084–1085.
- Scarlatti F, Granata R, Meijer AJ, et al. Does autophagy have a license to kill mammalian cells?. Cell Death Differ. 2009;16(1):12–20.
- White E, DiPaola RS. The double-edged sword of autophagy modulation in cancer. Clin Cancer Res. 2009;15(17):5308–5316.
- Denning DW, Bromley MJ. Infectious disease. How to bolster the antifungal pipeline. Science. 2015;347(6229):1414–1416.
- Juvvadi PR, Lee SC, Heitman J, et al. Calcineurin in fungal virulence and drug resistance: prospects for harnessing targeted inhibition of calcineurin for an antifungal therapeutic approach. Virulence. 2017;8(2):186–197.
- Steinbach WJ, Cramer RA Jr., Perfect BZ, et al. Calcineurin controls growth, morphology, and pathogenicity in Aspergillus fumigatus. Eukaryot Cell. 2006;5(7):1091–1103.
- Steinbach WJ, Cramer RA Jr., Perfect BZ, et al. Calcineurin inhibition or mutation enhances cell wall inhibitors against Aspergillus fumigatus. Antimicrob Agents Chemother. 2007;51(8):2979–2981.
- Fox DS, Heitman J. Good fungi gone bad: the corruption of calcineurin. Bioessays. 2002;24(10):894–903.
- Park HS, Chow EW, Fu C, et al. Calcineurin targets involved in stress survival and fungal virulence. PLoS Pathog. 2016;12(9):e1005873.
- Juvvadi PR, Fortwendel JR, Rogg LE, et al. Localization and activity of the calcineurin catalytic and regulatory subunit complex at the septum is essential for hyphal elongation and proper septation in aspergillus fumigatus. Mol Microbiol. 2011;82(5):1235–1259.
- Nelson G, Kozlova-Zwinderman O, Collis AJ, et al. Calcium measurement in living filamentous fungi expressing codon-optimized aequorin. Mol Microbiol. 2004;52(5):1437–1550.
- Kikuma T, Ohneda M, Arioka M, et al. Functional analysis of the ATG8 homologue Aoatg8 and role of autophagy in differentiation and germination in Aspergillus oryzae. Eukaryot Cell. 2006;5(8):1328–1336.
- Kotani T, Kirisako H, Koizumi M, et al. The Atg2-Atg18 complex tethers pre-autophagosomal membranes to the endoplasmic reticulum for autophagosome formation. Proc Natl Acad Sci U S A. 2018;115(41):10363–10368.
- Tang Z, Takahashi Y, Wang HG. ATG2 regulation of phagophore expansion at mitochondria-associated ER membranes. Autophagy. 2019;15(12):2165–2166.
- Wang S, Liu X, Qian H, et al. Calcineurin and Calcium channel CchA coordinate the salt stress response by regulating Cytoplasmic Ca2+ Homeostasis in Aspergillus nidulans. Appl Environ Microbiol. 2016;82(11):3420–3430.
- Zhang C, Lu L. Precise and efficient in-frame integration of an exogenous GFP tag in Aspergillus fumigatus by a CRISPR system. Methods Mol Biol. 2017;1625:249–258.
- Greene V, Cao H, Schanne FA, et al. Oxidative stress-induced calcium signalling in Aspergillus nidulans. Cell Signaling. 2002;14(5):437–443.
- Song J, Liu X, Zhai P, et al. A putative mitochondrial calcium uniporter in A. fumigatus contributes to mitochondrial Ca2+ homeostasis and stress responses. Fungal Genet Biol. 2016a;94:15–22.
- Song J, Zhai P, Zhang Y, et al. The aspergillus fumigatus damage resistance protein family coordinately regulates ergosterol biosynthesis and azole susceptibility. mBio. 2016b;7(1):e01919–01915.
- Zhang C, Meng XH, Wei XL, et al. Highly efficient CRISPR mutagenesis by microhomology-mediated end joining in Aspergillus fumigatus. Fungal Genet Biol. 2016a;86:47–57.
- Zhang Y, Zheng Q, Sun C, et al. Palmitoylation of the Cysteine residue in the DHHC Motif of a Palmitoyl Transferase Mediates Ca2+ Homeostasis in Aspergillus. PLoS Genet. 2016b;12(4):e1005977.
- Park YJ, Yoo SA, Kim M, et al. The role of Calcium-Calcineurin-NFAT signaling pathway in health and autoimmune diseases. Front Immunol. 2020;11:195.
- Blankenship JR, Heitman J. Calcineurin is required for Candida albicans to survive calcium stress in serum. Infect Immun. 2005;73(9):5767–5774.
- Ma Y, Sugiura R, Koike A, et al. Transient receptor potential (TRP) and Cch1-Yam8 channels play key roles in the regulation of cytoplasmic Ca2+ in fission yeast. PLoS One. 2011;6(7):e22421.
- Bonilla M, Cunningham KW. Mitogen-activated Protein Kinase Stimulation of Ca2+ Signaling is required for survival of endoplasmic reticulum stress in yeast. Mol Biol Cell. 2003;14(10):4296–4305.
- Pinchai N, Juvvadi PR, Fortwendel JR, et al. The Aspergillus fumigatus P-type Golgi apparatus Ca2+/Mn2+ ATPase PmrA is involved in cation homeostasis and cell wall integrity but is not essential for pathogenesis. Eukaryot Cell. 2010;9(3):472–476.
- Yu Q, Wang H, Xu N, et al. Spf1 strongly influences calcium homeostasis, hyphal development, biofilm formation and virulence in Candida albicans. Microbiology. 2012;158(9):2272–2282.
- Groll AH, De Lucca AJ, Walsh TJ. Emerging targets for the development of novel antifungal therapeutics. Trends Microbiol. 1998;6(3):117–124.
- Juvvadi PR, Fox D 3rd, Bobay BG, et al. Harnessing calcineurin-FK506-FKBP12 crystal structures from invasive fungal pathogens to develop antifungal agents. Nature Commun. 2019;10(1):4275.