ABSTRACT
Neisseria gonorrhoeae (gonococcus) causes the human sexually transmitted disease gonorrhea. Studying gonococcal pathogenesis and developing new vaccines and therapies to combat the increasing prevalence of multi-antibiotic resistant bacteria has made use of many ex vivo models based on human cells and tissues, and in vivo vertebrate models, for example, rodent, pig and human. The focus of the current study was to examine the utility of the invertebrate greater wax moth Galleria mellonella as an in vivo model of gonococcal infection. We observed that a threshold of ~106 – 107 gonococci/larva was required to kill >50% of larvae (P < 0.05), and increased toxicity correlated with reduced health index scores and pronounced histopathological changes such as increases in the total lesion grade, melanized nodules, hemocyte reaction, and multifocal adipose body degeneration. Larval death was independent of the expression of pilus or Opa protein or LOS sialylation within a single gonococcal species studied, but the model could demonstrate relative toxicity of different isolates. N. meningitidis, N. lacatamica and gonococci all killed larvae equally, but were significantly less toxic (P > 0.05) than Pseudomonas aeruginosa. Larvae primed with nontoxic doses of gonococci were more susceptible to subsequent challenge with homologous and heterologous bacteria, and larval survival was significantly reduced (P < 0.05) in infected larvae after depletion of their hemocytes with clodronate-liposomes. The model was used to test the anti-gonococcal properties of antibiotics and novel antimicrobials. Ceftriaxone (P < 0.05) protected larvae from infection with different gonococcal isolates, but not azithromycin or monocaprin or ligand-coated silver nanoclusters (P > 0.05).
Introduction
Neisseria gonorrhoeae (gonococcus) causes the human sexually transmitted disease gonorrhea. Worldwide, there are ~87 million cases of gonococcal infection reported annually by the World Health Organization (WHO) [Citation1], with the majority in the least developed and low-to-middle-income countries. The gonococcus infects the mucosal epithelium of the male urethra, causing urethritis and painful discharge, and the female endo/ectocervix, causing a mucopurulent cervicitis. However, in approximately 10–25% of untreated women, gonococci can ascend into the upper reproductive tract and cause pelvic inflammatory disease, which can result in long-term and/or permanent chronic pelvic pain, fallopian tube damage, endometritis, ectopic pregnancy, and infertility [Citation2]. Furthermore, rare Disseminated Gonococcal Infection (DGI) can be manifested as septicemia, meningitis, arthritis, tenosynovitis, endocarditis and cutaneous infection. Other body sites can be infected, with reports of gonococcal oro- and nasopharyngeal infections, tonsillitis, stomatitis, parotitis, scalp abscess, and mastitis [Citation3]. Gonococcal conjunctivitis is still reported in the least developed and low-to-middle-income countries and adult gonococcal keratoconjunctivitis is also now seen as a serious emerging infection. Furthermore, co-infection with other sexually transmitted disease pathogens, for example, HIV, Treponema pallidum, Trichomonas, and Chlamydia, is common, and gonococci are known to increase the risk of HIV transmission and infection.
There is a substantial literature on N. gonorrhoeae pathogenesis studies using many ex vivo models based on human cells and tissues, mouse models, a pig model, and the experimental human male volunteer urethral gonorrhea model [Citation4–9]. These models have informed considerably our understanding of gonococcal–host interactions, which is central to developing vaccines and new therapies to combat the increasing prevalence of multi-antibiotic resistant gonococci [Citation10]. Testing in vertebrate mouse models is a pre-requisite to human clinical trials, but they are expensive for high-throughput studies and require considerable operator experience. Complementary in vivo models could make use of invertebrates and the utility of the greater wax moth Galleria mellonella (Subfamily Galleriinae, Family Pyralidae, Order Lepidoptera) [Citation11] is the focus of our study. G. mellonella is a pest to honey bee colonies and undergoes complete metamorphosis, moving from egg to larva to pupa to adult [Citation12]. G. mellonella larvae possess several properties that make them useful for studying microbial infection [Citation13]: they are readily available and inexpensive to purchase, do not require special equipment or conditions of storage for short period of times, their size makes injection, homogenization and hemolymph extraction facile, they have a short life cycle making them suitable for large-scale studies, they can survive at between 15°C and 37°C, their genome has been sequenced and their use does not require ethical approval. In addition, their innate immune system shares many similarities with the mammalian innate immune system. Cellular immunity is mediated by hemocytes that function in phagocytosis, nodulation, and encapsulation [Citation14]. Other immunity factors include melanization [Citation15], the prophenolaxidase activating system (Pro-PO-AS) [Citation16], the synthesis of small antimicrobial peptides [Citation17] and lysozyme [Citation18].
There is extensive literature describing the use of G. mellonella to study infection by many bacteria [Citation13], for example Pseudomonas aeruginosa [Citation19], Mycobacterium tuberculosis [Citation20], Burkholderia spp [Citation21], Staphylococcus aureus [Citation22], Listeria monocytogenes [Citation23], Streptococcus pneumoniae [Citation24], Actinobacillus pleuropneumoniae [Citation25], Shigella spp [Citation26], Enterobacter cloacae [Citation27], Bacillus thuringiensis [Citation28], and fungi, such as Candida [Citation29] and parasites, such as Leishmania and Trypanosoma spp [Citation30]. In addition, G. mellonella is being used as a model for testing new antibacterial, antifungal, and anti-parasite strategies and novel drugs and antibiotics [Citation31]. In the current study, we describe the development of a G. mellonella larval model of N. gonorrhoeae infection, which to our knowledge has not been previously reported for this bacterium.
Materials and methods
Bacteria and growth media
Information on the Neisseria gonorrhoeae strains and other bacteria used in this study is provided in . Neisseria spp. and P. aeruginosa were grown on supplemented GC agar plates [Citation32] or in supplemented GC broth incubated at 37°C in an atmosphere containing 5% (v/v) CO2. Lactobacillus spp. were cultured on Tryptic Soy Agar (Oxoid) at 37°C with 5% (v/v) CO2.
Table 1. Neisseria gonorrhoeae strains and other bacteria used in this study
Killed gonococci were prepared by heating at 56°C for 60 min in a water bath. Sialylation of gonococcal lipooligosaccharide (LOS) glycans was done by growing bacteria on Cytidine-5′-monophospho-N-acetylneuraminic acid (CMP-NANA, Merck) supplemented plates. Briefly, 2 mg of CMP-NANA powder was dissolved in 1 mL of ultra-high quality (UHQ) water and the solution spread onto the surface of 20 mL of supplemented GC agar medium to give a final concentration of 50 µg/mL. Gonococci were grown directly onto CMP-NANA plates for use. Vancomycin, Colistin sulfate, Nystatin, and Trimethoprim (VCNT, Oxoid) supplemented GC agar plates were used to selectively isolate gonococci from infection experiments with mixed bacteria and to recover viable gonococci from the hemolymph extraction. Powdered VCNT was dissolved aseptically with 2 mL of sterile UHQ and mixed with 500 mL of GC agar medium to give final concentrations per 20 ml agar plate of V 0.06 mg, C 0.15 mg, N 250 IU and T 0.1 mg.
Preparation of N. gonorrhoeae lipooligosaccharide (LOS)-replete outer membranes (OM)
OM of N. gonorrhoeae strain P9-17 were prepared by lithium acetate extraction as described previously [Citation33]. Protein concentration was determined using a Bicinchoninic acid (BCA) assay, following the manufacturer’s instructions (Thermo Fischer).
Preparation of live microbial inocula for infection of G. mellonella
N. gonorrhoeae, P. aeruginosa, and Lactobacillus spp. were cultured from frozen stocks onto appropriate agar media for ≤16 h at 37°C with 5% (v/v) CO2. On the next day, single colonies were selected from the overnight culture and spread onto fresh agar plates, which were incubated at 37°C with 5% (v/v) CO2 for 6 h. Then, the culture from the agar plates was suspended in supplemented GC broth. Absorbance (Optical Density, OD) of the bacterial suspensions was measured by spectrophotometer at λ595 nm (iMark microplate reader, BIO-RAD). When the required optical density was reached for the microbial inocula, 10 µL of each different dose were pipetted onto a sterile Petri dish 11 times to generate 11 discrete bubbles. In addition, 10 µL of titrated microbial inocula were pipetted onto fresh agar plates in triplicate and grown overnight to determine concentrations of inocula (colony forming units (CFU)/mL).
Injection of G. mellonella larvae
Last larval stage Galleria larvae (Wazp-Brand, Monkfield Nutrition, UK) was collected from a local pet shop immediately following delivery to assure fresh insects. Larva were examined visually and sorted by size and each larva was weighed and larva of 0.25–0.35 g were used for the model. Larvae (n = 10) were placed into individual sterile Petri dishes and each test or control group had at least three sets of Petri dishes with larvae (n = 30). Larvae were handled gently and with care and maintained at 37°C with a 5% (v/v) CO2 atmosphere for the duration of the experiments.
Initial experiments were done to determine which medium was nontoxic toward Galleria mellonella larvae and maintained bacterial viability. Larvae were injected (10 µL/larva) with phosphate-buffered saline (PBS), pH 7.4, PBS containing the GC supplements [Citation32], PBS with 10 mM of ferric citrate, PBS with 10 mM of ferric nitrate, or with supplemented GC broth alone.
Groups of 10 individual larvae were injected in the last left pro-leg with 10 µL of various doses of live bacteria, dead bacteria, or OM using U-100 insulin syringes (BB Micro-Fine). A single syringe was used for the 10 caterpillars within the same group. Control groups for each experiment included 1) untreated larvae; 2) trauma – larvae were pricked once in the last left pro-leg with a sterile syringe; 3) sterile supplemented GC broth; and 4) PBS. In all experiments in which gonococci and other bacteria were tested, a positive control was included of larvae that were injected with 10 µL of supplemented GC broth containing ~150 CFU/larva of P. aeruginosa PAO1. This control group always resulted in all larvae dying within the first 16 hours of the experiment.
At the end of each experiment, both dead and surviving G. mellonella, were placed into plastic bags and kept at −20°C overnight to sedate and kill them humanely. On the following day, the plastic bag containing dead larvae was autoclaved at 121°C for 15 min, 2.68 kg/cm2 and discarded.
G. mellonella Health Index Scoring System (HISS)
A G. mellonella Health Index Scoring System (HISS) based on Loh et al. [Citation34] was used to observe and record larval health changes (). The virulence of strain P9-17 was measured at 0 h, 16 h, 24 h, 40 h, and 48 h. The development of melanization was observed, and larvae were recorded as dead when they stopped responding to stimuli, for example touching of larvae with disinfected tweezers or movement in response to tapping of the Petri dish.
Table 2. Galleria mellonella Health Index Scoring System (HISS). A healthy, uninfected waxworm typically scores between 9 and 10, and an infected, dead wax worm typically scores 0. The health index scores from a very early stage of post-infection correlate well with the infectious dose, with higher inocula generally resulting in lower health indices. Based on Loh et al. [Citation34]
Co-infection of G. mellonella with Lactobacillus spp. and N. gonorrhoeae
The method was adapted from Santos et al. [Citation35] and Scalfaro et al. [Citation36]. Lactobacillus brevis, crispatus, and gasseri were cultured as described above for live microbial inocula. Initially, G. mellonella sensitivity assay was done by injecting different dilutions (OD 0.1 (~105), 0.6 (~106), 0.8 (~107) and 1.0 (~108) CFU/larva) of all three Lactobacillus species into larvae and monitoring their survival for 48 h.
After assessing sensitivity, L. gasseri and N. gonorrhoeae P9-17 were cultured as described above and 10 larvae per group were each injected with 10 µL of supplemented GC broth containing either 0.1 (~105 CFU/larva), 0.6 (~106 CFU/larva) or 0.8 OD (~107 CFU/larva) of L. gasseri bacterial suspension or 0.8 OD (~107 CFU/larva) of P9-17. After 16 h, groups of L. gasseri-primed larvae were injected with 10 µL supplemented GC broth of 0.8 OD of P9-17 bacterial suspension. Larvae were monitored for 48 h and dead larvae were counted at 24 and 48 h post-infection with P9-17.
Effect of priming G. mellonella with N. gonorrhoeae
The method was adapted from Bergin et al. [Citation37] and Taszlow et al. [Citation38]. N. gonorrhoeae P9-17 was cultured on supplemented GC agar and in broth as described above for live microbial inocula. Fifteen larvae were infected with 10 µL of 0.1 OD (~0.7 × 105 CFU/larva), which is not lethal to G. mellonella. After 16 h, a high dose of 10 µL of 0.8 OD (107 CFU/larva), medium dose of 10 µL of 0.4 OD (106 CFU/larva) and low dose of 10 µL of 0.1 OD (105 CFU/larva) was used to infect larvae. We also tested the impact of priming the larvae with 0.1 OD of P9-17 followed by infection with 0.8 OD of live L. gasseri and 0.8 OD of heat-inactivated L. gasseri and P9-17. Survival of larvae was assessed for 48 h post-re-infection with bacteria.
Recovery of viable N. gonorrhoeae from G. mellonella
Initially, we trialed several methods to quantify gonococcal numbers within infected larvae, including hemolymph recovery using pestle and mortar, flow cytometry with P9-17 expressing Green Fluorescent Protein, and a LIVE/DEAD BacLight Bacterial Viability Kit, but none worked as reliably (data not shown) as the recovery of gonococci from the hemolymph of larvae infected with gonococci using VCNT selective agar plates. Briefly, larvae were placed at −20°C for 2 to 4 min and then transferred to a Class II biosafety cabinet. A sterile scalpel blade was used to cut 2–3 mm from the larval end and hemolymph was squeezed into 1.5 mL Eppendorf tubes. Extracted hemolymph was diluted serially in sterile supplemented GC broth and 10 µL aliquots spread onto VCNT supplemented GC agar plates in triplicate and incubated overnight at 37°C with 5% (v/v) CO2. CFU were counted and recorded after 16–48 h of incubation. Hemolymph extractions were done at 16 h, 24 h, 40 h, and 48 h post-injection of bacteria into G. mellonella.
Chemical depletion of G. mellonella hemocytes
Dichloromethylene-bisphosphonate (clodronate) is a chemical used to deplete macrophages [Citation39] and was used in our study to deplete hemocytes from G. mellonella. A suspension of artificially prepared lipid vesicles encapsulating clodronate was used for the experiments and adapted from the method of Kwon and Smith, who used clodronate-filled liposomes to deplete hemocytes in Anopheles gambiae mosquitos [Citation40]. Liposomes were from Liposoma research (https://clodronateliposomes.com), and two types of liposomes were used: control liposomes (Lip) in PBS and liposomes containing 5 mg/mL clodronate (CDLip). Groups of 10 G. mellonella larvae were injected in the last left pro-leg with Lip (10 µL) or CDLip (10 µL) at neat and at 1/5, 1/10 and 1/20 dilutions. After 16 h, the larvae were injected with various doses of N. gonorrhoeae P9-17 (OD 0.1, or 0.4 or 0.8) and larval survival was monitored over a 48 h period. Neither Lip nor CDLip killed gonococci when tested against the organism alone.
Histopathology
Live larvae were fixed at 2, 16, and 24 h to examine cellular immune responses to gonococcal infection. Larvae infected with N. gonorrhoeae P9-17 and control, uninfected larvae were fixed by injecting 100 µL of 10% (v/v) of neutral buffered formalin (CellPath) into the last right pro-leg of the caterpillar and immersing three larvae per time point in a labeled Cellstor Pot filled with 60 mL of 10% (v/v) of neutral buffered formalin (CellPath). Injection and fixation were repeated three times to generate larvae for histopathology from three independent experiments.
Larvae were sectioned to produce longitudinal or transverse sections, placed into cassettes and then processed overnight using a Sakura VIP 6 Vacuum Infiltration Processor. Tissues were embedded into molten paraffin wax using Sakura TEC 5 Tissue Embedding Console. The blocks were cut using a Leica RM2235 microtome at 3 microns and sections placed onto regular glass slides. Once the sections were dry, they were placed into the Sakura DRS 2000 with Dryer where they were stained using a standard Hematoxylin & Eosin (H&E) protocol with xylene and alcohol washes. Following staining, the slides were cover-slipped using a xylene-based mountant and left to dry for 12 h and then examined using light microscopy.
Longitudinal sections were generated by bisecting the larva and embedding both halves in paraffin with the cut surface toward the microtome blade, yielding two sections of each larva for examination. For transverse-sectioned larvae, sections were generated as follows: the larva was first bisected transversely into equal halves and both the cranial and caudal halves were sectioned transversely into three equal segments. These were then embedded in paraffin to produce blocks with two cranial cut surfaces, two mid-body cut surfaces, and two caudal cut surfaces oriented toward the microtome blade, yielding a total of six transverse sections of each larva for histopathological examination. Quality of fixation in all slides was excellent and section quality ranged from fair to excellent. A histological grading scheme for common lesions was used to score larvae ().
Table 3. Histopathology grading scheme for common lesions in Galleria mellonella.
Utility of G. mellonella for testing antimicrobial agents against N. gonorrhoeae
The method was adapted from Ignasiak and Maxwell [Citation41]. Standard microbial inocula (~8 × 107 CFU/larva, that is, 10 µL of 0.8 OD) of N. gonorrhoeae P9-17 and isolates selected from the CDC/FDA panel were prepared as described above. The G. mellonella infection model was used to assess the ability of antibiotics used to treat gonorrhea, and novel antimicrobials, to prevent larval death over time. Ceftriaxone (sodium salt, Merck), was dissolved in PBS and then diluted for testing (10 µL/larva of 0.008 µg/mL to 1000 µg/mL). Azithromycin (Merck) was dissolved in dimethyl sulfoxide and then diluted with PBS for testing (10 µL/larva of 256 µg/mL to 1.024 mg/mL). 5-Mercapto-2-nitrobenzoic Acid-Coated Silver Nanoclusters [Citation42] were diluted in PBS for testing (10 µL/larva of 0.467–58.44 µM). 1-Decanoyl-rac-glycerol (monocaprin, Merck) [Citation43] was dissolved in 100% (v/v) ethanol and then diluted with PBS for testing (10 µL/larva of 0.1–50 mM).
The toxicity of antibiotics and antimicrobials toward G. mellonella was assessed by injecting larvae with 10 µL of different doses of the compounds and monitoring survival for 48 h. To assess their activity against gonococci in vivo, G. mellonella larvae (n = 10 per sample dose group) were injected with 10 µL of gonococcal standard dose (~8 × 107 CFU/larva) and 10 µL of different doses of antimicrobial compound. Larval survival was measured at 16, 24, 40, and 48 h post-injection.
Minimum Inhibitory Concentration (MIC) values of ceftriazone and azithromycin for N. gonorrhoeae P9-17
The broth microdilution method (based on the Clinical and Laboratory Standards Institute (CLSI) guidelines with some modifications as used by Foerster et al. [Citation44]) was used to determine MIC values. Briefly, fresh 6 h cultures of P9-17 were grown as described above and 190 μL of a suspension of 5 × 105 CFU/mL in supplemented GC broth was added to individual wells of a sterile 96 well microtiter plate. Next, 10 μL aliquots of the doses of antibiotic in 2-fold dilutions were added to triplicate wells. Controls were bacteria with no antibiotic and medium alone. Inocula were counted on supplemented GC agar plates as described above. The plates were incubated for 24 h at 37°C and optical density was read at λ595 nm (iMark microplate reader, BIO-RAD). The MIC value was defined as the minimum dose (μg/mL) that showed >90% reduction in optical density compared to the untreated control. Data are from triplicate-independent experiments with each antibiotic.
Data analysis and reproducibility
Details of all technical and biological repeats for all of the experiments are reported in the Figure Legends. Data were analyzed using GraphPad Prism with unpaired t-Test to compare between groups, with P values <0.05 considered significant.
Results
Effect of different growth media on G. mellonella survival
Initially, we determined the optimal medium to use in our model that was not toxic to the larvae whilst maintaining gonococcal viability. Untreated larvae, traumatized larvae, and larvae injected with supplemented GC broth or PBS with and without the supplements all showed <10% mortality over a 72 h period (Figure S1). By contrast, larvae injected with PBS containing ferric nitrate or ferric citrate showed significant average mortality of 30–40% over 72 h, compared with untreated controls (P < 0.01). Although there was no significant difference between using supplemented GC broth or PBS for maximal larval survival (P > 0.05), we chose the former for all subsequent infection experiments since this medium is preferred for gonococcal growth and viability.
Infection of G. mellonella with N. gonorrhoeae strain P9-17
In our first experiments, we injected G. mellonella larvae with various doses of N. gonorrhoeae P9-17 (Pil+ Opab+) and examined larval survival over 48 h ()). We observed a dose-dependent relationship with larval survival, with increasing doses of bacteria reducing survival. A suspension of optical density (OD) of 0.6 (~9 × 106 CFU/larva) caused >50% death by 30 h and higher doses of OD 0.8 (~8 × 107 CFU/larva) and 1.0 (~6 × 108 CFU/larva) were the most lethal, causing >80% death by 48 h, compared to larvae injected with supplemented GC broth alone (P < 0.001). The mortality observed with the OD 0.8–1.0 doses was significantly greater than the mortality observed with all doses ≤ OD 0.6 (P < 0.05). For all subsequent experiments, we chose a standard inoculum of OD 0.8 (~8 × 107 CFU/larva) as this gave consistent and reproducible killing of >50% of larvae by 18 h.
Figure 1. Infection of Galleria mellonella with Neisseria gonorrhoeae strain P9-17. A) The effect of varying inoculum dose of N. gonorrhoeae on survival of G. mellonella. Healthy larva (n = 10 per group) were infected with differing inocula of gonococci, incubated at 37°C and examined for survival by response to touch over a period of 48 h. The symbols represent mean survival from a minimum of 8 independent experiments and the error bars represent the standard deviation of the mean. Unpaired t test was done at each time point to compare survival in infected groups with control group of larvae injected with GC broth. * denotes statistically significant difference for all data points with P < 0.001. B) Health Index Scores for G. mellonella injected with varying doses of N. gonorrhoeae P9-17. Healthy larva (n = 10 per group) were infected with differing inocula of gonococci, incubated at 37°C and examined with the Health Index Scoring System (HISS) over a period of 48 h. The symbols represent the mean health index score of 3 independent experiments and the error bars represent the standard deviation of the mean. Unpaired t test was done to compare at each time point between gonococcal-infected HISS data and control larvae injected with GC broth alone. * denotes statistically significant difference for all data points with P < 0.05. C) Recovery of N. gonorrhoeae from G. mellonella hemolymph. At least 30 larvae were infected with 10 µl of 8 × 107 CFU N. gonorrhoeae P9-17. Survival of N. gonorrhoeae within live and dead G. mellonella was determined by cutting the end of the larva, extracting hemolymph and plating it on to the VCNT supplemented GC agar plates at different time points (16, 24, 40, and 48 h). Data are pooled from four independent experiments with 1 larva per time-point per experiment. Symbols represent mean survival of larvae and the error bars show standard deviation of the mean. Unpaired t test was done to compare between live and dead CFU from larvae at each time point
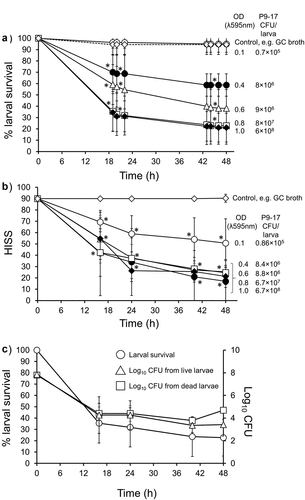
During these initial infection experiments, we also used a Health Index Scoring System (HISS) to score the health of the larvae. HISS enabled measurement of some of the more subtle differences that were observed during infection (). Generally, HISS scores declined over time with infection and were dose-dependent (). Compared to larvae injected with control supplemented GC broth, larvae infected with the lowest dose of OD 0.1 (~8.6 × 105 CFU/larva) showed a significant gradual decline in score to ~55 HISS points by 48 h (P < 0.05), whereas the highest doses tested of OD 0.8 − 1.0 (~6.7 × 107 to 6.7 × 108 bacteria/larva) showed significantly reduced HISS scores of ~20 by 48 h () (P < 0.05).
We also examined the levels of P9-17 within G. mellonella over time by sampling larval hemolymph onto selective agar medium plates. Larvae were infected with OD 0.8 (~8 × 107 bacteria/larva) and hemolymph was sampled from both live and recently dead larvae at intervals over 48 h. There was a rapid decrease in larval survival from 100% to 30% by 48 h. We also observed that bacterial numbers gradually fell from mean values of ~108 at 0 h to ~102 CFU/larva in live larvae and to ~104 in dead larvae by 48 h (). There were no significant differences between the numbers of bacteria recovered from live and dead larvae at each time point (P > 0.05).
Detailed histopathology examination of G. mellonella infected with N. gonorrhoeae strain P9-17
Larvae were infected with OD 0.8 (~8 × 107 CFU/larva) of N. gonorrhoeae P9-17 and processed at 2, 16, and 24 h post-infection for H&E staining and histopathology examination. Uninfected larvae were processed at the same time points. Representative H&E stained images are shown in . The grading scores for numbers of melanized nodules, adipose body necrosis grade, hemocyte reaction grade and total grade score for individual larva examined are shown in Table S1. Compared to the uninfected control larvae, infection resulted in significant increases in the total lesion grade (P < 0.01), in melanized nodules (P < 0.01) and hemocyte reaction (P < 0.01) at each time point, but there was no significant difference in adipose body necrosis (P > 0.05) ()). Representative images of increased melanization over time in whole larvae infected with P9-17 are shown in .
Figure 2. Histopathology examination of Galleria mellonella infected with Neisseria gonorrhoeae. Larvae were infected with Neisseria gonorrhoeae P9-17 (OD 0.8, ~8 × 107 CFU/larva) and histopathology examined at 2, 16 and 24 h after infection on fixed larval sections following H&E staining. Uninfected larvae were processed at the same time points. The images are representative of several larvae as described in Table S1. A) 2 h post-injection control, larva 1. Normal adipose body and no hemocyte reaction. Hemocytes form a monolayer in the subcuticular space and individual hemocytes are scattered within the adipose body. AB = adipose body, Cu = cuticle, arrows = hemocytes. B) 2 h gonococcal infected, larva 4. Locally extensive necrosis of the adipose body with scattered melanin is present between the crop and tracheal apparatus. AB = adipose body, Ne = necrosis, TA = tracheal apparatus, Cr = crop, arrows = melanin. C) 2 h gonococcal infected, larva 4. There are large numbers of hemocytes forming a densely cellular aggregate adjacent to the adipose body. Additional hemocytes are filling space between folds of the adipose body. AB = adipose body, H = hemocytes. D) 16 h post-injection control, larva 3. Normal adipose body (AB), silk gland (SG), and intestinal tract (IT). E) 16 h gonococcal infected, larva 3. Aggregates of hemocytes, occasionally with melanin, are present multi-focally throughout the proleg. AB = adipose body, Cu = cuticle, SM = skeletal muscle, H = hemocytes, MN = melanized nodule, TA = tracheal apparatus. F) 24 h post-injection control, larva 3. There is proteinaceous fluid in the coelom adjacent to the crop and intestinal tract. AB = adipose body, Cr = crop, IT = intestinal tract, PF = proteinaceous fluid. G) 24 h gonococcal infected, larva 1. Multiple melanized nodules and clusters of hemocytes within the coelom adjacent to the rectum. MN = melanized nodules, AB = adipose body, R = rectum, arrows = hemocyte clusters. H) Presence of N. gonorrhoeae P9-17 within G. mellonella tissue (magnification is x100)
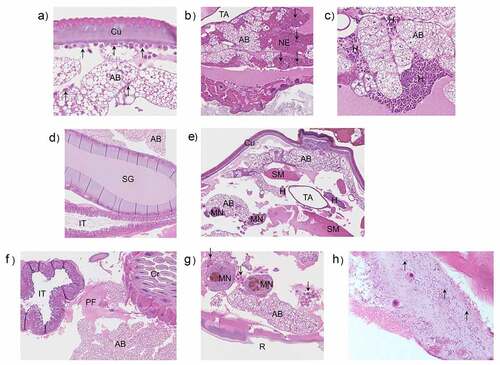
Figure 3. A) Use of a common lesion grading scheme for Galleria mellonella infected with N. gonorrhoeae strain P9-17. Groups of larvae infected with strain P9-17 (OD 0.8, ~8 × 107 CFU/larva) were assessed over 24 h for melanization, adipose body necrosis and hemocyte reaction grades to provide a total score in lesion grading. The symbol represents the mean and error bars the standard deviation of the mean. Unpaired t test was done to compare the scores between infected and uninfected larvae. Ns, not significant (p > 0.05) and * denotes statistical significance, with P < 0.01. B) Representative images of larvae infected with gonococci showing increasing levels of melanization with time.
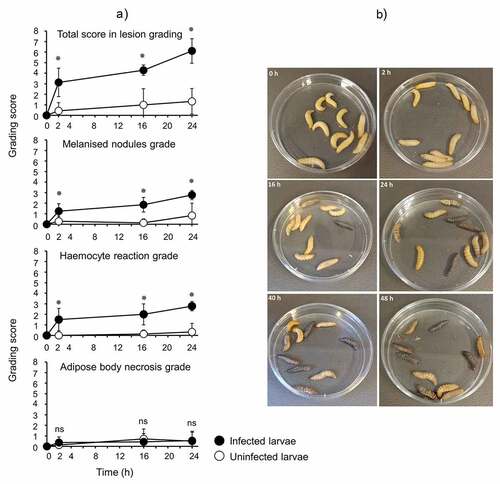
Infection of G. mellonella with phenotypic variants of Neisseria gonorrhoeae strain P9-17 and other gonococcal isolates
The pilus and Opa protein are cardinal molecules mediating gonococcal interactions with host cells [Citation45]. We injected larvae with OD 0.8 (~8 × 107 CFU) of different pilus and Opa protein-expressing phenotypic variants and found that they significantly reduced larval survival to between 10% and 40% by 48 h (P < 0.01), compared with 100% survival of control larvae injected with supplemented GC broth. However, there were no significant differences (P > 0.1) between the phenotypic variants in causing larval death ()). Sialylation of gonococcal LOS glycans is a virulence mechanism used by gonococci to evade the human immune system [Citation46]. We examined if sialylation of gonococcal LOS increased the virulence of bacteria in G. mellonella. Larvae were infected with OD 0.1, 0.6 and 1.0 of untreated and CMP-NANA grown P9-17 and survival monitored over 48 h. As expected, survival was increasingly reduced with doses of OD 0.6 and 1.0 bacteria, compared with control larvae injected with supplemented GC broth alone (P < 0.05). However, there was no statistically significant difference (P > 0.05) in the survival curves of larvae infected with untreated (non-sialylated) and sialylated bacteria (Figure S2).
Figure 4. A) Infection of Galleria mellonella with different phenotypic variants of Neisseria gonorrhoeae strain P9. Healthy larva (n = 10 per group) were infected with different P9 variants (~6-8 × 107 CFU per larva of P9-1 Pil- Opa-; P9-2 Pil+ Opa-; P9-16 Pil- Opa+; P9-17 Pil+ Opab+), incubated at 37°C and examined for survival over a period of 48 h. Symbols represent mean survival from a minimum of 3 independent experiments and error bars represent the standard deviation of the mean. Unpaired t test was done at each time point to compare survival of larvae infected with P9 variants and control, GC-injected larvae. * denotes statistical significance with P < 0.01. B) Infection of Galleria mellonella with different Neisseria gonorrhoeae isolates. Healthy larva (n = 10 per group) infected with different gonococcal isolates (~6-8 × 107 CFU per larva), incubated at 37°C and examined for survival over a period of 48 h. Symbols represent the mean survival from a minimum of 3 independent experiments and the error bars represent the standard deviation of the mean. Unpaired t test was done to compare survival of larvae infected with the different isolates and control, uninfected larvae injected with GC broth alone. * denotes statistical significance with P < 0.001. In addition, Unpaired t test was done to compare survival of the larvae infected with the different isolates compared to P9-17 (see text for statistical significance)
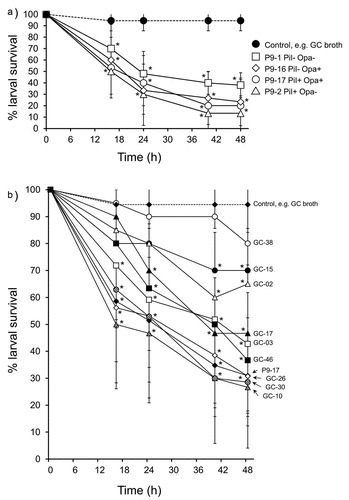
We also used the model to examine the virulence of other gonococcal isolates, in this case, of several representative isolates from the CDC/FDA AR Panel with reported high Minimum Inhibitory Concentration (MIC) values to ceftriaxone and azithromycin, the antibiotics recommended for treating gonorrhea ()). Larvae were infected with OD 0.8 (~8 x 107 CFU/larva) of 9 different isolates and also with P9-17, and survival recorded over 48 h. Compared with larvae injected with supplemented GC broth alone, survival of larvae was significantly reduced by 48 h after injection with all of the isolates (P < 0.001) except for GC-38, which did not induce significant death of larvae (P > 0.05). Isolates GC-03, −10, −17, −26, −30, −46 and P9-17 were toxic to larvae, with an average of 30–45% of larvae surviving after 48 h. By contrast, isolates GC-02, −15 and −38 appeared to be less virulent, with an average of ~70-85% of larvae surviving bacterial challenge after 48 h ()).
Killing of G. mellonella requires infection with live gonococci
Larvae were injected with a dose of OD 0.8 (~8 x 107 CFU) live N. gonorrhoeae P9-17/larva or with a similar dose of killed (heat-inactivated, HI) bacteria. Survival of larvae was significantly lower after infection with live gonococci compared with HI-bacteria at all the time points (P < 0.05). As expected, survival of larvae infected with live bacteria was ~20% by 48 h (P < 0.01), whereas ~70% of larvae survived after injection of HI-bacteria (P < 0.01) (), when compared with control larvae injected with supplemented GC broth only.
Figure 5. A) The effect of heat-killed and live inocula of Neisseria gonorrhoeae on the survival of G. mellonella. Healthy larva (n = 10 per group) were infected with heat-killed or live N. gonorrhoeae strain P9-17 gonococci (~8 × 107 CFU per larva), incubated at 37°C and examined for survival by response to touch over a period of 48 h. Symbols represent the mean survival from a minimum of 3 independent experiments and the error bars represent the standard deviation of the mean. Unpaired t test was done to compare survival between the two groups at all the time points where * denotes statistical significance with P < 0.05. Unpaired t test was also done to compare survival of larvae infected with heat-killed or live bacteria and with control larvae injected with GC broth, where ** denotes statistical significance with P < 0.01. B) The effect of Neisseria gonorrhoeae P9-17 Outer Membranes (OM) on the survival of Galleria mellonella. Healthy larva (n = 10 per group) were injected with varying doses of OM (up to 80,000 ng per larva), incubated at 37°C and examined for survival by response to touch over a period of 48 h. Symbols represent the mean survival from a minimum of 3 independent experiments and the error bars represent the standard deviation of the mean. Unpaired t test was done at each time point to compare survival of larvae injected with OM and control group injected with GC broth. * denotes statistical significance with P < 0.01
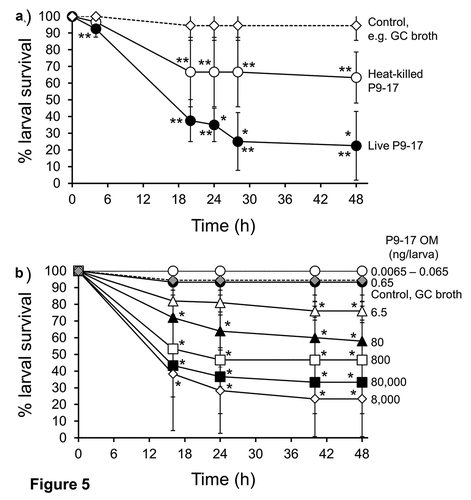
The release of OM by N. gonorrhoeae is a recognized virulence mechanism during infection [Citation47] and we tested the hypothesis that gonococcal OM vesicles could induce larval death. In our first experiments, larvae were injected with N. gonorrhoeae P9-17 OM doses from 0.0065 to 6.5 ng/larvae, which would approximate roughly to 6.5 × 105–6.5 × 108 Neisseria bacteria, based on LOS content [Citation48,Citation49]. Doses of 0.0065–0.65 ng OM/larva did not significantly affect survival of larvae compared with supplemented GC control injected larvae (P > 0.05). Significant death of ~25% of the larvae was first observed with a dose of 6.5 ng/larva (P < 0.01). Injection of a physiological excess of OM from between 80 and 800 ng OM/larva reduced survival of larvae by ~50% (P < 0.01) by 48 h, and doses of 8000–80,000 ng OM/larva reducing larval survival to <30% (P < 0.01) by 48 h ()).
Infection of G. mellonella with other Neisseria spp
We tested the hypothesis that other Neisseria species, that is, N. meningitidis and N. lactamica, could also infect Galleria mellonella larvae, but that they would show differences in virulence compared with each other and with gonococci. Groups of larvae were infected with different doses of N. gonorrhoeae P9-17, N. meningitidis MC58 or N. lactamica Y92-1009 and survival recorded over 48 h. At a dose of OD 0.1 (~105 CFU/larva), there was no significant difference in survival of the larvae compared to larvae injected with supplemented GC broth alone (P > 0.05). Increasing doses of OD 0.4–1.0 (~ 0.5 × 106 – ~108 CFU) of all three bacteria decreased larval survival significantly (P < 0.02) (). Although there seemed to be a trend of increased virulence of meningococci over both gonococci and N. lactamica, it was not statistically significant (P > 0.05). To give an idea of the relative toxicity of the Neisseriae, we also tested P. aeruginosa PAO-1 in parallel, using doses from 150 CFU/larva up to ~2.5 × 105 CFU/larva (Figure S3). Compared to control larvae injected with supplemented GC broth alone, P. aeruginosa significantly reduced larval survival (P < 0.01) with all doses tested, with no survival by 12–18 h in any of the groups.
Figure 6. Survival of Galleria mellonella infected with other Neisseria species. Healthy larva (n = 10 per group) were infected with differing doses N. gonorrhoeae, N. meningitidis, or N. lactamica, incubated at 37°C and examined for survival by response to touch over a period of 48 h. Symbols represent the mean survival from a minimum of 3 independent experiments and error bars represent the standard deviation of the mean. Unpaired t test was done to compare survival of larvae infected with the different Neisseria spp. and the control GC broth group at each time point. * denotes statistical significance with P < 0.02. Unpaired t test was also done at each time point to compare between the different Neisseria spp. on survival of G. mellonella. ns, not significant p > 0.05
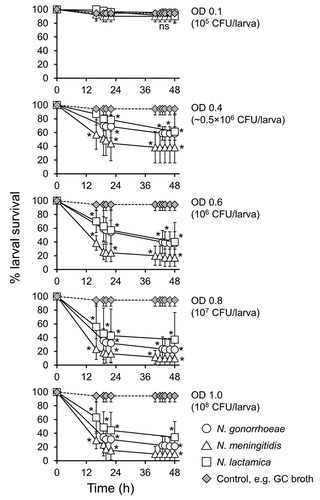
Effects of priming G. mellonella larvae with nontoxic doses of N. gonorrhoeae
In our study, we tested the hypothesis that pre-exposure of G. mellonella larvae to nontoxic doses of gonococci protected the larvae from subsequent challenge with various doses of the same organism. Larvae were primed with the nontoxic dose of 0.1 OD (~105 CFU/larvae) N. gonorrhoeae P9-17 and after 16 h they were injected with 0.1 OD, 0.4 OD (~8 × 106 CFU/larvae) or 0.8 OD (~8 × 107 CFU/larvae) of P9-17. Larvae were also injected with the higher doses of 0.4 and 0.8 OD as controls. Larval survival was followed for 48 h. As expected, doses of 0.1 and 0.4 OD showed little significant killing of larvae, whereas only ~30% of larvae survived after infection with OD 0.8 by 48 h, compared to larvae injected with supplemented GC broth only (P < 0.05) (- c). When we injected an OD 0.1 dose of P9-17 into primed larvae, larval survival was reduced (P < 0.05) to 60% by 48 h, compared to the non-primed larvae infected with OD 0.1 (). With an OD 0.4 dose, there was a greater rapid decline in survival of primed larvae and they were all dead by 12 h () (P < 0.05). Even compared to the higher toxic dose of OD 0.8, primed larvae injected with the same dose were more susceptible, with >90% death of the larvae by 4 h and no survivors by 16 h (P < 0.05) ().
Figure 7. Effects of priming Galleria mellonella larvae with Neisseria gonorrhoeae. Healthy larvae (n = 10 per group) were primed by injecting with a non-killing dose of N. gonorrhoeae strain P9-17 at −16 h (OD 0.1, ~105 CFU per larva) and then infected at 0 h with A) 0.1 OD, ~105 CFU per larva, B) 0.4 OD, ~106 CFU per larva, C) 0.8 OD, ~107 CFU per larva, or D) 0.8 OD ~107 CFU per larva of L. gasseri. Controls included infection with OD 0.1 A), 0.4 B), C) 0.8 and D) 0.8 of bacteria alone from −16 h. Larvae were incubated at 37°C and examined for survival by response to touch over a period of 48 h. Unpaired t test was done to compare survival of larvae in the primed and infected groups with the control group infected with the bacteria alone. * denotes statistical significance with P < 0.05. In E), larvae were primed at −16 h with an OD 0.1 dose of N. gonorrhoeae P9-17 and challenged at 0 h with OD 0.8 of heat-inactivated (HI) P9-17 or HI L. gasseri. Unpaired t test was done to compare survival of larvae between the different treatments, with statistical significance denoted by P < 0.05 (see text for details). Symbols in each panel represent the mean survival from a minimum of 3 independent experiments and the error bars represent the standard deviation of the mean
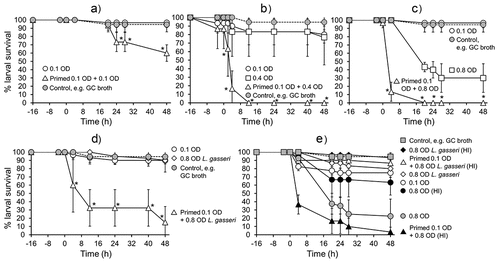
We tested next the hypothesis that a heterologous bacterium that is ordinarily nontoxic to G. mellonella could be rendered toxic to larvae that had been pre-exposed to gonococci. We examined a trio of Lactobacillus spp., which are common commensals of the female genital tract microbiome that may play niche-occupying and protective roles against sexually transmitted infections [Citation50]. We compared L. brevis, L. crispatus, and L. gasseri and found that both L. brevis and L. crispatus significantly reduced larval survival at all doses tested (OD 0.1–1.0, ~105 – 108 CFU/larva) (P < 0.01), compared with larvae injected with supplemented GC broth alone, whereas >80% of all larvae injected with even the highest doses of L. gasseri (OD 0.8 − 1.0, ~107 – 108 CFU/larva) survived (P > 0.05) (Figure S4a). Thus, to test the hypothesis, we primed larvae with an OD 0.1 dose of N. gonorrhoeae P9-17 and then challenged them with a high dose (OD 0.8, ~107 CFU/larva) of the nontoxic L. gasseri. clearly shows that doses of OD 0.1 P9-17 and OD 0.8 L. gasseri are not toxic, with >90% survival of larvae by 48 h, compared to larvae injected with supplemented GC broth alone (P > 0.05). By contrast, injection of OD 0.8 L. gasseri into gonococcal-primed larvae resulted in rapid death of the larvae, with only 30% surviving by 12 h and ≤10% by 48 h (P < 0.05).
Our experiments described in showed that live gonococci were necessary to kill G. mellonella larvae. We tested the hypothesis that injection of HI-gonococci or HI-L. gasseri similarly did not kill larvae that had been pre-exposed to gonococci. In these experiments, we primed larvae with a nontoxic OD 0.1 dose (~0.7 × 105 CFU/larva) of N. gonorrhoeae P9-17 and challenged them after 16 h with high doses of OD 0.8 of HI-gonococci or HI-L. gasseri bacteria (~107 – 108 CFU/larva) (). As expected, when compared to larvae injected with supplemented GC broth alone, ~70-80% of larvae survived after injection of OD 0.1 of live P9-17 or OD 0.8 of HI-gonococci by 48 h (P > 0.05) (), whereas only ~20% of larvae survived after injection of OD 0.8 of live gonococci (P < 0.05). Injection of OD 0.8 of HI-gonococci into larvae pre-exposed to gonococci resulted in significant larval death, with no survivors by 48 h (P < 0.05). In contrast, the injection of OD 0.8 of HI-L. gasseri was nontoxic for gonococcal-primed larvae, with >90% larval survival at 48 h () (P > 0.05).
One possible explanation for our observations is that exposure of larvae to nontoxic doses of gonococci affects the larval innate immune response system, possibly by engaging hemocytes and making them unavailable to control any secondary gonococcal exposure. To test this hypothesis, we injected larvae with various doses of liposomes (Lip) containing dichloromethylene-bisphosphonate (clodronate, CDLip) in order to try and deplete hemocytes, and after 16 h we exposed the larvae to various doses of N. gonorrhoeae P9-17 and measured larval survival over 48 h. Lip and CDLip alone showed no significant toxicity toward larvae (<10% death over 64 h) (Figure S5). Larval survival was significantly reduced in gonococcal-infected larvae that had been pre-treated with CDLip, compared with gonococcal-infected larvae pre-treated with Lip. For example, with an OD of 0.1 (~0.7 × 105 CFU/larva), the survival of CDLip-treated larvae by 48 h was reduced by ~20-50% with neat (P = 0.05) and 1/5 dilution (P < 0.02) of CDLip, respectively. With an OD of 0.4 (~8 × 106 CFU/larva), despite the wide error bars, survival of larvae treated with both doses of CDLip was reduced by ~50% by 48 h. Furthermore, even with the highest dose of OD 0.8 (~8 × 107 CFU/larva) gonococci tested, CDLip pre-treated larvae showed significantly lower levels of survival: by 48 h, there was ~40-50% survival amongst Lip-pre-treated larvae, and both neat and 1/5 doses of CDLip reduced survival significantly to <10% by 48 h (P < 0.05) (Figure S5).
G. mellonella as a model to examine the efficacy of antimicrobials to treat N. gonorrhoeae infection
There is a large and increasing literature describing the use of the G. mellonella model for testing antibiotics [Citation13,Citation31] and we tested the hypothesis that it would be useful for testing the in vivo efficacy of the clinically recommended antibiotics ceftriaxone and azithromycin. In preliminary experiments, we observed that neither ceftriaxone nor azithromycin had any significant effect on survival of larvae (P > 0.05), even when tested at doses as high as ~1000 μg/mL (i.e., 10 μg/larva) (Figure S6).
We next infected groups of larvae with different gonococcal strains from the CDC/FDA AR Panel (OD 0.8, ~8 x 107 CFU/larva) that showed high levels of resistance () to ceftriaxone and azithromycin and at the same time we administered various doses of the antibiotics alone, or together, to test their efficacy in reducing death. The antibiotic doses chosen reflected the MIC values and higher doses if the MIC values were ineffective. Time course experiments showed that ~30% of larvae survived infection with any of the gonococcal strains by 48 h in the no-antibiotic controls, compared to larvae injected with supplemented GC broth alone (P < 0.05) (). There was variation in the ability of ceftriaxone to improve survival in larvae infected with the different isolates, compared to untreated and infected larvae. Doses of >1 μg/mL, >0.625 μg/mL and 3.125 μg/mL of ceftriaxone significantly increased survival of P9-17-, GC-10- and GC-26-infected larvae by an average of 40–60%, respectively, by 48 h (P < 0.05). With isolate GC-03, a low dose of 0.1 μg/mL of ceftriaxone improved survival of larvae by ~40% (P < 0.03), but increasing doses were contra-indicated. However, ceftriaxone did not improve survival of larvae infected with either GC-17 or GC-30, even tested at doses as high as 60 μg/mL for the former and 1000 μg/mL for the latter (P > 0.05) ().
Table 4. Summary of antibiotic resistance profiles of gonococcal isolates used in this study
Figure 8. Effects of treating Neisseria gonorrhoeae-infected Galleria mellonella with antibiotics ceftriaxone and azithromycin. Healthy larva (n = 10 per group) were infected with different gonococcal isolates (OD 0.8, ~108 CFU per larva) and injected simultaneously with different doses of ceftriazone or azithromycin antibiotic, incubated at 37°C and examined for survival by response to touch over a period of 48 h. The symbols represent mean survival from a minimum of 3 independent experiments and the error bars represent the standard deviation of the mean. Unpaired t test was done at each time point to compare survival of gonococcal-infected larvae without antibiotic and the survival of gonococcal-infected larvae treated with antibiotic. * denotes statistical significance with P < 0.05
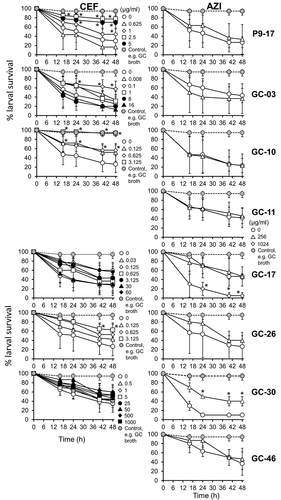
Treatment of larvae with a high dose of azithromycin (256 μg/mL) did not increase the percentage of survivors amongst larvae infected with OD 0.8 of gonococcal strains P9-17, GC-03, GC-10, GC-11, GC-17, GC-26, or GC-46 (). However, azithromycin showed some efficacy in larvae infected with strain GC-30, with larval survival improved by ~30% (P < 0.05). Interestingly, a higher dose of 1024 μg/mL reduced larval survival significantly (P < 0.05) in the presence of isolate GC-17, suggesting a toxic synergism (). This higher dose was not tested with the other isolates.
Finally, we tested the efficacy of dual treatment with ceftriaxone and azithromycin in reducing death in larvae that had been infected with OD 0.8 of strains P9-17, GC10, GC-26, or GC-30 (Figure S7). There was a suggestion of synergism between ceftriaxone and azithromycin, but this was isolate-specific and only apparent with low doses of ceftriaxone used. For example, a dose of 0.625 μg/mL ceftriaxone did not protect larvae from killing by P9-17, but addition of azithromycin significantly improved survival by ~70%, despite azithromycin alone having no effect. Similarly, with isolate GC-10, survival of larvae treated with 0.625 μg/mL of ceftriaxone was increased by ~25% when the same dose was administered with azithromycin. For both isolates, where larval survival was improved with increased doses of ceftriaxone, the presence of azithromycin had no significant effect (P > 0.05). By contrast, administration of azithromycin with ceftriaxone to treat larvae infected with isolates GC-26 and GC-30 had no significant effect (P > 0.05). summarizes the antibiotic testing data for and Figure S7.
Table 5. Summary of the antibiotic testing data shown in and Figure S7
There is also an increasing literature describing the use of the G. mellonella model to test the bioactivity of novel antimicrobials, for example, manganese(i) tricarbonyl complexes, theaflavin-epicatechin combinations, and carbon monoxide-releasing molecule [Mn(CO)3(tpa-kappa3N)]Br, to cite a few [Citation51–53]. We used the model to examine whether two novel antimicrobial compounds, monocaprin [Citation54] and 5-Mercapto-2-nitrobenzoic Acid-Coated Silver Nanoclusters (MNBA-AgNCs) [Citation42] could stop systemic gonococcal infection in vivo. In the current study, we infected G. mellonella with 0.8 OD (~8 × 107 CFU/larva) of N. gonorrhoeae P9-17 and then treated larvae with various doses of the compounds. Neither monocaprin (0.1–50 mM doses) nor MNBA-AgNCs (0.467–58.44 µM doses) improved the survival of infected larvae (Figure S8), compared to untreated, infected larvae (P > 0.05). In addition, compared to larvae injected with supplemented GC broth alone, monocaprin was significantly toxic at the highest dose of 50 mM tested, reducing larval survival by 80% (P < 0.05). By contrast, the MNBA-AgNCs showed no toxicity (P > 0.05) (Figure S8).
Discussion
The key findings from this initial descriptive study are that N. gonorrhoeae can infect and kill G. mellonella larvae and that increasing gonococcal toxicity correlated with reduced health index scores and pronounced histopathological changes such as increases in the total lesion grade, melanized nodules, hemocyte reaction, and multifocal adipose body degeneration. The cytopathic effect required injection of live bacteria and could be abrogated by heat-killing; moreover, killing could be induced by isolated LOS-replete OM, but only when used at physiologically excess amounts. Larval death was also independent of the expression of pilus or Opa protein and of sialylation of LOS glycans, within a single gonococcal species studied. In the current study, we only used a single dose of ~8 × 107 CFU/larva to compare the different pilus and Opa variants, and future studies could test other doses, which may potentially highlight some differences in pathology, and also the contribution of other possible adhesins. However, the model could demonstrate relative toxicity of different isolates; the majority of the isolates we tested showed comparable toxicity as P9-17, but we also identified isolates that showed reduced toxicity and one that was avirulent. The explanation for this observed variability in toxicity is not clear and may be related to other phenotypic differences than pilus or Opa expression, or growth rate.
There was no difference between gonococci and other Neisseria spp., that is, meningococci and lactamica, in their ability to kill the larvae. By contrast, P. aeruginosa, which has been exhaustively studied in the larval model, was significantly more cytotoxic to the larvae than gonococci. This is unsurprising, as P. aeruginosa possesses an arsenal of virulence factors and toxins [Citation55] and displays a faster growth rate than gonococci. Surprisingly, we found that L. brevis and L. crispatus killed larvae like gonococci, whereas L. gasseri did not. The reasons for this discrepancy are unclear, but could be related to variable production of lactic acid, which is known to kill G. mellonella larvae, and/or the differing cell surface architecture, expression and/or secretion of other compounds by these different Lactobacillus spp [Citation56].
In simple co-infection experiments in our study, we found that L. gasseri failed to improve the survival of G. mellonella infected with P9-17 (Figure S4b). However, several studies have reported that Lactobacillus spp. can inhibit gonococcal adherence to epithelial cells in vitro [Citation57–59] and that the generation of an acidic environment of pH <4.0 associated with Lactobacillus metabolism was a major mechanism for counter-acting gonococcal growth [Citation60]. Other mechanisms may include the production of surfactants [Citation60], and bacteriocin-like substances [Citation61] and acidic compounds that have been reported to inhibit GC viability in vitro [Citation62] and in a porcine vagina mucosa model [Citation63], respectively. In light of these reported biological effects of lactobacilli on gonococci, further studies could include pH adjustment and the use of other Lactobacillus spp.
Priming injection of low doses of microorganisms has been reported in several studies to apparently protect G. mellonella larvae from subsequent repeated exposure to the same microorganism or other organisms [Citation35,Citation37,Citation38,Citation64–68]. Conversely, in our study, gonococcal-primed larvae were more susceptible to the pathogenic effects of secondary exposure to both live gonococci and killed gonococci. Moreover, the effect was not organism-specific, and nontoxic L. gasseri could kill gonococcal-primed larvae, but only when injected as live organisms and not killed organisms. A possible explanation for the observed difference in the effects of killed gonococci and killed L. gasseri is that that gonococci contain heat-stable components that are toxic to hemocyte-depleted larvae. It is also possible that the priming event engages the innate response of the resident larval population of hemocytes, which are then unavailable to potentially control subsequent infection. Many studies have demonstrated the binding of bacterial pathogens to larval hemocytes and their subsequent uptake, for example recent studies with Yersinia pseudotuberculosis [Citation69], Salmonella Enteritidis [Citation70] and Coxiella burnetti [Citation71]. In preliminary confocal experiments we did see an association of gonococci with hemocytes in extracted hemolymph (data not shown), but more detailed studies are necessary to examine these bacterial-larval innate cell interactions, and are outside the scope of the current manuscript.
In our study, larvae were used to examine the efficacy of the clinically used antibiotics ceftriaxone and azithromycin to improve survival of gonococcal-infected larvae. We did not measure bacterial burden within the larvae as the surrogate measure of antimicrobial efficacy, as the numbers of recovered gonococci generally declined over time in untreated larvae, which may have made it more difficult to demonstrate statistically significant antimicrobial activity. Ceftriaxone significantly protected larvae from gonococcal infection. This protection was dose-dependent and varied between the isolates tested, and doses higher than the reported in vitro MIC values were necessary for a biological effect in vivo. Conversely, azithromycin was ineffective, and even toxic to the larvae when administered in high doses. Previously, we showed that two novel antimicrobial compounds, monocaprin [Citation54] and MNBA-AgNCs [Citation42], were both highly efficient in killing gonococci in vitro. Both compounds were developed as topical antimicrobials to treat gonococcal infections and showed no efficacy systemically in preventing gonococcal-induced larval death. Interestingly, a recent study has reported that a different silver nanoparticle preparation that did not carry a bioactive ligand, such as MNBA, could increase the survival of larvae infected with P. aeruginosa [Citation72]. The larval model therefore seems to be useful for comparing nanoparticle compounds, and disparities in bioactivity may reflect different mechanisms of action in vivo. Furthermore, the larval model in our study did provide an indication of the toxicity of the novel compounds in vivo.
The Galleria model has advantages over other models for testing the efficacy and toxicity of novel antimicrobials [Citation13]. In drug development, novel antimicrobials are screened initially in vitro to determine MICs against pathogens and their toxicity is evaluated in cultured mammalian cell lines. Successful compounds then usually progress to animal infection models to examine in vivo efficacy and also to examine pharmokinetic and pharmocodynamic properties. Although necessary, gonococcal, and other pathogen-testing animal models are expensive, time-consuming, and challenging for screening large numbers of compounds that initially are successful in in vitro studies. By contrast, the Galleria model is a simple, cheap, and highly reproducible alternative for rapid in vivo pre-screening of antimicrobial compounds, and offers a bridge between the in vitro studies and final animal testing. Potentially, the model could identify and exclude compounds that are either ineffective in vivo despite promising MIC determinations and/or show toxicity, and thus reduce the number of compounds that go forward to testing in animal infection models. Thus, pre-screening using the Galleria model could lead to a reduction in animal use and supports the 3Rs principles of Replacement, Reduction, and Refinement.
In summary, we have shown that N. gonorrhoeae can infect Galleria mellonella larvae and induce pathological changes that result in larval death. However, the model has some clear limitations. Such larvae are not a natural target for infection with Neisseria spp. Indeed, gonococci did not appear to be multiplying within larvae and CFU numbers gradually declined over time in the hemolymph, although future studies would also examine larval feces for the presence of gonococci. The most probable explanation is that the larvae may not express the specific receptors that make them significantly labile to gonococcal interactions, for example, the presence of pilus-binding receptors, or the presence of CEACAM or heparin sulfate proteoglycan (HSPG) receptors for Opa [Citation73,Citation74]. However, insects are known to produce HSPG molecules [Citation75] and mucopolysaccharides have been reported in Galleria [Citation76], and further studies would be needed to examine whether these molecules are involved with binding Opa proteins. In addition, the target receptor of the Opab variant expressed by strain P9-17 is unknown and would need to be determined. Another possible explanation is that Galleria do not possess the specific innate immune responses that can recognize low levels of Pathogen Associated Molecular Patterns (PAMPs) such as Neisseria LOS that are indicative of the start of an infectious process. The exact mechanism of inducing larval death is not known, but may involve other toxic components of the gonococcus, for example, the potential release of peptidoglycan fragments or other potential toxins [Citation77,Citation78]. The model may therefore not prove useful for studying gonococcal adhesion and invasion events in vivo, but may provide a method for assessing putative toxins/virulence factors, without resorting to cell cultures and mammalian models.
We also attempted to develop the model as an in vivo bactericidal assay, by co-injecting live bacteria, antibodies to OM with demonstrable bactericidal activity in vitro [Citation79] and human serum complement, and then examining survival over time (data not shown). This proved unviable as a strategy due to the limitations of the volume of sample that can be injected into the larvae without causing swelling and stress. Furthermore, the doses of gonococci required to induce pathology are high, compared with other pathogens. Further possible limitations of the study are the provenance of the waxmoth larvae, whether they have residual antibiotics or whether other commensal bacteria might interfere: thus, future mechanistic studies would make use of untreated larvae with known flora and these are becoming increasingly available from scientific suppliers, albeit at considerably higher cost. Regardless, our expectation for the long-term applicability of the G. mellonella larval model is that it may prove most useful as part of the drug discovery pipeline for in vivo screening of novel antimicrobials for bioactivity against gonococci and for toxicity, prior to studies in mammalian models.
Author contributions
Conceived and designed the experiments: MC, AD, MVH, EB, RMLR.
Performed the experiments: AD, EB.
Analysed the data: MC, AD, MVH, EB, RMLR.
Wrote the paper: MC, AD, MVH, EB, RMLR.
Reviewed and approved the manuscript: MC, AD, MVH, EB, RMLR.
Supplemental Material
Download MS Word (16.3 KB)Supplemental Material
Download Zip (3.4 MB)Acknowledgments
We thank Dr Sandra Wilks, Biological Sciences, University of Southampton, for providing the Lactobacillus ATCC/NCTC isolates. We also thank Dr. Maria Isabel Lucio and Professor Antonios G. Kanaras, Physics and Astronomy, Faculty of Physical Sciences and Engineering, University of Southampton, for the MNBA-AgNC compound for testing. Some of these data were presented in a poster at the Microbiology Society Annual Conference, 2020, and an abstract reported in Access Microbiology, Vol. 2, Issue 7A (Aiste Dijokaite, Victoria-Maria Humbert, Myron Christodoulides. Galleria mellonella – a novel infection model for the study of Neisseria gonorrhoeae virulence and pathogenicity” Open Access First Published: 10 July 2020 10.1099/acmi.ac2020.po1042).
Disclosure statement
Aiste Dijokaite is a student at the University of Southampton and participated in a postgraduate studentship program at GlaxoSmithKline (GSK): the studentship was sponsored by GSK (80%) and the University of Southampton (20%). The authors report no other conflicts of interest.
Data availability statement
Data generated for this study have been deposited with the University of Southampton, 10.5258/SOTON/D1818.
Supplementary material
Supplemental data for this article can be accessed here
Additional information
Funding
References
- Rowley J, Vander Hoorn S, Korenromp E, et al. Chlamydia, gonorrhoea, trichomoniasis and syphilis: global prevalence and incidence estimates, 2016. Bull World Health Organ. 2019 Aug 1;97(8):548–562.
- Mayor MT, Roett MA, Uduhiri KA. Diagnosis and management of gonococcal infections. Am Fam Physician. 2012 Nov 15;86(10):931–938.
- Humbert MV, Christodoulides M. Atypical, yet not infrequent, infections with Neisseria species. Pathogens. 2019 Dec 20;9(1):10.
- Hobbs MM, Sparling PF, Cohen MS, et al. Experimental gonococcal infection in male volunteers: cumulative experience with Neisseria gonorrhoeae strains FA1090 and MS11mkC. Front Microbiol. 2011;2:123.
- Rice PA, Shafer WM, Ram S, et al. Neisseria gonorrhoeae: drug resistance, mouse models, and vaccine development. Annu Rev Microbiol. 2017 Sep 08;71(1):665–686.
- Islam EA, Anipindi VC, Francis I, et al. Specific binding to differentially expressed human carcinoembryonic antigen-related cell adhesion molecules determines the outcome of Neisseria gonorrhoeae infections along the female reproductive tract. Infect Immun. 2018 Aug;86(8). doi:10.1128/IAI.00092-18.
- Kaser T, Renois F, Wilson HL, et al. Contribution of the swine model in the study of human sexually transmitted infections. Infect Genet Evol. 2018 Dec;66:346–360.
- Christodoulides M. Neisseria gonorrhoeae: methods and protocols. Christodoulides M, editor. New York: Springer/Humana Press. 2019. Walker J, editor. Methods in Molecular Biology.
- Ma M, Powell DA, Weyand NJ, et al. A natural mouse model for Neisseria colonization. Infect Immun. 2018 May;86(5). doi:10.1128/IAI.00839-17.
- Unemo M, Del Rio C, Shafer WM. Antimicrobial resistance expressed by Neisseria gonorrhoeae: a major global public health problem in the 21st century. Microbiol Spectr. 2016 Jun;4(3):DOI:10.1128/microbiolspec.EI10-0009-2015
- Chantawannakul P, De Guzman LI, Li JL, et al. Parasites, pathogens, and pests of honeybees in Asia. Apidologie. 2016 May;47(3):301–324.
- Ellis JD, Graham JR, Mortensen A. Standard methods for wax moth research. J Apicult Res. 2013;52(1):1–17.
- Tsai CJ, Loh JM, Proft T. Galleria mellonella infection models for the study of bacterial diseases and for antimicrobial drug testing. Virulence. 2016 Apr 2;7(3):214–229.
- Vilmos P, Kurucz E. Insect immunity: evolutionary roots of the mammalian innate immune system. Immunol Lett. 1998 Jun;62(2):59–66.
- Ratcliffe NA. Invertebrate immunity--a primer for the non-specialist. Immunol Lett. 1985;10(5):253–270.
- Soderhall K, Cerenius L. Role of the prophenoloxidase-activating system in invertebrate immunity. Curr Opin Immunol. 1998 Feb;10(1):23–28.
- Kavanagh K, Reeves EP. Exploiting the potential of insects for in vivo pathogenicity testing of microbial pathogens. FEMS Microbiol Rev. 2004 Feb;28(1):101–112.
- Wilson R, Ratcliffe NA. Effect of lysozyme on the lectin-mediated phagocytosis of Bacillus cereus by haemocytes of the cockroach, Blaberus discoidalis. J Insect Physiol. 2000 May 1;46(5):663–670.
- Lysenko O. The mechanism of pathogenicity of Pseudomonas aeruginosa (Schroeter) Migula. I. The pathogenicity of the strain N 06 to the wax moth larvae. J Insect Pathol. 1963;5.
- Li Y, Spiropoulos J, Cooley W, et al. Galleria mellonella - a novel infection model for the Mycobacterium tuberculosis complex. Virulence. 2018;9(1):1126–1137.
- Schell MA, Lipscomb L, DeShazer D. Comparative genomics and an insect model rapidly identify novel virulence genes of Burkholderia mallei. J Bact. 2008 Apr;190(7):2306–2313.
- Peleg AY, Monga D, Pillai S, et al. Reduced susceptibility to vancomycin influences pathogenicity in Staphylococcus aureus Infection. J Infect Dis. 2009 Feb;199(4):532–536.
- Mukherjee K, Altincicek B, Hain T, et al. Galleria mellonella as a model system for studying Listeria pathogenesis. Appl Environ Microbiol. 2010 Jan;76(1):310–317.
- Evans BA, Rozen DE. A Streptococcus pneumoniae infection model in larvae of the wax moth Galleria mellonella. Eur J Clin Micro Infect Dis. 2012 Oct;31(10):2653–2660.
- Pereira MF, Rossi CC, Vieira de Queiroz M, et al. Galleria mellonella is an effective model to study Actinobacillus pleuropneumoniae infection. Microbiology (Reading). 2015 Feb;161(Pt 2):387–400.
- Barnoy S, Gancz H, Zhu Y, et al. The Galleria mellonella larvae as an in vivo model for evaluation of Shigella virulence. Gut Microbes. 2017 Jul 4;8(4):335–350.
- Yang HF, Pan AJ, Hu LF, et al. Galleria mellonella as an in vivo model for assessing the efficacy of antimicrobial agents against Enterobacter cloacae infection. J Micro Imm Infect. 2017 Feb;50(1):55–61.
- White AG, Gibson NHE, Wolf J. A method for measuring dose of a pathogen ingested by larvae of greater wax moth Galleria mellonella, in bioassays of Bacillus thuringiensis. J Invert Path. 1970;15(2):196.
- Cotter G, Doyle S, Kavanagh K. Development of an insect model for the in vivo pathogenicity testing of yeasts. FEMS Immunol Med Micro. 2000 Feb;27(2):163–169.
- Torrecilhas AC, Xander P, Ferreira KS, et al. Alternative host models for testing anti-protozoal or antifungal compounds and fungal infection. Current Top Med Chem. 2018;18(4):300–311.
- Cutuli MA, Petronio Petronio G, Vergalito F, et al. Galleria mellonella as a consolidated in vivo model hosts: new developments in antibacterial strategies and novel drug testing. Virulence. 2019;10(1):527–541. 2019/01/01.
- Zak K, Diaz JL, Jackson D, et al. Antigenic variation during infection with Neisseria gonorrhoeae: detection of antibodies to surface proteins in sera of patients with gonorrhea. J Infect Dis. 1984;1984(149):166–173.
- Williams JN, Skipp PJ, Humphries HE, et al. Proteomic analysis of outer membranes and vesicles from wild-type serogroup B Neisseria meningitidis and a lipopolysaccharide-deficient mutant. Infect Immun. 2007 2007;75(3):1364–1372.
- Loh JM, Adenwalla N, Wiles S, et al. Galleria mellonella larvae as an infection model for group A streptococcus. Virulence. 2013 Jul 1;4(5):419–428.
- Santos RB, Scorzoni L, Namba AM, et al. Lactobacillus species increase the survival of Galleria mellonella infected with Candida albicans and non-albicans Candida clinical isolates. Med Mycol. 2019 Apr 1;57(3):391–394.
- Scalfaro C, Iacobino A, Nardis C, et al. Galleria mellonella as an in vivo model for assessing the protective activity of probiotics against gastrointestinal bacterial pathogens. FEMS Micro Lett. 2017 Apr 1;364(7). 10.1093/femsle/fnx064
- Bergin D, Murphy L, Keenan J, et al. Pre-exposure to yeast protects larvae of Galleria mellonella from a subsequent lethal infection by Candida albicans and is mediated by the increased expression of antimicrobial peptides. Microbes Infect. 2006 Jul;8(8):2105–2112.
- Taszlow P, Vertyporokh L, Wojda I. Humoral immune response of Galleria mellonella after repeated infection with Bacillus thuringiensis. J Invertebr Pathol. 2017 Oct;149:87–96.
- Kameka AM, Haddadi S, Jamaldeen FJ, et al. Clodronate treatment significantly depletes macrophages in chickens. Can J Vet Res. 2014 Oct;78(4):274–282.
- Kwon H, Smith RC. Chemical depletion of phagocytic immune cells in Anopheles gambiae reveals dual roles of mosquito hemocytes in anti-Plasmodium immunity. Proc Natl Acad Sci U S A. 2019 Jul 9;116(28):14119–14128.
- Ignasiak K, Maxwell A. Galleria mellonella (greater wax moth) larvae as a model for antibiotic susceptibility testing and acute toxicity trials. BMC Res Notes. 2017 Aug 29;10(1):428.
- Lucio MI, Kyriazi ME, Hamilton J, et al. Bactericidal effect of 5-Mercapto-2-nitrobenzoic Acid-Coated silver nanoclusters against multidrug-resistant Neisseria gonorrhoeae. ACS Appl Mater Interfaces. 2020 Jun 24;12(25):27994–28003.
- Churchward CP, Alany RG, Kirk RS, et al. Prevention of Ophthalmia Neonatorum Caused by Neisseria gonorrhoeae Using a fatty acid-based formulation. mBio. 2017 Jul 25;8(4). DOI:10.1128/mBio.00534-17.
- Foerster S, Gustafsson TN, Brochado AR, et al. The first wide-scale drug repurposing screen using the prestwick chemical library (1200 bioactive molecules) against Neisseria gonorrhoeae identifies high in vitro activity of auranofin and many additional drugs. APMIS. 2020 Mar;128(3):242–250.
- Merz AJ, So M. Interactions of pathogenic Neisseriae with epithelial cell membranes. Annu Rev Cell Dev Biol. 2000;2000(16):423–457.
- Wetzler LM, Barry K, Blake MS, et al. Gonococcal lipooligosaccharide sialylation prevents complement-dependent killing by immune sera. Infect Immun. 1992;60(1):39–43.
- Francis IP, Lui X, Wetzler LM. Isolation of naturally released gonococcal outer membrane vesicles as vaccine antigens. In: Christodoulides M, editor. Neisseria gonorrhoeae: methods and protocols. Methods in molecular biology 1997. New York: Springer/Humana Press; 2019. 2019/05/24ed: pp 121–142.
- Uronen H, Williams AJ, Dixon G, et al. Gram-negative bacteria induce proinflammatory cytokine production by monocytes in the absence of lipopolysaccharide (LPS). Clin Exp Immunol. 2000;122(3):312–315.
- Fowler MI, Yin KYHW, Humphries HE, et al. Comparison of the inflammatory responses of human meningeal cells following challenge with Neisseria lactamica and with Neisseria meningitidis. Infect Immun. 2006;74(11):6467–6478.
- Vaneechoutte M. The human vaginal microbial community. Res Microbiol. 2017 Nov-Dec;168(9–10):811–825.
- Betts JW, Hornsey M, Wareham DW, et al. In vitro and In vivo Activity of Theaflavin-Epicatechin combinations versus Multidrug-Resistant Acinetobacter baumannii. Infect Dis Ther. 2017 Sep;6(3):435–442.
- Betts J, Nagel C, Schatzschneider U, et al. Antimicrobial activity of carbon monoxide-releasing molecule [Mn(CO)3(tpa-kappa3N)]Br versus multidrug-resistant isolates of avian pathogenic Escherichia coli and its synergy with colistin. PLoS One. 2017;12(10):e0186359.
- Guntzel P, Nagel C, Weigelt J, et al. Biological activity of manganese(i) tricarbonyl complexes on multidrug-resistant Gram-negative bacteria: from functional studies to in vivo activity in Galleria mellonella. Metallomics. 2019 Dec 11;11(12):2033–2042.
- Dolange V, Churchward CP, Christodoulides M, et al. The Growing Threat of Gonococcal Blindness. Antibiotics (Basel). 2018 Jul 12;7(3):59.
- Strateva T, Mitov I. Contribution of an arsenal of virulence factors to pathogenesis of Pseudomonas aeruginosa infections. Ann Microbiol. 2011;61(4):717–732. 2011/12/01.
- Sengupta R, Altermann E, Anderson RC, et al. The role of cell surface architecture of Lactobacilli in host-microbe interactions in the gastrointestinal tract. Mediators Inflamm. 2013; Article ID 237921. 2013/03/13.
- Spurbeck RR, Arvidson CG. Inhibition of Neisseria gonorrhoeae epithelial cell interactions by vaginal Lactobacillus species. Infect Immun. 2008 Jul;76(7):3124–3130.
- Spurbeck RR, Arvidson CG. Lactobacillus jensenii surface-associated proteins inhibit Neisseria gonorrhoeae adherence to epithelial cells. Infect Immun. 2010 Jul;78(7):3103–3111.
- Vielfort K, Sjolinder H, Roos S, et al. Adherence of clinically isolated lactobacilli to human cervical cells in competition with Neisseria gonorrhoeae. Microbes Infect. 2008 Oct;10(12–13):1325–1334.
- Foschi C, Salvo M, Cevenini R, et al. Vaginal lactobacilli reduce Neisseria gonorrhoeae viability through multiple strategies: an in vitro study. Front Cell Infect Microbiol. 2017;7:502.
- Zheng HY, Alcorn TM, Cohen MS. Effects of H2O2-producing lactobacilli on Neisseria gonorrhoeae growth and catalase activity. J Infect Dis. 1994 Nov;170(5):1209–1215.
- Graver MA, Wade JJ. The role of acidification in the inhibition of Neisseria gonorrhoeae by vaginal lactobacilli during anaerobic growth. Ann Clin Microbiol Antimicrob. 2011 Feb;17(10):8.
- Breshears LM, Edwards VL, Ravel J, et al. Lactobacillus crispatus inhibits growth of Gardnerella vaginalis and Neisseria gonorrhoeae on a porcine vaginal mucosa model. BMC Microbiol. 2015 Dec;9(15):276.
- Fallon JP, Troy N, Kavanagh K. Pre-exposure of Galleria mellonella larvae to different doses of Aspergillus fumigatus conidia causes differential activation of cellular and humoral immune responses. Virulence. 2011 Sep-Oct;2(5):413–421.
- Kohler GA, Assefa S, Reid G. Probiotic interference of Lactobacillus rhamnosus GR-1 and Lactobacillus reuteri RC-14 with the opportunistic fungal pathogen Candida albicans. Infect Dis Obstet Gynecol. 2012;2012:636474.
- Vilela SF, Barbosa JO, Rossoni RD, et al. Lactobacillus acidophilus ATCC 4356 inhibits biofilm formation by C. albicans and attenuates the experimental candidiasis in Galleria mellonella. Virulence. 2015;6(1):29–39.
- Rossoni RD, Fuchs BB, De Barros PP, et al. Lactobacillus paracasei modulates the immune system of Galleria mellonella and protects against Candida albicans infection. PLoS One. 2017;12(3):e0173332.
- Huang XW, Xu MN, Zheng HX, et al. Pre-exposure to Candida glabrata protects Galleria mellonella against subsequent lethal fungal infections. Virulence. 2020;11(1): 1674–1684
- Krachler AM, Sirisaengtaksin N, Monteith P, et al. Defective phagocyte association during infection of Galleria mellonella with Yersinia pseudotuberculosis is detrimental to both insect host and microbe. Virulence. 2021 Dec;12(1):638–653.
- Luiz de Freitas L, Pereira da Silva F, Km F, et al. The virulence of Salmonella Enteritidis in Galleria mellonella is improved by N-dodecanoyl-homoserine lactone. Microb Pathog. 2021 Jan;11(152):104730.
- Kovacs-Simon A, Metters G, Norville I, et al. Coxiella burnetii replicates in Galleria mellonella hemocytes and transcriptome mapping reveals in vivo regulated genes. Virulence. 2020 Dec;11(1):1268–1278.
- Thomaz L, Gustavo de Almeida L, Fro S, et al. In vivo activity of silver nanoparticles against Pseudomonas aeruginosa Infection in Galleria mellonella. Front Microbiol. 2020;11:582107.
- Sadarangani M, Pollard AJ, Gray-Owen SD. Opa proteins and CEACAMs: pathways of immune engagement for pathogenic Neisseria. FEMS Microbiol Rev. 2011;35(3):498–514. 5/2011.
- Chen T, Belland RJ, Wilson J, et al. Adherence of pilus− Opa+ gonococci to epithelial cells in vitro involves heparan sulfate. J Exp Med. 1995;1995(182):511–517.
- Princivalle M, de Agostini A. Developmental roles of heparan sulfate proteoglycans: a comparative review in Drosophila, mouse and human. Int J Dev Biol. 2002 May;46(3):267–278.
- Estes Z, Faust R. Studies on the mucopolysaccharides of the greater wax moth, Galleria mellonella (Linnaeus). Comp Biochem Physiol. 1964;13(4):443–452.
- Melly MA, McGee ZA, Rosenthal RS. Ability of Monomeric Peptidoglycan Fragments from Neisseria gonorrhoeae to Damage Human Fallopian-Tube Mucosa. J Infect Dis. 1984;149(3):378–386.
- Cloud KA, Dillard JP. A lytic transglycosylase of Neisseria gonorrhoeae is involved in peptidoglycan-derived cytotoxin production. Infect Immun. 2002 Jun;70(6):2752–2757.
- Almonacid-Mendoza HL, Humbert MV, Dijokaite A, et al. Structure of the recombinant Neisseria gonorrhoeae adhesin complex protein (rNg-ACP) and generation of murine antibodies with bactericidal activity against gonococci. mSphere. 2018 Oct 10;3(5):5.
- Ward ME, Watt PJ, Glyn AA. Gonococci in urethral exudates possess a virulence factor lost on subculture. Nature. 1970;227(5256):382–384.
- Lambden PR, Heckels JE. Outer membrane protein composition and colonial morphology of Neisseria gonorrhoeae strain P9. FEMS Micro Lett. 1979;5(4):263–265.
- McGuinness BT, Clarke IN, Lambden PR, et al. Point mutation in meningococcal porA gene associated with increased endemic disease. Lancet. 1991;337(8740):514–517.
- Pandey AK, Cleary DW, Laver JR, et al. Neisseria lactamica Y92–1009 complete genome sequence. Stand Genomic Sci. 2017;12(1):41.