ABSTRACT
Fasciolosis caused by the liver flukes Fasciola hepatica and Fasciola gigantica is one of the most important neglected parasitic diseases of humans and animals. The ability of the parasites to infect and multiply in their intermediate snail hosts, and their adaptation to a wide variety of mammalian definitive hosts contribute to their high transmissibility and distribution. Within the mammalian host, the trauma caused by the immature flukes burrowing through the liver parenchyma is associated with most of the pathogenesis. Similarly, the feeding activity and the physical presence of large flukes in the bile ducts can lead to anemia, inflammation, obstruction and cholangitis. The high frequency of non-synonymous polymorphisms found in Fasciola spp. genes allows for adaptation and invasion of a broad range of hosts. This is also facilitated by parasite’s excretory-secretory (ES) molecules that mediate physiological changes that allows their establishment within the host. ES contains cathepsin peptidases that aid parasite invasion by degrading collagen and fibronectin. In the bile ducts, cathepsin-L is critical to hemoglobin digestion during feeding activities. Other molecules (peroxiredoxin, cathepsin-L and Kunitz-type inhibitor) stimulate a strong immune response polarized toward a Treg/Th2 phenotype that favors fluke’s survival. Helminth defense molecule, fatty acid binding proteins, Fasciola-specific glycans and miRNAs modulate host pro-inflammatory responses, while antioxidant scavenger enzymes work in an orchestrated way to deter host oxidant-mediated damage. Combining these strategies Fasciola spp. survive for decades within their mammalian host, where they reproduce and spread to become one of the most widespread zoonotic worm parasites in the world.
ABSTRACT
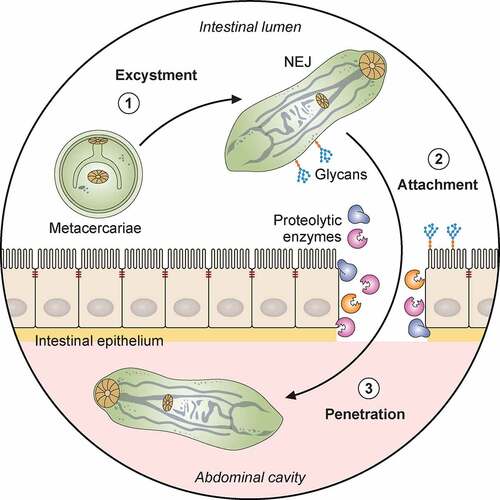
Introduction
Fasciolosis is a highly pathogenic parasitic disease of humans and their livestock caused by flatworms of the genus Fasciola, also known as liver flukes. Infection caused by the liver fluke species, Fasciola hepatica and Fasciola gigantica, are amongst the most neglected zoonotic diseases, despite their global distribution [Citation1–3]. These parasites are found on all inhabited continents, in more than 70 countries. F. hepatica is predominately found in temperate climates, but is also prevalent in the tropical and subtropical countries, including those in the Middle East (Egypt and Iran), South America (Bolivia, Ecuador and Peru) and Asia. F. gigantica, the cause of tropical fasciolosis, is primarily found in less-developed regions throughout Asia, Africa and the Middle East [Citation1,Citation4].
Liver flukes are extremely successful parasites and infections have been documented in humans and a range of ruminants, including sheep, cattle, goats, buffalo, camelids and cervids. Less commonly, these parasites infect non-ruminant herbivores (e.g., equids, lagomorphs, macropods, and rodents) [Citation5]. In livestock animals, Fasciola spp. infection causes significant morbidity and mortality, and is linked to reduced productivity and fertility and increased susceptibility to co-infections. Together, these contribute to annual economic losses in the order of €2.5 billion worldwide [Citation6–8].
The socio-economic and medical importance of fasciolosis is unquestionable. It is estimated that between 2.6 million and 17 million people are infected with Fasciola spp. globally [Citation9,Citation10]. Whilst human cases of F. gigantica infection are less common, they have been reported in tropical regions of Asia, Africa, Iran, and Hawaii [Citation5]. However, despite liver flukes being one of the most pathogenic human-infecting trematodes in terms of parasite-associated morbidity, a lack of epidemiological studies and case notification make it difficult to calculate the exact current burden of human fasciolosis. The most recent data, from 2012, estimated the disability-adjusted life-years (DALYs) for this infection at 35,000 per year [Citation10].
Genomic and transcriptomic studies, aligned with functional characterization of somatic and secreted molecules, have allowed a better understanding of Fasciola spp. parasite biology, and its relationship with their hosts [Citation11–15]. It is now clear that the different developmental stages of F. hepatica express and secrete a set of regulatory proteins, glycans and micro-RNAs (miRNA) that interact with host factors and tissues, mediating physiological changes that favor the establishment of the parasite. The high frequency of non-synonymous polymorphisms found in genes expressed by the flukes has been linked to the ability of Fasciola spp. to adapt to such a broad range of definitive hosts [Citation12]. Interestingly, many genes have expanded and diverged to create multi-membered families with very specific, although sometimes overlapping, functions that may also allow the parasite to adapt to different hosts and infection sites during its lifecycle [Citation3,Citation13,Citation14]. Of note, various parasite molecules also display key immunomodulatory properties and often function in conjunction to subvert the host immune responses in favor of the liver fluke parasite [Citation16–22]. Many of these molecules are being exploited to improve diagnostics, and to develop vaccine and drugs to control fasciolosis.
Here we review the main mechanisms of virulence and pathogenicity of Fasciola spp. parasites. We discuss how surface and excreted-secreted (ES) molecules contribute to infection and establishment within mammalian hosts, in spite of their induction of elaborate immune responses. Specific pathogenesis caused by each lifecycle stage of the liver fluke within the definitive hosts are highlighted (), as well as virulence aspects of different isolates and species.
Figure 1. Pathogenicity of F. hepatica within the mammalian host. 1: The metacercariae of F. hepatica become activated by a series of stimuli (CO2, temperature, bile salts, reducing conditions, pH) as they pass through the digestive system of a mammalian host. 2: The metacercariae excyst in the small intestine releasing NEJs that attach to the gut wall via surface glycans and penetrate through the intestinal epithelia with the aid of secreted cathepsin peptidases. 3: Once in the abdominal cavity, and throughout its lifecycle, F. hepatica expresses a plethora of virulence factors that enable the parasite to evade and modulate the host’s immune response. Many of these factors (Cathepsins; Fatty acid binding proteins, FABP; Helminth defense molecule, FhHDM; Extracellular vesicles, EVs) hamper the activation of host immune cells by limiting their ability to respond to inflammatory stimuli and subsequent capacity to promote antigen specific Th1/Th17- responses that are required to effectively clear infection. 4: In contrast, secreted proteins (Peroxiredoxin, FhPrx; parasite glycoproteins), as well as glycoconjugates on the tegmental surface of the parasite, actively recruit and modulate dendritic cells and M2 macrophages which favor the induction of Th2/regulatory immune responses, creating an immunological environment that benefits the parasites survival. 5: Over a period of days, the NEJs migrate through the abdominal cavity to the liver, where they begin tunneling a path through the connective tissue of the parenchyma, facilitated by parasite secreted cathepsin peptidases (FhCL2, FhCL3) capable of degrading the liver extracellular matrix. 6 and 7: The extensive tissue damage caused by the migration through the liver initiates a wound healing response characterized by the influx of immune cells, and subsequent induction of fibrosis to repair the damage. 8: Flukes reach the biliary ducts of the mammalian host approximately 12 weeks after infection. 9: Blood is a vital nutrient source for the mature parasites in the bile ducts, and they express several proteins related to red blood cell lysis, hemoglobin digestion and metabolism (Saposins, Cathepsins, Aminopeptidases). 10: The acquisition of these extra nutrients from blood allows Fasciola to produce thousands of eggs which are shed via the host’s feces that results in the infection of the intermediary snail host, restarting the parasite lifecycle. Adapted from different templates available in BioRender.com (2021). Retrieved from https://app.biorender.com/biorender-templates
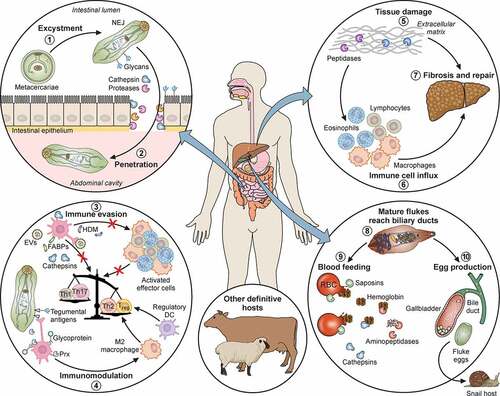
Fasciola spp. lifecycle and biology
As with most trematodes, F. hepatica and F. gigantica have a complex lifecycle, requiring a vertebrate primary host, in which the liver flukes reproduce sexually, and an intermediate host (aquatic snails in the family Lymnaeidae), in which asexual reproduction occurs [Citation1,Citation23]. Adults of the two Fasciola species differ in size and have distinct morphological characteristics. Both are large leaf-shaped worms: F. hepatica adults are ~4 cm in length and ~1.5 cm wide, while F. gigantica are ~7.5 cm in length and ~1.5 cm wide [Citation24]. These reside in the biliary ducts and gall bladder of the primary host, where they reproduce. Fasciola spp. are hermaphroditic, and therefore capable of self-fertilization; however, cross-fertilization between two adult flukes is the most common form of reproduction and contributes to the gene polymorphism observed within these species [Citation12]. The flukes can live for decades within the host and produce up to 25,000 eggs per day per fluke [Citation25]. These eggs are released into the intestine and are passed into the surrounding environment within the feces.
The eggs released into the environment are initially immature but following a period of embryonation develop into a miracidium, a ciliated larva that hatches out of the egg through an opening termed the operculum, and actively seeks and infects a suitable snail intermediate host. The miracidium lifespan (8 to 24 hr) is greatly limited by their glycogen stores, which is their primary energy source. To increase the chances of locating and invading a suitable host snail within this period, the larvae have developed refined chemo-sensorial mechanisms that involve positive phototropism and expression of genes involved in the secretion of pheromones and tissue-degrading metallopeptidases [Citation3,Citation26].
Inside the snail, the parasite undergoes asexual development, going through various stages in sequence, sporocyst, rediae and finally cercariae. Remarkably, during this process, known as clonal expansion, a single miracidium can produce 10 to 700 cercariae [Citation26]. The large number of cercariae emerging from the snail ensures that the lifecycle will progress. The ability of the parasite to survive and reproduce within the snail has been linked to the expression of genes involved in the manipulation of snail innate immune responses. Specifically, transcriptome analysis of the intra-snail stages of F. gigantica revealed an up-regulation of genes responsible for innate immune responses, B cell receptor signaling pathways and lymphocyte activation. In addition, increased expression of cathepsin L peptidases has been shown to be associated with their migration and feeding [Citation13,Citation23].
Appropriate temperature and light conditions stimulate the snails to shed cercariae. These large (~250 µm) and motile larvae swim in the water and encyst either on leafy vegetables or at the water surface to form the resistant metacercariae, which is the infective stage for the definitive mammalian host. Encystment occurs in response to changes of environmental conditions (e.g., oxidative stress, UV light, salinity and CO2 concentration) that are sensed by the cercariae. Specific proteins expressed on the tegumental surface of cercariae (e.g., aquaporins) have been linked to their ability to detect some of these changes [Citation13].
Fasciola spp. infection only occurs when the mammalian host ingest vegetation or water contaminated with metacercariae [Citation1]. In the small intestine, the metacercariae excyst releasing the infectious newly excysted juveniles (NEJs). The NEJs are small (~0.1 mm), active parasites that penetrate the gut wall and can be found in the abdominal cavity 6 to 72 h post infection, depending on the host species [Citation27,Citation28]. This process is fundamental for fasciolosis infection to ensue and marks the beginning of the disease pathology.
Although far smaller and sexually immature, the NEJs already possess most of the structural features of an adult fluke. The outer surface of the juvenile flukes is called the tegument, and its primary function is to protect the fluke from host enzymes and immune attack [Citation29]. It consists of a syncytial layer surrounding the surface of the parasite, and is contained by a plasma membrane covered with a thick carbohydrate coat or glycocalyx. The tegument is a highly functional, metabolically active structure, responsible for absorption of nutrients, synthesis and secretion of substances, osmoregulation, protection, and production of extracellular vesicles (EVs) [Citation19,Citation30]. It has a sensorial role, due to the presence of small spines on the surface, which are also likely to be involved in locomotion [Citation31,Citation32].
The tegument also plays a key role during fluke infection as it actively suppresses the immune response of the mammalian host, allowing the juveniles to develop into adult flukes and continue the lifecycle. Early studies have demonstrated that the tegument is very dynamic, being continuously sloughed off and replaced as the parasite migrates through different host tissues [Citation31,Citation33]. Initially, the NEJ tegumental syncytial layer is dominated by structures referred to as T0 secretory bodies, which change into T1 bodies as the parasite enters the liver parenchyma, and finally to T2 bodies within the fully mature adult parasite [Citation31]. This change of secretory body type is directly associated with the tegument composition and microenvironment the parasite is in, and is considered an immune evasion strategy as the contents of these bodies are released at the apical membrane and added to the glycocalyx [Citation34].
Within one week of ingestion, the parasite crosses the peritoneum and reaches the liver parenchyma by penetrating the Glisson’s capsule. While the juvenile fluke moves through the liver, it grows significantly by feeding on host tissue cells and, eventually, on blood [Citation32,Citation35]. These activities cause most of the clinical symptoms associated with acute fasciolosis. At this stage, the parasite is ~5 mm and mechanical damage arises from abrasion by the parasite tegument, the digestion of the tissue and the action of the suckers as the parasite moves within the parenchyma. These physical actions result in lesions marked with hemorrhagic migratory tracts that are commonly observed in the liver parenchyma. The NEJs quickly manipulate the host’s immune response, preventing the onset of Th1-mediated immune responses by modulating protective innate cells, such as macrophages, and establish a Th2-type of immune response that benefits their survival. After 3 to 4 months, the parasites reach the bile ducts where they develop into sexually mature adults and initiate egg production [Citation36].
Adult parasites possess a tough outer tegument surface, with the highly specialized functions discussed above. The spines of the tegument are longer at this stage and help to maintain the position of the fluke within the tissues. The mechanical interactions between hepatic cells and the parasite tegument cause sufficient trauma leading to cell destruction [Citation37]. The spines also facilitate feeding as the parasite uses them for puncturing small blood vessels [Citation27]. Fasciola spp. have two suckers, the oral sucker, located at the anterior end surrounding the mouth, and the ventral sucker, both of which cause major tissue damage as the flukes use them to feed, attach to the bile duct walls and to migrate [Citation29,Citation38,Citation39]. Through the oral sucker, food enters the parasite’s bifurcated, blind-ending gut, where it is digested. Nutrients are absorbed through the epithelial layer, or gastrodermis, lining the parasite gut, while the excess undigested material is regurgitated [Citation40] (). Therefore, this material, together with a number of excreted-secreted components, is released into the host via the parasite’s mouth [Citation41]. The parasite also secretes many molecules through its tegument, both directly into the microenvironment and packaged into EVs [Citation42,Citation43]. These molecules act at the parasite-host interface, helping the parasite to survive by manipulating the host environment.
Figure 2. Life stages of F. hepatica and F. gigantica. A: Liver from an infected sheep with acute F. hepatica infection (reported by López Corrales et al. [Citation157]) showing gross pathology. The white tracks delineate the tunneling activity of the parasites through the liver tissues, most commonly observed on the left lobe of liver closest to the intestine in situ (LL). GB: Gall bladder. Scale bar, 1 cm. B: Microscopical liver pathology shown by hematoxylin and eosin (HE) stained serial liver sections from a mouse infected with F. hepatica (Molina-Hernandez and Dalton, unpublished). Top panel: Liver section displaying the migratory tracts (white arrows) formed by the invading F. hepatica immature flukes (black arrow). Bottom panel: The damage caused by the migrating parasite (black arrow) is resolved with no visible tracts in the liver and acute necrotic foci (ne) comprised of inflammatory cells (mainly eosinophils and macrophages). Scale bar, 200 µM. C: Adult F. gigantica (Fg) and F. hepatica (Fh) parasites. Note the typical leaf shape morphology of each species and the size variation. The length-to-width ratio of adult F. gigantica is greater than that of F. hepatica parasites. F. hepatica parasites have a broader anterior end, with defined shoulders, whilst F. gigantica is narrower and lacks this definition. Scale bar, 1 cm. D: The differential morphology of eggs from F. hepatica (white arrows) and F. gigantica (black arrows). The eggs of F. gigantica are typically larger, but variation exists in different definitive hosts and thus a considerable overlap is observed. Scale bar, 100 µM. E: The invasive newly excysted juvenile stage of F. hepatica has a typical cephalic cone shape. Antibodies to the digestive cathepsin peptidase L3 were used to probe the parasite and highlights their bifurcated gut (g) represented by the green fluorescence. The musculature of the parasite is highlighted by the red fluorescence, that accentuates the oral sucker (OS), ventral sucker (VS) and tegument of the parasite. Scale bar, 20 µM
![Figure 2. Life stages of F. hepatica and F. gigantica. A: Liver from an infected sheep with acute F. hepatica infection (reported by López Corrales et al. [Citation157]) showing gross pathology. The white tracks delineate the tunneling activity of the parasites through the liver tissues, most commonly observed on the left lobe of liver closest to the intestine in situ (LL). GB: Gall bladder. Scale bar, 1 cm. B: Microscopical liver pathology shown by hematoxylin and eosin (HE) stained serial liver sections from a mouse infected with F. hepatica (Molina-Hernandez and Dalton, unpublished). Top panel: Liver section displaying the migratory tracts (white arrows) formed by the invading F. hepatica immature flukes (black arrow). Bottom panel: The damage caused by the migrating parasite (black arrow) is resolved with no visible tracts in the liver and acute necrotic foci (ne) comprised of inflammatory cells (mainly eosinophils and macrophages). Scale bar, 200 µM. C: Adult F. gigantica (Fg) and F. hepatica (Fh) parasites. Note the typical leaf shape morphology of each species and the size variation. The length-to-width ratio of adult F. gigantica is greater than that of F. hepatica parasites. F. hepatica parasites have a broader anterior end, with defined shoulders, whilst F. gigantica is narrower and lacks this definition. Scale bar, 1 cm. D: The differential morphology of eggs from F. hepatica (white arrows) and F. gigantica (black arrows). The eggs of F. gigantica are typically larger, but variation exists in different definitive hosts and thus a considerable overlap is observed. Scale bar, 100 µM. E: The invasive newly excysted juvenile stage of F. hepatica has a typical cephalic cone shape. Antibodies to the digestive cathepsin peptidase L3 were used to probe the parasite and highlights their bifurcated gut (g) represented by the green fluorescence. The musculature of the parasite is highlighted by the red fluorescence, that accentuates the oral sucker (OS), ventral sucker (VS) and tegument of the parasite. Scale bar, 20 µM](/cms/asset/1d730547-b162-4d52-9354-0004ef1a7d9e/kvir_a_1996520_f0002_oc.jpg)
A newly laid ovoid egg contains an immature miracidia, and is relatively large (130–150 μm by 63–90 μm), operculated, and yellowish brown in color. The eggs are passed into the bile and might remain in the gall bladder for a certain period. Eventually, they reach the host intestine with the bile during digestion, and from there are excreted with the feces into the environment. In fact, the presence of eggs in feces is one of the most commonly employed tools used for diagnosing patent fasciolosis and the total egg count can give a general indication of the parasite burden [Citation44].
F. hepatica isolates and variation in virulence
Genetic analyses of F. hepatica isolates throughout the world have shown that these parasites display high levels of genetic heterogeneity, which has been linked to their wide host range and capacity for rapid adaptation to the host environment and external selection pressures, such as drug interventions [Citation12,Citation45,Citation46]. These genetic differences may also play a role in the parasite virulence. In our laboratory, studies of F. hepatica infection have shown that the proportion of parasites that survive to adult stages can vary depending on the isolate. To date, the majority of studies investigating the phenotypic differences between liver fluke isolates are linked to studies of drug resistance, namely triclabendazole (TCBZ) resistance, that is the most commonly used flukicidal treatment of F. hepatica in both humans and livestock [Citation47]. The F. hepatica isolates most frequently used for these studies are isolates of known TCBZ susceptibility/resistance that have been maintained experimentally for over 20 years [Citation48], including the Sligo (Ireland), Oberon (Australia), Dutch (Netherlands), Cajamarca (Peru) and Rubino isolates (Uruguay) that are considered resistant to TCBZ, while the Cullompton (UK), Fairhurst (UK), Sunny Corner (Australia), and Centro de Diagnóstico e Investigaciones Veterinarias (CEDIVE) isolates (Argentina) are susceptible to TCBZ [Citation49–51].
Analysis of these isolates has shown that they display different phenotypic traits that may influence the virulence and pathogenicity of these parasites. The study by McConville et al. [Citation52] compared the Sligo and Cullompton isolates in sheep, which showed that the resistant isolate from Sligo reached the bile ducts one week earlier compared to the susceptible Cullompton isolate, and also produced eggs two weeks earlier. However, the Sligo isolate flukes were smaller in size, produced fewer eggs and their metacercariae were less infective to sheep when compared to the Cullompton isolate [Citation52]. Similarly, Walker et al. [Citation53] examined differences in cercarial production by the Fairhurst and Oberon isolates and their infectivity in rats. The Oberon isolate demonstrated accelerated egg hatching and production of cercariae, as well as yielding four times the number of cercariae. The resulting metacercariae were also more infectious and displayed an accelerated developmental progression, which was two and half weeks faster when compared to the Fairhurst isolate [Citation49,Citation53]. However, these results must be interpreted with caution as after years of laboratory maintenance these isolates may no longer be representative of field isolates; in fact they display traits such as abnormal spermatogenesis (Sligo) [Citation54] or aspermia, polyploidy (Cullompton) [Citation55] and reduced genetic heterogeneity (Fairhurst) [Citation56] that may affect their virulence and pathogenicity.
Most recently, the study by Hodgkinson et al. [Citation57] highlighted the phenotypic intra- and inter-variation between triclabendazole-susceptible and -resistant clones that were recently propagated from the field. The adult parasites from the TCBZ susceptible isolates (80–280 mg) were larger than their resistant counterparts (20–160 mg). However, despite the parasites being derived from a single miracidium (and therefore considered a clonal line) variation in the size of the parasites obtained from their mammalian host was observed, indicating that the host interactions also play a role in parasite growth and survival. Analysis of the snail-associated stages revealed no demonstrable differences between the isolates in the timing of cercarial shedding or the number of cercariae recovered.
The snail intermediate hosts of liver fluke parasites
F. hepatica utilizes snails of the Lymnaeidae family as intermediate hosts, with the favorable species varying between continents and climates. Within Europe, Galba truncatula is the predominant intermediate host used by F. hepatica [Citation1], likely due to the similar adaptation of both species to require a wet, temperate climate [Citation58]. G. truncatula is found throughout Africa, North and South America, and Asia, though other species are also commonly identified as host species in these continents () [Citation59–61]. In fact the ability of F. hepatica to infect a wide range of lymnaeids that, according the study by Correa et al. [Citation62], are distributed into three main clades (C1 (American species), C2 (Eurasian species) and C3 (Australasian species)) is possibly a determinant for the broader geographic distribution of this species, compared to F. gigantica, which have been particularly associated to species in the C3 group.
Table 1. Major Species of snails from the Family Lymnaeidae infected with F. hepatica globally
A study into the host specificity of F. hepatica miracidia identified miracidia-attracting glycoproteins (MAGs) in snail-conditioned water of G. truncatula, but not L. stagnalis, which play a role in stimulating host-finding responses in the miracidia [Citation64]. Following infection of the snail host, the miracidia undergo the asexual, or clonal expansion, phase of their parasitic lifecycle [Citation65]. When environmental conditions are optimal, the development of F. hepatica miracidia to cercariae takes approximately 5 to 7 weeks [Citation66]. The efficiency of the clonal expansion is affected by environmental stresses encountered by the snail; for example, in snails that were exposed to 10 days of desiccation, the number of rediae ranged between 18–25, compared to 43 in the unstressed control [Citation67], suggesting that dry conditions impact the ability of the snails to act as an intermediate host, as well as making conditions challenging for miracidia to survive in the environment. On the other hand, infection with F. hepatica has also been shown to have a negative effect on the lifespan and reproductive activity of the snail host, which may play a role in the subsequent prevalence of fasciolosis on pasture [Citation68].
The species of snail host may also impact the number of metacercariae in the environment. An investigation into the suitability of G. truncatula and P. columnella for metacercarial production suggested that P. columnella is the more proficient intermediate host of the two, due to its greater survival rate 30 days post-infection, and ability to produce two-fold higher numbers of cercariae than G. truncatula [Citation69]. While multiple species of snails are able to act as the F. hepatica intermediate host, the host species may impact the lifecycle of F. hepatica, as the composition of ES products from NEJs can change depending on the intermediate snail host. A study comparing the secretome of NEJs from L. viatrix and P. columnella identified five proteins that were unique to NEJs that had used L. viatrix as the intermediate host, and thirty nine that were unique to P. columnella-derived NEJs [Citation65]. It is important to note that this study used miracidia from two different F. hepatica isolates, and therefore the differences in the NEJs secretome may not necessarily be solely attributed to the snail host species.
Unlike F. hepatica, the lifecycle of F. gigantica tends to occur in regions with defined wet and dry seasons. In areas where F. gigantica is most common, various Radix, Lymnaea and Galba snail species act as intermediate hosts, all of which rely on the presence of well-oxygenated, slow-moving water for survival [Citation62,Citation70,Citation71]. Even so, in regions of varying altitudes, where the environment supports the survival of several snail species permissive to infection with either species, such as in Pakistan and Tanzania, overlaps in the distribution of F. hepatica and F. gigantica occurs [Citation72,Citation73]. The snail species susceptible to infection with F. gigantica have varying capacities to adapt to dry seasons and may undergo aestivation in mud until suitable conditions return the following season [Citation70]. The susceptibility to drying affects the survival of parasite stages within the infected snails and, consequently, the availability of infective metacercariae in the environment. The development of F. gigantica cercariae in infected snails is impeded at temperatures below 16°C, which is higher than the 10°C limit typically observed with F. hepatica, and demonstrates an adaptation to warmer climatic conditions [Citation70,Citation74].
Snails infected with F. gigantica produce a higher proportion of floating metacercariae than those infected with F. hepatica (17–35% vs 4–7%, respectively) [Citation75,Citation76]. This finding has important implications for routes of transmission in both livestock and human populations, and suggests that contaminated water sources may play a more significant role in the transmission of F. gigantica than F. hepatica. Once in the environment, the survival of F. gigantica metacercariae is a function of humidity, temperature, pH, and exposure to direct sunlight. The metacercariae of F. gigantica are more tolerant to higher temperatures than F. hepatica and will remain viable between 2–35°C when adequate humidity is maintained [Citation77–79]. Exposure to direct sunlight results in 100% mortality of F. gigantica metacercariae within eight hours, compared to only two hours for F. hepatica [Citation80,Citation81]. Transcriptome analysis of F. gigantica cercariae have revealed a shift in gene expression toward decreased metabolic processes and nucleotide synthesis as they mature into recently encysted metacercariae, and suggests that their low metabolic rate is maintained by pH regulation and an avoidance of autolysis via an associated reduction in endopeptidase activity [Citation3]. Each of these factors play an important role in the maintenance of F. gigantica in the environment and influence the various routes of transmission to mammalian hosts.
Pathogenicity of Fasciola spp. in their definitive hosts
Livestock
Fasciola spp. are zoonotic parasites that infect primarily domestic livestock animals, most commonly sheep, goats, cattle and buffaloes. Infection manifests in three phases: acute, sub-clinical and chronic. In sheep, acute disease is often first detected by sudden death of up to 10% of the flock, usually due to high levels of blood loss from physical damage to the liver [Citation82]. Symptoms of acute infection in sheep can also include reluctance to run due to abdominal pain, lethargy and reduced appetite for grazing. Acute fasciolosis in sheep can be complicated by secondary infection of the liver by Clostridium noyvi, resulting in clostridial necrotic hepatitis [Citation83]. Sub-clinical disease is slightly more delayed than acute disease and presents as hemorrhagic anemia. Chronic fasciolosis in sheep presents as failure to thrive due to low body weight and poor quality fleece, and also severe swelling under the jaw known as bottle-jaw [Citation82]. While Bos taurus cattle usually build a degree of immunity to infection with F. hepatica infection, this is not observed in sheep [Citation83].
Studies in sheep have revealed the impact of F. hepatica on the serology profiles of infected animals. Significantly lower levels of total protein, albumin, glucose, triglyceride, cholesterol and high-, low- (LDL) and very low-density (VLDL) lipoproteins were detected in infected sheep compared to uninfected controls, while the activity of aspartate aminotransferase (AST), alanine aminotransferase (ALT), γ-glutamyl transferase (GGT) and lactate dehydrogenase (LDH) were significantly higher in infected animals [Citation84]. These differences were still detectable 28 days after drug treatment, but no significant differences between the control and treated groups were observed 56 days post-treatment [Citation84]. In another study, infected sheep had significantly higher levels of GGT, and total and direct bilirubin compared to uninfected controls [Citation85]. These changes in the serology profiles of infected animals are associated with liver damage that causes liver enzymes to leach into the blood, and with the establishment of adult flukes in the biliary ducts. While the cellular response of sheep is consistent regardless of the level of infection, the degree of eosinophilia increases in line with the level of infection [Citation86].
Acute disease is rarely seen in cattle, instead, the animals tend to develop chronic disease if they acquire a particularly heavy infection [Citation87]. However, cattle and buffaloes calves exposed to heavy infections may suffer from acute fasciolosis and death [Citation88]. In the event of extremely high fluke burdens, clinical disease may occur as a result of the extensive damage to the liver caused by migrating juvenile flukes [Citation66]. Liver fibrosis in infected cattle is far more severe than that in infected sheep [Citation89] and there is a positive correlation between the extent of liver fibrosis and the number of adult fluke recovered at necropsy [Citation90]. An assessment of cattle carcasses in Uruguay identified a positive correlation between F. hepatica infection and a reduction in carcass weight, with the degree of weight loss being more significant in younger animals aged less than 30 months [Citation91]. A similar study conducted in Brazil comparing the weight of cattle following F. hepatica infection showed that weight loss of up to 11% can occur in infected animals compared to uninfected animals [Citation92]. In agreement with these studies, infection with F. hepatica was shown to reduce weight gain by up to 10 g per day, and delay slaughter by up to 2 weeks, compared to uninfected animals [Citation93].
The clinical symptoms of fasciolosis in cattle generally include weight loss and diarrhea, while dairy cows may also present with reduced milk production and fertility [Citation89]. In high yielding dairy herds, milk production may be reduced by up to 15% [Citation94], representing a financial loss of approximately £300 per cow per annum [Citation95]. As well as a reduction in milk production, infected dairy herds show a significant average reduction in milk protein and fat content of 0.06 kg compared to uninfected herds [Citation96]. F. hepatica infection in dairy cattle is also associated with an increase of 4.69 days in the time between calving and conception [Citation97]. Calves born to infected cows may be weak and sickly due to receiving inadequate nutrition from their mothers [Citation89].
Similar to sheep, studies in cattle have identified the effects of F. hepatica on the serological profiles of infected cattle. Infected animals show significant increases in the liver enzymes AST, GGT and alkaline phosphatase (AP) compared to uninfected animals [Citation98], reflecting the damage to the liver caused by the flukes. A study in Argentina found that cattle infected with F. hepatica showed significant increases in leukocytes, eosinophils, GGT, gamma globulin and total protein in blood and serum samples compared to uninfected controls, which is indicative of cholestasis and liver inflammation, dysfunction and necrosis [Citation99].
Postmortem examinations of sheep and cattle carcasses can confirm liver fluke infection by the presence of lesions and tracks in the liver and eggs in the gall bladder. However, the pathology of fasciolosis could be greatly prevented by early diagnosis, which allows for appropriate anthelmintic treatment before the parasite reaches the liver and bile duct of the host. Unfortunately, most diagnostic methods available have drawbacks and are not ideal for detection of the immature stages. Fecal eggs counts (FEC) are only useful to detect patent infections and have poor sensitivity with low burden infections [Citation100]. Enzyme-linked immunosorbent assays (ELISAs) such as the coproantigen-ELISAs offer an alternative method of diagnosis from FEC, but they are limited in detecting infection inside the pre-patent period [Citation101,Citation102]. Of the methods mentioned in Table 2, only serological ELISAs were proven to detect specific anti-F. hepatica antibodies as early as ~3 weeks post-infection [Citation103,Citation104]; however, this method cannot distinguish between new and historic infections. Diagnosis can be complemented by considering nonspecific symptoms, namely increased liver enzymes in serum (i.e., ALT, AST, GGT, LDH and AP), anemia and decreased serum albumin levels. Elevation of specific hepatic enzymes in host circulation has been demonstrated to be synchronous with the pre-patent (AST and ALT) and patent (AP) phase of infection [Citation105]. Moreover, currently only DNA-based diagnostic methods can reliably differentiate between infections with F. hepatica and F. gigantica [Citation106].
Humans
Liver flukes have been infecting humans for over 5000 years [Citation115,Citation116], yet it was not until 1760 that the first case was described during the autopsy of a female in Germany [Citation117]. But even up to the beginning of the 1990s, fasciolosis was still not considered an important disease of humans. That changed in the early 1990s when Hillyer [Citation118], Bjorland and colleagues [Citation119] described a very high prevalence of fasciolosis amongst the native Aymaran population of the Bolivian Altiplano. The Altiplano corridor stretching from Bolivia, through Peru to Ecuador still represents the region of highest endemic fasciolosis in the world. Remarkably, since the introduction of F. hepatica from Europe, sometime during the last 450 years, the parasite has adapted well to the high altitudes of >13,000 ft and to the local intermediate hosts. Varying levels of prevalence, from 5.9% to 70%, are found sporadically throughout the region depending on the hydrology, geography, snail host distribution and levels of animal infection [Citation120,Citation121]. A greater focus on the emergence of human fasciolosis over the last 30 years has discovered major endemic regions in China, South-East Asia (such as Vietnam), Egypt, Turkey and Northern Iran [Citation10,Citation122–125], and that outbreaks or cases occur across 80 countries where animal fasciolosis is also present [Citation10,Citation126]. Consequently, fasciolosis has been recently recognized as an important neglected zoonotic disease of humans by the World Health Organization [Citation10]. The emerging importance of human fasciolosis has spurred the publication of several excellent detailed reviews over the last five years [Citation9,Citation46,Citation126].
Infection in humans is mainly acquired following ingestion of edible aquatic vegetables and plants, which vary depending on the region [Citation46,Citation121,Citation127,Citation128]. Contaminated vegetables may be sold in local markets that are distant from the source of parasites and snails such as that found in outbreaks in some towns in Northern Iran [Citation129,Citation130]. As many metacercariae float rather than adhere to vegetation the drinking of water carrying parasites or the consumption of vegetables washed in contaminated water can be another means of infection [Citation46,Citation131]. Sporadic cases in Europe and elsewhere are often associated with the eating of wild watercress foraged from the side of rivers [Citation132,Citation133]. Not surprisingly therefore, outbreaks tend to be local and familial [Citation134]. In highly endemic regions, such as Bolivia, Egypt and Vietnam, children are more in danger of the consequences of disease (anemia, liver damage, impaired cognitive development) and appear to be more susceptible to infection. While Parkinson et al. [Citation135] found no significant association between infection levels and sex in their studies in Bolivia, a survey of >21,000 children in Egypt found a higher prevalence in females as well as a greater number of eggs in their stool samples [Citation136].
The pathology of fasciolosis in humans depend on several variables, including fluke species and isolates, parasite burden and host biology (e.g., immune status, age, nutrition). The clinical manifestations of fasciolosis caused by F. hepatica and F. gigantica are considered the same, although the larger size of the latter may result in a greater chance of biliary obstruction [Citation137]. Disease pathology is mostly associated with the trauma caused by the immature flukes burrowing through the intestines and liver parenchyma and this correlates with the level of infection [Citation88]. Low level infections may be asymptomatic or include mild symptoms at the acute stages but can progress to a serious chronic inflammatory situation at a later stage [Citation36]. However, in general, acute infection is characterized by vigorous host immune responses directed to the invasive parasites and their antigens, and may result in fever, nausea, abdominal pain, hepatomegaly, weight loss, anemia, transitional eosinophilia and elevation of liver enzymes [Citation138,Citation139]. The migrating parasites damage tissues and blood vessels causing large subcapsular liver hematomas that can be life-threatening [Citation36,Citation140,Citation141]. These symptoms can last for 2 to 4 months but in endemic regions repeated infections result in overlapping of acute and chronic symptoms [Citation120,Citation142].
Although chronic infections are often asymptomatic, they may be associated with signs of biliary obstruction, abdominal pain and fatty food intolerance [Citation88]. These can take months or even years to manifest themselves. Over time adult parasites cause damage to the bile duct with their spines when they move along the biliary tree. They also secrete many antigens and puncture the bile duct walls to gain access to blood which ultimately leads to hyperplasia of the bile duct epithelium and chronic inflammation, and eventually cholangitis and cholecystitis [Citation121,Citation143]. The pathological signs of human fasciolosis are varied and are dependent on overall parasite dose [Citation1], but include fibrotic lesions and micro-abscesses, and necrotic tracts within the liver parenchyma surrounded by immune cells including eosinophils, consistent with that observed in sheep and goats [Citation144] (). Ectopic fasciolosis, whereby parasite migrate to tissues other than the liver (e.g., lungs, intestines, brain), has been described in humans but is not the norm [Citation145].
While diagnosis of human fasciolosis in endemic areas is relatively straight-forward because it is suspected and recurrent, in areas where it is not common difficulties arise in diagnosis because the complex development and migration of the parasites ensure a changing face of symptoms. Moreover, symptoms are generally nonspecific in nature and can be easily mistaken for other diseases, especially those of the liver, and range from mild to severe [Citation146,Citation147]. Patients usually present with a variety of indicators including fever, headache, fatigue, chills, sweats, abdominal pain, epigastric discomfort, rashes and may also suffer from anemia and weight loss [Citation142]. Clinical hallmarks includes elevated levels of liver enzymes (e.g. AST, AP in acute stages and GGT in chronic stages) and high peripheral eosinophilia [Citation9,Citation105,Citation148]. In the clinic, computerized topography (CT) imaging can identify hypodense liver nodules and lesions, branching tracks associated with parasite migration and bile duct enlargement in the chronic infection [Citation148–150]. Liver biopsy may show hepatitis, inflammation, acute necrosis and eosinophilia in portal and sinusoidal spaces, but is unlikely to detect parasites in tissue [Citation148]. Ultrasonography of the abdomen could detect intra-hepatic bile duct enlargement. In a recent study, live worms were visualized in the major duodenal papilla by endoscopic retrograde cholangiopancreatography and then extracted for identification [Citation151].
Serological examination, especially enzyme-linked immunosorbent assay ELISA, has proven an important adjunct for diagnosis, but these tests are not routinely available in the clinic. In the USA, the Center for Disease Control (CDC) recommends an immunoblot assay with Fasciola saposin antigens (FhSAP2) [Citation152], whereas in Europe ELISAs that exploit parasite-secretory antigens or cathepsin L peptidases (FhCLs) are used [Citation132,Citation153]. Following treatment with triclabendazole (Novartis, 10 mg/kg on day 1 and 2), which is effective against both migratory and bile duct parasites, symptoms and clinical signs generally resolve within 1 to 2 months, although a follow-up treatment may be required [Citation121,Citation154,Citation155]. The emergence of triclabendazole-resistant parasites in livestock globally is of concern for the treatment of human infection with at least one study indicating reduced treatment efficacy in an endemic area of Peru [Citation156].
Mechanisms of pathogenicity of Fasciola spp. parasites
Means of infection and excystment
The ability of Fasciola spp. cercariae to encyst on vegetation and in water allows for the contamination of both food and drink, and increases the chances of infection of both animals and people. Moreover, encysted metacercariae are hardy and can survive for long periods in the environment, which contributes to the parasite survival and virulence [Citation3,Citation158]. Indeed, cysts were observed to remain infective after being dried or exposed to 1% corrosive sublimate solution for 24 hr or to 50% alcohol for 2 hr [Citation159], which is mainly due to the resistant walls that enclose the metacercariae. The four layers that form the cyst are composed of tanned protein, mucoprotein, acid mucopolysaccharide, and keratinized protein embedded in a matrix consisting of protein and lipid, which together provide structural rigidity and protection against desiccation, toxic substances and attack by bacteria and fungi [Citation159]. Furthermore, contrary to earlier assumptions that the metacercariae are dormant, transcriptional analysis revealed that these stages are metabolically active, transcribing genes involved in the regulation of redox metabolism (FhPrx; superoxide dismutase, FhSOD; and, FABP), pH and endopeptidase activity (cathepsin L and B peptidases, and legumain) [Citation3]. This significant metabolic activity of metacercariae, however, is associated with their reduced infectivity of older cysts and limited longevity [Citation158,Citation160,Citation161].
Infectivity of the metacercariae is influenced by a number of factors including the definitive host, parasite isolate, climatic conditions, seasonality, snail host species and larval stage of development in the snail [Citation49,Citation69,Citation158,Citation161]. As the metacercariae are the infective stage of Fasciola spp., the progression of the disease is associated with the dose of metacercariae ingested, the isolate and the host species. Nonetheless, in general, after ingestion of the metacercariae by the mammalian host, excystment occurs within a few hours and the NEJs immediately begin boring through the wall of the host intestine.
The excystation process is complex. In the stomach, host acid peptidases remove the outer cyst layer, initiating the active emergence phase. Activation of the larvae within the inner cyst occurs in the stomach, and is stimulated by high CO2 conditions and a temperature of approximately 39°C. Within the duodenum, escape of the NEJs from the metacercarial cyst is prompted by bile salts and reducing conditions () [Citation3,Citation158]. Genes involved in the expression of cell adhesion molecules such as intergrins and cadherins, as well as cytoskeletal proteins such as talins, are up-regulated in the metacercariae relative to the other lifecycle stages. Although the role of these molecules during this stage of infection is yet to be characterized, it is possible that they enable the metacercariae to sense the environmental changes necessary to start the excystment process [Citation12].
Recently, Cwiklinski et al. [Citation13] reported the up-regulation of two genes associated with the response to lipopolysaccharide (LPS) in the metacercariae stage. Considering the environments the metacercariae must endure (pasture and then the host gut), these proteins could have important roles in protecting the cyst. Moreover, although not yet characterized, it is possible that these proteins function to stop bacteria entering the host blood stream as the NEJs burrow through the host intestinal wall, hence preventing local pro-inflammatory responses that could damage the larvae and block infection [Citation13].
Invasion and migration in the mammalian host
Post-excystment, the parasites enter a new phase of their infective cycle, migrating through the tissues of a mammalian host. The NEJs must transverse the wall of the small intestine rapidly, as their viability decreases significantly while they remain in the gut. They rapidly burrow through the gut wall and have been observed in the abdominal cavity of multiple experimentally infected hosts’ hours after ingestion [Citation162,Citation163]. There is a marked change in the parasite’s metabolism as it migrates through the host, from aerobic energy metabolism to anaerobic metabolism, highlighted by changes in the expression of enzymes related to these pathways at different stages of infection [Citation13,Citation164]. Concomitantly, to penetrate through the intestinal tissues, NEJs secrete a range of stage specific peptidases and proteolytic-related proteins, key virulence-associated factors required to breakdown components of the extracellular matrixes (ECM) that hold tissues together ().
Figure 3. The profile of proteins secreted by the three main life cycle stages migrating through the host. The proteomic data for the newly excysted juveniles 24 h post-excystment (NEJ 24), the immature fluke 21 days post-infection (Immature) and the adult parasites (Adult) is extrapolated from Cwiklinski et al. [Citation13], Cwiklinski et al. [Citation197] and Murphy et al. [Citation183], respectively. The protein abundance across the three stages, represented by the emPAI values detailed, is highlighted from low to high abundance by the yellow to green color scale, respectively. Proteins that are secreted in multiple isoforms, such as the cathepsin L peptidases, have been grouped together. The 12 uncharacterized proteins are shown as one group. *Abbreviated name for 4-methyl-5(B-hydroxyethyl)-thiazole monophosphate biosynthesis enzyme
![Figure 3. The profile of proteins secreted by the three main life cycle stages migrating through the host. The proteomic data for the newly excysted juveniles 24 h post-excystment (NEJ 24), the immature fluke 21 days post-infection (Immature) and the adult parasites (Adult) is extrapolated from Cwiklinski et al. [Citation13], Cwiklinski et al. [Citation197] and Murphy et al. [Citation183], respectively. The protein abundance across the three stages, represented by the emPAI values detailed, is highlighted from low to high abundance by the yellow to green color scale, respectively. Proteins that are secreted in multiple isoforms, such as the cathepsin L peptidases, have been grouped together. The 12 uncharacterized proteins are shown as one group. *Abbreviated name for 4-methyl-5(B-hydroxyethyl)-thiazole monophosphate biosynthesis enzyme](/cms/asset/ee31dad1-547c-47d9-adab-ee96adec17b4/kvir_a_1996520_f0003_oc.jpg)
The high expression and subsequent excretion-secretion of five cathepsin cysteine peptidases, namely cathepsin L3 (FhCL3) and cathepsin B peptidases (FhCB1, FhCB2, FhCB3, and FhCB9), contribute to the rapid excystment of the metacercariae and subsequent invasion of the host by the NEJs [Citation13,Citation165–168]. In addition, three legumains are present within the cysts at twice the abundance of the cathepsin L and B peptidases, and are likely essential to speed up the trans-processing of the zymogen forms of the cathepsins to active peptidases [Citation41,Citation167,Citation169]. Unlike traditional cathepsins, F. hepatica cathepsin peptidases have acquired substitutions in their active site that enable these enzymes to degrade a diverse range of host ECM macromolecules including collagen, fibronectin and lamins [Citation170,Citation171], which is a common evolutionary adaptation shared by other invasive parasitic helminths [Citation172]. These peptidases were shown to play a pivotal role in the virulence of the parasite as RNA interference experiments targeting the cathepsin L and B genes significantly impaired the ability of the NEJs to penetrate through the intestinal wall [Citation173,Citation174].
More recently, the interaction of NEJs with the intestinal epithelia has also been shown to be implicated in initiating migration. Oligomannose-type N-glycans identified on the surface of NEJs play an integral role in this interaction, mediating contact between the parasite and the intestinal epithelia that signals NEJs to up-regulate essential factors required to penetrate through the intestinal wall [Citation168,Citation175]. Furthermore, blocking of these surface glycans significantly impaired the capacity of NEJs to attach and migrate through the intestine, highlighting the importance of tegumental carbohydrates in the pathogenesis of the parasite during the establishment of infection [Citation176].
Once in the abdominal cavity, the NEJs migrate toward the liver. However, in naïve animals, there is generally very little evidence of pathology associated with the migration of the NEJs during this early invasive stage [Citation177–179]. Several studies attribute the lack of host responses to the broad repertoire of virulence-associated factors expressed by NEJs, which often are involved in subverting the hosts’ capacity to elicit an immune response to stop invasion [Citation180]. It was suggested that this host immunomodulation occurs soon after excystment as NEJs begin interacting with the intestinal epithelia and down-regulating proteins related to ubiquitination [Citation168], a critical process required for intracellular signaling and subsequent triggering of the immune responses [Citation181]. Whilst the mechanism by which NEJs suppress ubiquitination remains unclear; the juvenile parasites express a plethora of molecules that are known to antagonize immune cell-signaling cascades.
As mentioned above, NEJs slough off and rapidly replace their outer tegmental surface as a means of evading surveillance and damage by the host immune response [Citation34,Citation182]. However, ES products and EVs released from the tegument also contribute to such escape. Several of the proteins identified in the secretome of NEJs lack a signal peptide and, thus, it has been suggested that the parasite uses EVs as an alternative route to deliver them into the host tissues where they have been observed to interact and modulate host cells [Citation183,Citation184]. Recent studies demonstrated that the EVs’ cargo contains not only proteins and glycoconjugates, but also specific miRNAs that inhibit mitogen-activated protein kinase (MAPK) signaling in macrophages and their subsequent capacity to respond to inflammatory stimuli [Citation185]. Fromm et al. [Citation43] also showed that these parasite EVs modulate the innate immune system of the host via specific miRNAs, which are enriched in EVs and taken up by host cells. In fact, the distribution of specific miRNAs was shown to vary greatly between EVs released by juveniles and adults stages, which might reflect the levels of interaction each lifecycle stage has with the host.
The peritoneal cavity is a critical location in the development of F. hepatica infection. It is not only the route of migration, but also the site where the parasite begins actively inducing an immunological environment that benefits their survival and likely plays a critical role in determining the ultimate outcome of the infection [Citation186]. In this environment, the NEJs have to deal with the rapid cellular and innate immune responses that are alerted by their presence. The parasites are thought to respond to this pressure and avoid, amongst other host defenses, the oxidant-mediated damage by increasing the expression of an array of antioxidant scavenger enzymes [Citation187]. However, Ruiz-Campillo et al. [Citation188] noted that NEJs in the peritoneum do not increase the expression of inducible nitric oxide synthases (iNOS), responsible for producing the toxic nitric oxide, indicating that the parasite anti-oxidant proteins are not involved in antioxidant defense at this stage, but in modulation of the immune response.
Several animal studies have demonstrated that experimentally-acquired or naturally-occurring resistance to F. hepatica infection requires a Th1-type immune response. In contrast, in susceptible hosts the dominant immune response elicited by the liver flukes is strongly polarized toward a T regulatory/Th2 phenotype [Citation189,Citation190], which is thought to help repair the damage caused as the parasites migrates through tissues. Several molecules expressed and excreted/secreted by NEJs have been shown to actively contribute toward establishing this regulatory/Th2 immunological environment, including the antioxidant enzyme FhPrx1, which induces the polarization of peritoneal macrophages toward an M2-like phenotype () [Citation189,Citation191]. Similarly, secreted glycoproteins and glycoconjugates on the tegumental surface of NEJs are also heavily implicated in the recruitment and modulation of dendritic cells (DCs) and M2-macrophages in the peritoneal cavity. These cells secrete cytokines that favor the induction of Th2/regulatory immune responses [Citation19,Citation20,Citation175].
The migration of NEJs in the abdominal cavity can last for up to a week, but some immature flukes have been observed in the liver parenchyma as early as 3 days post infection [Citation162]. However, not all NEJs reach the liver. Many experimental infection studies have demonstrated that infectivity is always below 100%, whereby the number of mature flukes recovered in the hepatic canals is never equal to the number of infective forms administered [Citation160,Citation192]. In part, this is due to a portion of NEJs failing to excyst or penetrate the gut wall. Moreover, a thickening of the external fibrous layer of the liver occurs in response to damage induced by NEJs that have already penetrated the liver parenchyma, rendering it less amenable to late-arriving NEJs or subsequent infections. Therefore, either because of their inability to penetrate this hardened tissue or due to the unfavorable environment and less readily available nutrients as a result of liver damage, some NEJs fail to develop into mature adult flukes. These unsuccessful NEJs may migrate through the diaphragm instead, causing occasional hemorrhagic tracts and necrotic lesions in the thoracic cavity [Citation25,Citation193]. This is more evident as the infective dose is increased, resulting in a crowding effect that lowers overall percent take of infective doses [Citation192,Citation194].
Mechanism of migration through the liver parenchyma
Liver pathogenesis associated with fasciolosis arises from a complex interplay between host and parasite. It is instigated by a combination of mechanical and enzymatic damage caused by the parasite’s migratory and feeding activities in the parenchyma, in addition to the host’s inflammatory immune responses aimed at repairing the ensuing tissue damage, and eliminating the parasite [Citation195].
Liver fluke-induced liver damage
Damage to the liver parenchyma ensues following penetration of the Glisson’s capsule, whereby the NEJs migrate into the liver by tunneling a path through the connective tissue between the fibrillary collagen bundles [Citation196]. In the case of infection with F. hepatica, this damage continues over the course of approximately eight to 10 weeks, as the parasite continues to burrow through the liver tissue. It occurs by mechanical means aided by the oral and ventral suckers, as illustrated by the presence of hepatic cells inside the suckers [Citation38,Citation138], and by the proteolytic actions of the parasite secreted enzymes such as cathepsin L peptidases (FhCL2 and FhCL3) [Citation197–199]. These cathepsin L peptidases are abundantly secreted by the liver migratory stages () [Citation197] and display potent collagenolytic activity capable of degrading insoluble collagen, which leads to the destruction of the liver ECM and facilitates passage of the parasite [Citation200].
During the liver migratory phase, the liver fluke’s digestive system undergoes rapid development [Citation201]. This allows the parasite to actively feed on host tissue and blood rather than relying on endogenous glycogen stores, expediting growth and development. In addition to FhCL2 and FhCL3, at 21 days post-infection the parasites begin to secrete an array of peptidases involved in the digestion of blood, reflecting the transition to obligate blood feeding () [Citation197].
Liver repair mechanisms
The liver is a unique organ with the capacity to not only repair but also regenerate following injury [Citation202,Citation203]. Penetration of the liver by parasites initiates a wound healing response, which in sheep is characterized by an influx of lymphocytes, macrophages and eosinophils, and the induction of fibrosis to repair the damage [Citation186]. This leads to the subsequent formation of visible fibrotic hepatic tracts and granulomas, which are correlated with increased levels of Foxp3 + T regulatory cells that may play a role in reducing tissue pathology [Citation204], overexpression of regulatory cytokines (IL-10 and TGF-β), and pro-inflammatory cytokines (TNF-α and IL-1β) [Citation205]. However, in the case of liver fluke infections in the field, animals can be continually re-infected, perpetuating the damage and wound-healing repair and resolution mechanisms and eventually compromising overall liver function () [Citation186,Citation206].
The delicate interplay between mammalian host and parasite results in different severities of clinical signs and liver pathology depending on the host species. In sheep and goats that are highly susceptible to reinfection, the migratory tracts are surrounded by an infiltration of immune cells as described above, resulting in inflammation and the formation of granulomas [Citation186]. In contrast, infection in cattle causes more extensive fibrosis and less visible tracts, which is thought to play a role in the partial resistance to reinfection, but can progress to cirrhosis of the liver in severe cases [Citation90].
Evasion and modulation of host immune responses
During the migratory phase, the liver fluke parasites employ several methods to escape the host immune response to ensure their survival. In addition to evading the influx of immune cells directed to their migratory path by rapidly migrating through the liver parenchyma [Citation37,Citation207], the parasites stimulate polarization of the host immune responses toward a Th2/T regulatory phenotype.
The secretome of the immature 21-day parasites found in the liver is dominated by cathepsin peptidases and their inhibitors [Citation197]. In addition to their role in tissue degradation and feeding, the cathepsin L peptidases have also been shown to cleave the Fc domains of immunoglobulins, preventing antibody-mediated attachment of host immune effector cells and complement activation [Citation208,Citation209], both of which are protective mechanisms required to clear the parasite in some resistant animal species [Citation210]. Moreover, cathepsin-L peptidases are internalized by host immune cells and degrade the pathogen recognition receptor Toll-like Receptor 3 (TLR-3), preventing TRIF-dependent signaling that is crucial for the development of Th1 inflammatory responses that are harmful to the parasite’s survival [Citation211]. This is complemented by the cathepsin peptidase inhibitor, Kunitz type inhibitor, FhKT1, which has also been shown to prevent the development of Th1 and Th17 responses by regulating LPS-stimulated dendritic cells in an IL-27 dependent manner [Citation212]. The liver migrating parasites also secrete several proteins that play a role in the reduction of pro-inflammatory responses, including the FhHDM [Citation213,Citation214] and several FABP; Fh2, Fh3, Fh15 [Citation215–218]. In addition, interaction with the glycans associated with the parasite tegument and ES proteins has been shown to regulate the maturation and function of CD11c+ dendritic cells that are recruited to the liver, where they drive Th2/T regulatory polarization of the host immune response [Citation219].
The ES products also play a role in moderating the eosinophils recruited to repair and regulate liver damage during fasciolosis [Citation220], by inducing apoptosis in the liver-associated eosinophils [Citation221]. This is comparable to the in vitro apoptotic effects of the ES proteins on peritoneal eosinophils and macrophages [Citation222–224]. Furthermore, transcriptional analysis revealed that pro-apoptotic signals are increased in peripheral blood mononuclear cells recovered from F. hepatica infected sheep and cattle [Citation225,Citation226]. The specific proteins that bring about this cellular apoptosis have yet to be characterized, and further studies are required to determine whether similar parasite proteins are involved within the different immune compartments of the infected animal.
Recently, in silico analysis has revealed that Fasciola-specific miRNAs may also play a role in regulating the recruitment and functionality of key innate immune cells, targeting several host genes related to dendritic cells, neutrophils and eosinophils [Citation227]. Consistent with their effect on the host immune cells, in vitro analyses have shown that the F. hepatica ES proteins can also have a direct effect on the liver hepatocytes, reducing their metabolism and overall survival [Citation228–230].
Fasciola spp. associated oxidative stress in the liver
A characteristic feature of fasciolosis are the high levels of oxidative stress associated with the pathology caused by the migrating parasites and the resulting host damage repair mechanisms, which both host and parasite must contend with [Citation231–233]. The host’s first line defense responses quickly become overrun, and down-regulation of proteins such as SOD and catalase occurs concomitantly to increasing levels of oxidative stress associated with the parasite-induced liver fibrosis [Citation234–237]. This results in a switch to glutathione thiol-dependent based antioxidants, with increased transcription of glutathione peroxidase (GPx) and glutathione S transferases (GSTs) by the host [Citation197]. In response, F. hepatica expresses and secretes an abundance of FhGSTs, FhTrx and FhSOD during the liver stage, indicating that the parasite utilizes a combination of thioredoxin and glutathione thiol-dependent antioxidant systems to counter-attack the levels of damaging reactive oxygen species (ROS) in their environment [Citation197]. Similarly, survival of the parasite within the bile ducts also depends on its ability to eliminate ROS (i.e., hydrogen peroxide and superoxide) generated by host immune effector cells such as macrophages and eosinophils. Consequently, an up-regulation of FhTGR, FhTrx, and FhPrx is also observed within the adult parasites [Citation13,Citation30,Citation183,Citation210].
Consistent with many genes within the F. hepatica genome, the repertoire of genes involved in anti-oxidant defenses have undergone gene duplication and expanded into larger gene families [Citation12]. This has resulted in FhTrx and FhPrx members with extended functions that play a role in the parasite-host interplay. Although these molecules generally function in an interdependent manner, by FhTrx reducing and activating FhPrx, recently it was proposed that F. hepatica Prx1 and Trx1 could also work autonomously [Citation187]. Moreover, both FhPrx1 and FhTrx1 may contribute to host immune evasion by inducing Th2 immune responses [Citation187,Citation189,Citation191].
The bile-associated parasite stages also produce and secrete high levels of proline, which has been shown to be involved in bile duct hyperplasia during the chronic phases of infection [Citation238–241]. Proline may also play an important role in stabilizing the antioxidant enzymes, in addition to its role in direct scavenging of ROS [Citation197,Citation242,Citation243].
Adult flukes in the bile ducts
Fasciola flukes reach the biliary ducts of the mammalian host approximately 10 to 12 weeks post infection, marking the beginning of the chronic phase of the fasciolosis. This is considered a safe environment for the parasites, away from most of the components of the host innate and acquired immune responses. In this compartment, the liver flukes may live for several decades. They move along the biliary network while feeding on blood, bile, lymph, and tissue fragments, which they use as a source of energy to produce eggs [Citation39]. Although most chronic infections are asymptomatic, pathology at this stage can be severe, and is often related to the number of parasites that reach the bile duct. The physical presence of numerous flukes in the bile duct causes abrasion and even blockage of the bile circulation [Citation244]. Hence, the host species and the host’s overall health, in addition to the parasite species and the burden of infection are all factors that influence pathology during this stage of infection.
The mechanical damage observed during blood feeding within the bile duct is mainly due to the parasites spines, which puncture small blood vessels causing erosion of the epithelium [Citation27,Citation138,Citation245]. In severe infection, extensive damage can cause some parasite eggs to leak out into the liver parenchyma, leading to eosinophilic and granulomatous inflammatory responses [Citation37]. In addition, pathology is exacerbated by the continuous release of fluke molecules into the host biliary network [Citation41,Citation246], which is illustrated by the ability of F. hepatica adult ES products alone to induce damage and enlargement of the bile duct [Citation247,Citation248]. As expected, the presence of liver flukes in the bile ducts is marked by a spike of liver enzyme levels, such as GGT and AP, in serum. The increase of AP levels has been linked to the establishment of adult flukes in the bile ducts and hepato-biliary obstruction, which in turn stimulates de novo synthesis of the hepatic AP [Citation105]. Indeed, in buffalo infected with F. gigantica, bile obstruction was associated with a 107.9% increase in serum AP concentrations [Citation105].
Adult flukes have adapted to survive within the bile. Their tegument is tough and resistant to bile, which consists of a mixture of bile salts, lipids, amino acids, enzymes, and heavy metals, as well as exogenous drugs and toxins that the host consumes [Citation249]. Moreover, the parasites thrive in such an environment by adjusting their metabolism and varying protein expression. For example, the resistance of certain F. hepatica isolates to the drug salicylanilide has been linked to increased expression of FhGST [Citation250], and an amino acid substitution in position 143 of the GST was shown to increase TCBZ susceptibility of isolates [Citation251].
The flukes tolerate the low oxygen levels in the bile by expressing high-oxygen affinity hemoglobin [Citation252] and by activating genes involved in anaerobic glycolysis, which allow the fluke to be a facultative anaerobe [Citation253]. Similarly, adult liver flukes can metabolize lipids (i.e., LDL, VLDL, HDL) that are present in large amounts in the bile, and such activity can, eventually, be reflected in the host serum levels of lipids and triglycerides [Citation84,Citation254]. Indeed, humans may develop gallstone disease after months to years of infection, often during the obstructive phase of fasciolosis [Citation142,Citation255,Citation256]. Ultimately, obstruction of the bile ducts arises from both the parasite’s presence and its ES products that cause inflammation and hyperplasia of the epithelium, contributing to the enlargement and mineralization of the bile ducts (cholangitis) and gall bladder (cholecystitis) [Citation255,Citation256].
Similar to the liver-associated stage, the mature adult releases ES products rich in cathepsin L and B peptidases, legumains, peptidase inhibitors, enzymes, glycoproteins and FhHDM () [Citation3,Citation13–15,Citation41,Citation253,Citation257]. Several biochemical and immunological studies have shown the importance of many of these molecules for parasite feeding and detoxification of bile components, as well as their roles in the evasion of host immune responses [Citation170,Citation189,Citation191,Citation210,Citation258–260].
Without doubt, the adult fluke ES products change the bile composition of infected animals but the systemic effects of these molecules released with the bile are still undefined. Through enterohepatic circulation the bile synthesized in the liver is released into the small intestine where it helps in the digestive processes by acting as a detergent. Subsequently, about 95% of the bile contents are reabsorbed in the distal ileum [Citation261]. Hence, the composition of the bile has important implications for the homeostasis of the intestine and liver. During fasciolosis, bile circulation in the gut and liver will transport parasite antigens to distant sites, which may contribute to the immune stimulation observed in the host, even during the chronic phase of the disease [Citation104]. Morphew et al. [Citation262] showed large amounts of F. hepatica cathepsin L peptidases in bile fluid collected from naturally infected sheep. Similarly, anti-cathepsin L IgG and IgA antibodies are present in bile, although in considerably lower levels than in serum [Citation263]. These data support the idea that even when hidden in the bile ducts, the adult liver flukes are still capable of stimulating the host immune system. This might explain why the titer of specific anti-cathepsin L antibodies in infected animals’ serum remains high even after the immature stages have left the liver, and only drops after treatment with TCBZ and removal of adult flukes [Citation104,Citation264].
As the main nutrients for adult flukes are derived from blood digestion, at this stage the parasites express several proteins related to host hemoglobin digestion and metabolism, namely FhCL1, leucine aminopeptidases (FhLAP), myoglobin, ferritins, prolylcarboxypeptidase, saposins and FhHDM [Citation12,Citation30,Citation41,Citation259,Citation265]. The process of blood digestion involves the lysis of red blood cells by saposins, releasing hemoglobin that is digested into small peptides by FhCL1, followed by the terminal degradation of hemoglobin peptides by FhLAP () [Citation266–269]. Moreover, heme-binding proteins such as FhHDM play essential roles in detoxifying heme, which is the main product of the metabolism of hemoglobin [Citation270,Citation271]. As Fasciola spp. are unable to form hemozoin crystals to eliminate the heme, it secretes high amounts of FhHDM that forms high-molecular weight complexes with heme, inhibiting its harmful peroxidase-associated activity [Citation265,Citation272].
The major components of bile have been investigated by proteomic analysis, which revealed a range of abundant proteins including albumin and immunoglobulins, complement components, coagulation factors (e.g., kallikrein, fibrinogen and anti-thrombin), digestive enzymes such as trypsin, elastase, chymotrypsin and various peptidase inhibitors [Citation14,Citation30,Citation273]. To cope with these host factors, Fasciola adult parasites express a range of molecules that are secreted and/or attached to the parasite surface, including serine peptidases inhibitors (serpins). We have recently characterized two F. hepatica serpins, FhSrp1 and FhSrp2, which appear to be deliberately expressed to inhibit host chymotrypsin and kallikrein [Citation17]. Both serpins were located on the surface of the immature parasites, but are also highly prevalent in the ES products of all F. hepatica life stages [Citation15,Citation17]. The F. hepatica serpin family includes seven members, and further characterization of these molecules might link them to the regulation of cascades in which serine peptidases play a central role (e.g., coagulation and complement systems).
Transcriptome and proteome analysis of F. hepatica and F. gigantica adult parasites and the respective repertoire of secreted proteins from this lifecycle stage has revealed that proteins involved in glycolytic processes are up-regulated [Citation3,Citation183]. Aldolase (fructose-bisphosphate), enolase, and glyceraldehyde 6-phosphate dehydrogenase (GAPDH) are among the enzymes the Fasciola spp. flukes either secrete or attach to their tegument surface, where they act mainly as ligands for a variety of host components [Citation3]. As such, these molecules contribute to invasion, modulation of the host’s immune and hemostatic systems, angiogenesis, and acquisition of nutrients [Citation42,Citation274–277]. These enzymes were also characterized as plasminogen-binding proteins, and thus were linked to plasmin generation activity [Citation277]. As plasmin degrades fibrin, this could be the mechanism by which the adult parasites prevent clot formation during blood feeding (). In addition, peptides released during fibrin degradation might act as regulators of fibrinogen, reducing fibrin formation that could restrain the parasites.
F. gigantica – not just another fluke
Despite affecting human and livestock health in an area that represents up to 77% of the global population, research interest in F. gigantica consistently lags behind that of F. hepatica [Citation278]. As a consequence of this neglect, far less is known about the factors contributing to the pathogenicity and virulence of this species. Recent increases in reports of hybrid or introgressed forms between F. hepatica and F. gigantica in areas where they co-exist suggest the potential for adaptive introgression of various traits between the two species that may enhance their pathogenicity and virulence in mammalian hosts, warranting further investigation [Citation279–282].
The lifecycle of F. gigantica follows a similar progression to that of F. hepatica, including the reliance on an aquatic intermediate snail host. After infection of the definitive mammalian hosts by ingestion, F. gigantica metacercariae excyst in the small intestines before reaching the liver via the abdominal cavity, where they migrate for a period of up to 16 weeks [Citation283–285]. Mature adults reside in the bile ducts of infected hosts and shed eggs into their feces. Under optimal conditions, the eggs of F. gigantica hatch after a period of 10–11 days, releasing the short-lived miracidia that must find a suitable intermediate snail hosts to continue the parasite lifecycle [Citation286].
The lifecycle similarities between F. hepatica and F. gigantica have led to the erroneous assumption that their epidemiologies, and therefore their ability to cause disease in infected mammalian hosts, are similar. Differences between the susceptibility of various species and breeds of mammalian hosts, on the other hand, have prompted the conclusion that F. gigantica is less virulent than F. hepatica. As it stands, however, there is limited empirical data available to support current conclusions comparing the pathogenicity and virulence of F. gigantica to that of F. hepatica, and existing information must be interpreted with an appreciation for the differences between these two parasites.
Virulence and pathogenicity in mammalian hosts
Several studies have attempted to compare the virulence of F. gigantica to F. hepatica in mammalian hosts by contrasting the percentage take of infective metacercariae during experimental infections along with the impact of infection on immunological and biochemical markers [Citation287–294]. A reliance on small sample sizes (1–5 animals/group) and large varying infectious doses (500–20,000 metacercariae/dose) have limited the statistical significance of these findings and their application to our understanding of natural infections, making it difficult to determine if one species is truly more virulent and/or pathogenic than the other. The length of time post infection has also been shown to influence the number of parasites available for recovery from the liver, with fewer F. gigantica adults present in the livers of infected cattle from 5 months post infection [Citation284]. What is clear, however, is that a difference in susceptibility to F. gigantica infection exists not only between different species of mammalian hosts, but also between different breeds within the same host species. These differences may help us to infer the mechanisms of innate and acquired resistance and immune-based pathogenesis against infection with F. hepatica and F. gigantica, as well as help us shed light on the defense mechanisms employed by the parasites when under attack by the host’s immune response.
Swamp buffalo appear to be the most resilient mammalian host to infection with F. gigantica, as demonstrated by lower parasite burdens, reduced fecal egg counts, less apparent clinical signs and less significant impact on biochemical parameters such as packed cell volume (PCV), GGT and LDH compared to various Bos indicus cattle breeds exposed to the same infectious dose [Citation285,Citation295–297]. Global serum, liver, hepatic lymph node and spleen proteome analysis has recently been conducted on experimentally infected riverine buffaloes in order to elucidate the mechanisms of host responses to infection during the invasive (3–10 days post infection; DPI), early (28–70 DPI) and late (≥ 98 DPI) stages of infection [Citation298]. These analyses have revealed the downregulation of metabolic processes in infected host liver throughout infection and a shift toward redox processes during early infection, likely as a form of offense against the invading immature flukes [Citation298]. Similarly, Indonesian thin tail (ITT) sheep have been shown to have a high level of resistance to infection against F. gigantica via the generation of both a strong innate and adaptive immune response [Citation287–289,Citation291]. Interestingly, ITT are susceptible to infection with F. hepatica, suggesting that this parasite species is more adept at modulating the host response to infection [Citation287,Citation291]. Proteomic and transcriptional analyses of liver, serum and hepatic lymph nodes during experimental infection with F. hepatica and F. gigantica in this species and its comparison to existing datasets from water buffalo may help shed light on the exact processes involved in parasite invasion and evasion of host immune responses.
Recent studies by Zhang et al. [Citation299] applied proteomic techniques to identify a signature of F. gigantica infection in serum from swamp buffaloes at 3, 42 and 70 DPI. Six significantly up-regulated proteins were identified in infected serum compared to uninfected buffaloes, namely MHC I antigen, microglobulin, NID2 protein, fetuin-B and fibrinogen gamma-B chain. Histopathological examination of hosts infected with F. gigantica revealed cellular infiltration, hemorrhage and fibrosis without calcification in the liver parenchyma, which increased over the course of infection [Citation300]. This pathogenesis has been attributed to the suppression of the host’s pro-inflammatory responses, emphasized by low levels of cytokines such as interleukin-1β (IL-1β), IL-2, IL-6, IL-12, and IFN-γ [Citation301], and changing in the expression profile of genes involved in TLRs and NOD-like receptors (NLRs) signaling pathways in serum, liver and peripheral blood mononuclear cell (PBMC) of infected buffaloes [Citation302]. During the early stages of infections (3–13 DPI), a mixed Th1- and Th2-type immune response is observed, which is thought to facilitate the parasite’s establishment [Citation300,Citation301,Citation303]. Conversely, systemic immunological analysis of the serum and lymphoid organs of infected animals 98 DPI revealed that during chronic infection the host responses are completely skewed toward a Th2 pattern. This is illustrated by enhanced expression of IL-4 and the IgG1 antibody isotype [Citation300,Citation303]. Furthermore, the strength of the Th2 response elicited is thought to be indicative of the susceptibility of the host species to F. gigantica infection [Citation303]. Unlike F. hepatica, factors responsible for modulating the immune response during infection with F. gigantica remain largely unknown. However, studies have demonstrated that F. gigantica ES products play an important role by suppressing maturation of immune cells such as DCs [Citation304], as well as altering the expression of genes associated with the host immune responses, receptor signaling, disease and metabolism [Citation305]. Recent mass spectrometry analysis of F. gigantica ES has identified many of the same virulence associated proteins involved in immune modulation by F. hepatica, including cathepsin L and B peptidases, antioxidants and FABPs [Citation306], but as to whether they exert similar modulatory effects remains to be determined.
There are fewer reports of human infections with F. gigantica than with F. hepatica, leading to the assumption that F. hepatica is more pathogenic in areas where human fasciolosis is common [Citation46,Citation255,Citation307]. The tendency for F. gigantica to occur in less-developed regions where access to medical facilities is limited, however, suggests that perhaps human cases of F. gigantica infection are simply underreported. There are also suggestions that F. gigantica may be less virulent in human infections, resulting in a milder form of disease that causes less pain and therefore goes unnoticed for longer [Citation307,Citation308]. The production of a higher proportion of floating cysts by F. gigantica compared to F. hepatica may provide additional sources of infection such as via the use of contaminated water for washing otherwise safe vegetables or through drinking [Citation76]. The occurrence of F. gigantica in regions maintaining the livelihoods of up to 6 billion people further supports the suggestion that human cases of F. gigantica infection are equally – if not more – prevalent than F. hepatica, and are simply undocumented.
Fasciola-hybrid or introgressed forms
Increasing reports of hybridization and/or introgression between F. hepatica and F. gigantica have raised the possibility of the existence of Fasciola spp. with intermediate pathogenicity and virulence traits [Citation278]. Experimentally, hybridization between F. hepatica and F. gigantica has been demonstrated under laboratory conditions and the continued identification of these forms in field samples suggests that they are a continually occurring phenomenon [Citation309–311]. While studies on the functional implications of these genetic events are currently unavailable, lab-maintained Fasciola-hybrid adults demonstrated an intermediate body size between that of their parent species and are considered more infectious in Wistar rats than F. gigantica alone based on higher recovery rates [Citation309]. Hybridization between these two parasites may not necessarily generate permanent hybrid strains and yet the potential for introgression of advantageous traits between these two species as a result of the back-crossing of hybrids is worthy of further consideration [Citation278].
Increasing areas of parasite sympatry as a result of international livestock movements, combined with climate change-derived shifts in conditions suitable for the survival of both species, suggests that future work should be directed toward understanding the potential human and animal health risks associated with these genetic events, including potential impacts on their pathogenicity and virulence [Citation106,Citation278]. Furthermore, as TCBZ resistant F. hepatica continue to spread across the globe the potential for the emergence of drug-resistant Fasciola hybrids should be closely monitored, particularly since this is the only drug available for the treatment of human fasciolosis.
Conclusion
Fasciolosis caused by flatworms of the species Fasciola have been scourges of farmed animals for centuries but only in recent decades has their zoonotic importance become realized. There is no vaccine available and, despite much progress in understanding the biology of the parasites and experimental research toward this goal, it is unlikely that we will see one in the next five years. Meanwhile, parasites that are resistant to frontline drugs such as triclabendazole are continuing to spread globally leaving farmers and veterinarians without a means of controlling on-farm disease and medics without an effective treatment for human infection with drug-resistant parasites. Climate change is also impacting on the prevalence and distribution of the disease [Citation120] and live animal trade is helping to fast-forward the spread of new species or isolates to new regions as well has promoting the expansion of hybrid F. hepatica/gigantica parasite forms [Citation278]. Research advances are therefore indispensable to overcome these issues. As shown in this review, great advances in molecular biology, genomics/genetics and -omics are allowing us to develop a detailed molecular picture of parasite infection, virulence and pathogenicity. This information is advancing our understanding of the parasite-host interactions, enabling the development of effective control strategies (vaccines and drugs), as well as diagnostic tools that will ultimately allow us to identify and treat infections, which is fundamental to prevent both disease spread and economic losses that result from both F. hepatica and F. gigantica.
Disclosure statement
No potential conflict of interest was reported by the author(s).
Data Availability
Data sharing is not applicable to this article as no new data were created or analysed in this study.
Additional information
Funding
References
- Mas-Coma S, Valero MA, Bargues MD. Chapter 2. Fasciola, lymnaeids and human fascioliasis, with a global overview on disease transmission, epidemiology, evolutionary genetics, molecular epidemiology and control. Adv Parasitol. 2009;69:41–146.
- Sripa B. Global burden of food-borne trematodiasis. Lancet Infect Dis. 2012;12(3):171–182.
- Zhang XX, Cwiklinski K, Hu RS, et al. Complex and dynamic transcriptional changes allow the helminth Fasciola gigantica to adjust to its intermediate snail and definitive mammalian hosts. BMC Genomics. 2019;20(1):729.
- Hotez PJ, Brindley PJ, Bethony JM, et al. Helminth infections: the great neglected tropical diseases. J Clin Investig. 2008;118(4):1311–1321.
- Tolan R. Fascioliasis due to Fasciola hepatica and Fasciola gigantica infection: an update on this neglected tropical disease. Lab Med. 2011;42(2):107–116.
- Mazeri S, Rydevik G, Handel I, et al. Estimation of the impact of Fasciola hepatica infection on time taken for UK beef cattle to reach slaughter weight. Sci Rep. 2017;7(1):7319.
- Bennema SC, Scholte RG, Molento MB, et al. Fasciola hepatica in bovines in Brazil: data availability and spatial distribution. Rev Inst Med Trop São Paulo. 2014;56(1):35–41.
- Copeman DB, Copland RS. Importance and potential impact of liver fluke in cattle and Buffalo. In: Gray RSC GD, Copeman DB, editors. Overcoming liver fluke as a constraint to ruminant production in South-East Asia. ACIAR monograph series. Canberra Australia: Australian Centre for International Agricultural Research; 2008. p. 1–34.
- Caravedo MA, Cabada MM. Human fascioliasis: current epidemiological status and strategies for diagnosis, treatment, and control. Res Rep Tropical Med. 2020;11:149–158.
- Fürst T, Keiser J, Utzinger J. Global burden of human food-borne trematodiasis: a systematic review and meta-analysis. The Lancet Infectious Diseases. 2012;12(3):210–221.
- McNulty SN, Tort JF, Rinaldi G, et al. Genomes of Fasciola hepatica from the Americas reveal colonization with neorickettsia endobacteria related to the agents of potomac horse and human sennetsu fevers. PLoS Genet. 2017;13(1):e1006537.
- Cwiklinski K, Dalton JP, Dufresne PJ, et al. The Fasciola hepatica genome: gene duplication and polymorphism reveals adaptation to the host environment and the capacity for rapid evolution. Genome Biol. 2015;16:71.
- Cwiklinski K, Jewhurst H, McVeigh P, et al. Infection by the helminth parasite Fasciola hepatica requires rapid regulation of metabolic, virulence, and invasive factors to adjust to its mammalian host. Mol Cell Proteomics. 2018;17(4):792–809.
- Di Maggio LS, Tirloni L, Pinto AF, et al. Across intra-mammalian stages of the liver fluke Fasciola hepatica: a proteomic study. Sci Rep. 2016;6:32796.
- Cwiklinski K, Dalton JP. Advances in Fasciola hepatica research using ‘omics’ technologies. Int J Parasitol. 2018;48(5):321–331.
- Cwiklinski K, Donnelly S, Drysdale O, et al. The cathepsin-like cysteine peptidases of trematodes of the genus Fasciola. Adv Parasitol. 2019;104:113–164.
- De Marco Verissimo C, Jewhurst HL, Tikhonova IG, et al. Fasciola hepatica serine protease inhibitor family (serpins): purposely crafted for regulating host proteases. PLoS Negl Trop Dis. 2020;14(8):e0008510.
- Smith D, Tikhonova IG, Jewhurst HL, et al. Unexpected activity of a novel Kunitz-type inhibitor: inihibition if cysteine proteases but not serine proteases. J Biol Chem. 2016;291(37):19220–19234.
- Ravida A, Aldridge AM, Driessen NN, et al. Fasciola hepatica surface coat glycoproteins contain mannosylated and phosphorylated N-glycans and exhibit immune modulatory properties independent of the mannose receptor. PLoS Negl Trop Dis. 2016;10(4):e0004601.
- Rodriguez E, Noya V, Cervi L, et al. Glycans from Fasciola hepatica modulate the host immune response and TLR-Induced maturation of dendritic cells. PLoS Negl Trop Dis. 2015;9(12):e0004234.
- Dalton JP, Skelly P, Halton DW. Role of the tegument and gut in nutrient uptake by parasitic platyhelminths. Can J Zool. 2004;82(2):211–232.
- Berasain P, Carmona C, Frangione B, et al. Fasciola hepatica: parasite-secreted proteinases degrade all human IgG subclasses: determination of the specific cleavage sites and identification of the immunoglobulin fragments produced. Exp Parasitol. 2000;94(2):99–110.
- Rondelaud D, Belfaiza M, Vignoles P, et al. Redial generations of Fasciola hepatica: a review. J Helminthol. 2009;83(3):245–254.
- Sumruayphol S, Siribat P, Dujardin JP, et al.Fasciola gigantica, F. hepatica and Fasciola intermediate forms: geometric morphometrics and an artificial neural network to help morphological identification. PeerJ. 2020;8:e8597.
- Boray JC. Experimental fascioliasis in Australia. Adv Parasitol. 1969;7:95–210.
- Graczyk TK, Fried B. Development of Fasciola hepatica in the intermediate host. Fasciolosis: CABI Publishing. Editor: Dalton JP. 1999. p. 31–46.
- Dow C, Ross JG, Todd JR. The pathology of experimental fascioliasis in calves. J Comp Pathol. 1967;77(4):377–385.
- van Milligen FJ, Jan BWJC, Bokhout BA. Location of induction and expression of protective immunity against Fasciola hepatica at the gut level: a study using an ex vivo infection model with ligated gut segments. J Parasitol. 1998;84(4):771–777.
- Bils RF, Martin WE. Fine structure and development of the trematode integument. Trans Am Microscopical Soc. 1966;85(1):78–88.
- Wilson RA, Wright JM, De Castro-borges W, et al. Exploring the Fasciola hepatica tegument proteome. Int J Parasitol. 2011;41(13–14):1347–1359.
- Bennett CE, Threadgold LT. Fasciola hepatica: development of tegument during migration in mouse. Exp Parasitol. 1975;38(1):38–55.
- Bennett CE. Scanning electron microscopy of Fasciola hepatica L during growth and maturation in the mouse. J Parasitol. 1975;61(5):892–898.
- Jefferies JR, Campbell AM, van Rossum AJ, et al. Proteomic analysis of Fasciola hepatica excretory-secretory products. Proteomics. 2001;1(9):1128–1132.
- Hanna RE. Fasciola hepatica: glycocalyx replacement in the juvenile as a possible mechanism for protection against host immunity. Exp Parasitol. 1980;50(1):103–114.
- Han JK, Choi BI, Cho JM, et al. Radiological findings of human fascioliasis. Abdominal Imaging. 1993;18(3):261–264.
- Mas-Coma S, Valero MA, Bargues MD. Fascioliasis. Adv Exp Med Biol. 2014;766:77–114.
- Zafra R, Perez-Ecija RA, Buffoni L, et al. Early hepatic and peritoneal changes and immune response in goats vaccinated with a recombinant glutathione transferase sigma class and challenged with Fasciola hepatica. Res Vet Sci. 2013;94(3):602–609.
- Dawes B, Hughes DL. Fascioliasis: the Invasive stages of Fasciola hepatica in mammalian hosts. Adv Parasitol. 1964;2:97–168.
- Kotpal R. Modern Text Book of Zoology: invertebrates. New Delhi: Rastogi Publications; 2012.
- Hanna REB, Trudgett AG. Fasciola hepatica: development of monoclonal antibodies and their use to characterize a glycocalyx antigen in migrating flukes. Parasite Immunol. 1983;5(4):409–425.
- Robinson MW, Menon R, Donnelly SM, et al. An integrated transcriptomics and proteomics analysis of the secretome of the helminth pathogen Fasciola hepatica: proteins associated with invasion and infection of the mammalian host. Mol Cell Proteomics. 2009;8(8):1891–1907.
- de la Torre-Escudero E, Gerlach JQ, Bennett APS, et al. Surface molecules of extracellular vesicles secreted by the helminth pathogen Fasciola hepatica direct their internalisation by host cells. PLoS Negl Trop Dis. 2019;13(1):e0007087.
- Fromm B, Trelis M, Hackenberg M, et al. The revised microRNA complement of Fasciola hepatica reveals a plethora of overlooked microRNAs and evidence for enrichment of immuno-regulatory microRNAs in extracellular vesicles. Int J Parasitol. 2015;45(11):697–702.
- Burton JB, Carter CE, Oeltmann TN. Human parasitology. 4 ed ed. Amsterdam; Boston: Academic Press; 2012.
- Beesley NJ, Williams DJ, Paterson S, et al. Fasciola hepatica demonstrates high levels of genetic diversity, a lack of population structure and high gene flow: possible implications for drug resistance. Int J Parasitol. 2017;47(1):11–20.
- Mas-Coma S, Bargues MD, Valero MA. Human fascioliasis infection sources, their diversity, incidence factors, analytical methods and prevention measures. Parasitology. 2018;145(13):1665–1699.
- Kelley JM, Elliott TP, Beddoe T, et al. Current threat of triclabendazole resistance in Fasciola hepatica. Trends Parasitol. 2016;32(6):458–469.
- Hodgkinson J, Cwiklinski K, Beesley NJ, et al. Identification of putative markers of triclabendazole resistance by a genome-wide analysis of genetically recombinant Fasciola hepatica. Parasitology 2013;140(12):1523–1533.
- Fairweather I. Liver fluke isolates: a question of provenance. Vet Parasitol. 2011;176(1):1–8.
- Ortiz P, Scarcella S, Cerna C, et al. Resistance of Fasciola hepatica against triclabendazole in cattle in Cajamarca (Peru): a clinical trial and an in vivo efficacy test in sheep. Vet Parasitol. 2013;195(1–2):118–121.
- Radio S, Fontenla S, Solana V, et al. Pleiotropic alterations in gene expression in Latin American Fasciola hepatica isolates with different susceptibility to drugs. Parasit Vectors. 2018;11(1):56.
- McConville M, Brennan GP, Flanagan A, et al. An evaluation of the efficacy of compound alpha and triclabendazole against two isolates of Fasciola hepatica. Vet Parasitol. 2009;162(1–2):75–88.
- Walker SM, Hoey E, Fletcher H, et al. Stage-specific differences in fecundity over the life-cycle of two characterized isolates of the liver fluke, Fasciola hepatica. Parasitology 2006;133((Pt 2)):209–216.
- Hanna RE, Edgar H, Moffett D, et al. Fasciola hepatica: histology of the testis in egg-producing adults of several laboratory-maintained isolates of flukes grown to maturity in cattle and sheep and in flukes from naturally infected hosts. Vet Parasitol. 2008;157(3–4):222–234.
- Fletcher HL, Hoey EM, Orr N, et al. The occurrence and significance of triploidy in the liver fluke, Fasciola hepatica. Parasitology 2004;128((Pt 1)):69–72.
- Walker SM, Prodohl PA, Fletcher HL, et al. Evidence for multiple mitochondrial lineages of Fasciola hepatica (liver fluke) within infrapopulations from cattle and sheep. Parasitol Res. 2007;101(1):117–125.
- Hodgkinson JE, Cwiklinski K, Beesley N, et al. Clonal amplification of Fasciola hepatica in Galba truncatula: within and between isolate variation of triclabendazole-susceptible and -resistant clones. Parasit Vectors. 2018;11(1):363.
- Fox NJ, White PC, McClean CJ, et al. Predicting impacts of climate change on Fasciola hepatica. Risk PloS One. 2011;6(1):e16126.
- Bargues MD, Mas-Coma S. Reviewing lymnaeid vectors of fascioliasis by ribosomal DNA sequence analyses. J Helminthol. 2005;79(3):257–267.
- Dung BT, Doanh PN, The DT, et al. Morphological and molecular characterization of lymnaeid snails and their potential role in transmission of Fasciola spp. in Vietnam. Korean J Parasitol. 2013;51(6):657–662.
- Kaset C, Eursitthichai V, Vichasri-Grams S, et al. Rapid identification of lymnaeid snails and their infection with Fasciola gigantica in Thailand. Exp Parasitol. 2010;126(4):482–488.
- Correa AC, Escobar JS, et al. Bridging gaps in the molecular phyologeny of the lymnaeidae (gastropoda: pulmonata), vectors of fascioliasis. BMC Evol Biol. 2010;10:381. doi: 10.1186/1471-2148-10-381
- Dar Y, Djuikwo TF, Vignoles P, et al. Radix natalensis (Gastropoda: lymnaeidae), a potential intermediate host of Fasciola hepatica in Egypt. Parasite. 2010;17(3):251–256.
- Kalbe M, Haberl B, Haas W. Snail host finding by Fasciola hepatica and Trichobilharzia ocellata: compound analysis of “miracidia-attracting glycoproteins. Exp Parasitol. 2000;96(4):231–242.
- Di Maggio LS, Tirloni L, Pinto AFM, et al. A proteomic comparison of excretion/secretion products in Fasciola hepatica newly excysted juveniles (NEJ) derived from Lymnaea viatrix or Pseudosuccinea columella. Exp Parasitol. 2019;201:11–20.
- Kaplan RM. Fasciola hepatica: a review of the economic impact in cattle and considerations for control. Vet Ther. 2001;2(1):40–50.
- Rondelaud D. Fasciola hepatica: the infection rate and the development of redial generations in Lymnaea truncatula exposed to miracidia after experimental desiccation and activation in water. J Helminthol. 1994;68(1):63–66.
- Dar Y, Vignoles P, Rondelaud D, et al. Radix natalensis: the effect of Fasciola hepatica infection on the reproductive activity of the snail. Parasite. 2014;21:24.
- Vignoles P, Dreyfuss G, Rondelaud D. Fasciola hepatica: comparative metacercarial productions in experimentally-infected Galba truncatula and Pseudosuccinea columella. Parasite. 2015;22:15.
- Kendall SB. Relationships between the species of Fasciola and their molluscan hosts. Adv Parasitol. 1965;3:59–98.
- Bargues MD, Mas-Coma S. Reviewing lymnaeid vectors of fascioliasis by ribosomal DNA sequence analyses. Journal of Helminthology. 2005;79(3):257–267.
- Kendall SB. Fascioliasis in Pakistan. Ann Tropic Med Parasitol. 1954;48:307–313.
- Walker SM, Makundi AE, Namuba FV, et al. The distribution of Fasciola hepatica and Fasciola gigantica within southern tanzania - constraints associated with the intermediate host. Parasitology. 2008;135(4):495–503.
- Dinnik JA, Dinnik NN. The influence of temperature on the succession of redial and cercarial generations of Fasciola giganticain a snail host. Parasitology. 1964;54:59–65.
- Dreyfuss G, Rondelaud D. Fasciola gigantica and F. hepatica: a comparative study of some characteristics of Fasciola infection in Lymnaea truncatula infected by either of the two trematodes. Vet Res. 1997;28::123–130.
- Da Costa C, Dreyfuss G, Rakotondravao RD. Several observations concerning cercarial sheddings of Fasciola gigantica from Lymnaea natalensis. Parasite. 1994;1(1):39–44.
- Kimura S, Shimizu A, Kawano J. Extermination of Fasciola gigantica metacercariae. J Parasitol. 1980;66(4):699–700.
- Boray JC, Enigh K. Laboratory studies on the survival and infectivity of Fasciola hepatica and F. gigantica metacercariae. Zeitschrift fur Tropenmedizin und Parasitologie. 1964;15:324–331.
- Al-Kubaisee RY, Altaif KI. Comparative study of sheep and buffalo isolates of Fasciola gigantica in the intermediate host Lymnaea auricularia. Res Vet Sci. 1989;47(2):273–274.
- Suhardono RJA, Copeman DB. The effect of temperature and humidity on longevity of metacercariae of Fasciola gigantica. Tropical Anim Health Prod. 2006;38(5):371–377.
- Shirai M. The biological observiation of the cysts of Fasciola hepatica and the route of migration of the young worms in the final host. Scientific Reports from the Government Institute for Infectious Diseases. 1927;6:511–523.
- Simcock E. Liver fluke control in sheep 2019. Available from: https://www.nadis.org.uk/disease-a-z/sheep/liver-fluke-control-in-sheep/. Accessed in June 2021.
- Ballweber LR. Fasciola hepatica in ruminants 2014.
- Kozat S, Denizhan V. Glucose, lipid, and lipoprotein levels in sheep naturally infected with Fasciola hepatica. J Parasitol. 2010;96(3):657–659.
- Hodzic A, Zuko A, Avdic R, et al. Influence of Fasciola hepatica on serum biochemical parameters and vascular and biliary system of sheep Liver. Iran J Parasitol. 2013;8(1):92–98. PMC3655246.
- Chauvin A, Moreau E, Boulard C. Responses of Fasciola hepatica infected sheep to various infection levels. Vet Res. 2001;32(1):87–92.
- Williams DJL, Howell A, Graham-Brown J, et al. Liver fluke an overview for practitioner. 2014. Available in: https://www.cattleparasites.org.uk/app/uploads/2018/04/Liver-fluke-an-overview-for-practitioners.pdf. Accessed in: June 2021.
- Sharma RL, Godara R, Thilagar MB. Epizootiology, pathogenesis and immunoprophylactic trends to control tropical bubaline fasciolosis: an overview. J Parasitic Dis. 2011;35(1):1–9.
- Scott P. Liver Fluke control in cattle 2016. Available from: https://www.nadis.org.uk/disease-a-z/cattle/liver-fluke-control-in-cattle/. Accessed in: June 2021.
- Marcos LA, Yi P, Machicado A, et al. Hepatic fibrosis and Fasciola hepatica infection in cattle. J Helminthol. 2007;81(4):381–386.
- Da Costa RA, Corbellini LG, Castro-Janer E, et al. Evaluation of losses in carcasses of cattle naturally infected with Fasciola hepatica: effects on weight by age range and on carcass quality parameters. Int J Parasitol. 2019;49(11):867–872.
- Molento MB, Dutra LH, Pritsch IC, et al. Fasciola hepatica infection in cattle and the use of simulation models for endemic areas. J Helminthol. 2020;94:e185.
- Hayward AD, Skuce PJ, McNeilly TN. Tolerance of liver fluke infection varies between breeds and producers in beef cattle. Animal. 2021;15(2):100126.
- Howell A, Baylis M, Smith R, et al. Epidemiology and impact of Fasciola hepatica exposure in high-yielding dairy herds. Prev Vet Med. 2015;121(1–2):41–48.
- Board AaHD. Milk farmgate and dairy league - Farmers weekly, farmers weekly. 2021. Available from: https://www.fwi.co.uk/prices-trends/dairy-prices/milk-farmgate-and-dairy-league. Accessed in: June 2021.
- May K, Bohlsen E, Konig S, et al. Fasciola hepatica seroprevalence in Northern German dairy herds and associations with milk production parameters and milk ketone bodies. Vet Parasitol. 2020;277:109016.
- May K, Brugemann K, Konig S, et al. Patent infections with Fasciola hepatica and paramphistomes (Calicophoron daubneyi) in dairy cows and association of fasciolosis with individual milk production and fertility parameters. Vet Parasitol. 2019;267:32–41.
- Lotfollahzadeh S, Mohri M, Sh R B, et al. The relationship between normocytic, hypochromic anaemia and iron concentration together with hepatic enzyme activities in cattle infected with Fasciola hepatica. J Helminthol. 2008;82(1):85–88.
- Coppo J, Mussart N, Zeinsteger P. Hematological indicators of liver damage during the subclinical phase of fasciolosis in steers from Northeastern Argentina. Comp Clini Pathol. 2010;20(4):397–401.
- Mezo M, Gonzalez-Warleta M, Carro C, et al. An ultrasensitive capture ELISA for detection of Fasciola hepatica coproantigens in sheep and cattle using a new monoclonal antibody (MM3). J Parasitol. 2004;90(4):845–852.
- George SD, George AJ, Rolfe PF, et al. Comparative assessment of faecal diagnostics for detection and predictive modelling of endemic Fasciola hepatica infection in sheep and cattle on Australian farms. Vet Parasitol X. 2019;1:100001.
- George SD, Vanhoff K, Baker K, et al. Application of a coproantigen ELISA as an indicator of efficacy against multiple life stages of Fasciola hepatica infections in sheep. Vet Parasitol. 2017;246:60–69.
- Martinez-Sernandez V, Perteguer MJ, Hernandez-Gonzalez A, et al. Comparison of recombinant cathepsins L1, L2, and L5 as ELISA targets for serodiagnosis of bovine and ovine fascioliasis. Parasitol Res. 2018;117(5):1521–1534.
- López Corrales J, Cwiklinski K, De Marco Verissimo C, et al. Diagnosis of sheep fasciolosis caused by Fasciola hepatica using cathepsin L enzyme-linked immunosorbent assays (ELISA). Vet Parasitol. 2021;298:109517.
- Edith R, Godara R, Sharma RL, et al. Serum enzyme and hematological profile of Fasciola gigantica immunized and experimentally infected riverine buffaloes. Parasitol Res. 2010;106(4):947–956.
- Calvani NED, Ichikawa-Seki M, Bush RD, et al. Which species is in the faeces at a time of global livestock movements: single nucleotide polymorphism genotyping assays for the differentiation of Fasciola spp. Int J Parasitol. 2020;50(2):91–101.
- Happich FA, Boray JC. Quantitative diagnosis of chronic fasciolosis. 1. Comparative studies on quantitative faecal examinations for chronic Fasciola hepatica infection in sheep. Aust Vet J. 1969;45(7):326–332.
- Martinez-Sernandez V, Orbegozo-Medina RA, Gonzalez-Warleta M, et al. Rapid enhanced MM3-COPRO ELISA for detection of Fasciola coproantigens. PLoS Negl Trop Dis. 2016;10(7):e0004872.
- Gonzales Santana B, Dalton JP, Vasquez Camargo F, et al. The diagnosis of human fascioliasis by enzyme-linked immunosorbent assay (ELISA) using recombinant cathepsin L protease. PLoS Negl Trop Dis. 2013;7(9):e2414.
- Cornelissen JB, Gaasenbeek CP, Borgsteede FH, et al. Early immunodiagnosis of fasciolosis in ruminants using recombinant Fasciola hepatica cathepsin L-like protease. Int J Parasitol. 2001;31(7):728–737.
- Mezo M, Gonzalez-Warleta M, Ubeira FM. The use of MM3 monoclonal antibodies for the early immunodiagnosis of ovine fascioliasis. J Parasitol. 2007;93(1):65–72.
- Martinez-Sernandez V, Muino L, Perteguer MJ, et al. Development and evaluation of a new lateral flow immunoassay for serodiagnosis of human fasciolosis. PLoS Negl Trop Dis. 2011;5(11):e1376.
- Calvani NED, Windsor PA, Bush RD, et al. Scrambled eggs: a highly sensitive molecular diagnostic workflow for Fasciola species specific detection from faecal samples. PLoS Negl Trop Dis. 2017;11(9):e0005931.
- Martinez-Valladares M, Rojo-Vazquez FA. Loop-mediated isothermal amplification (LAMP) assay for the diagnosis of fasciolosis in sheep and its application under field conditions. Parasites Vectors. 2016;9(1):73.
- Dittmar K, Teegen WR. The presence of Fasciola hepatica (liver-fluke) in humans and cattle from a 4,500 year old archaeological site in the Saale-Unstrut valley, Germany. Memorias Do Instituto Oswaldo Cruz. 2003;98(Suppl 1):141–143.
- Le Bailly M, Bouchet F. Etude parasitologique des coprolithes humains du site néolithique Arbon-Bleiche 3. Die Jungsteinzeitliche Seeufersiedlung Arbon-Bleiche 3, Umwelt und Wirtschaft. Archäologie im Thurgau. 2004:372–377.
- Grove DIA. History of Human Helminthology. Oxon UK: CAB International Wallingford; 1990. p. 848.
- Hillyer GV, Soler de Galanes M, Rodriguez-Perez J, et al. Use of the Falcon assay screening test--enzyme-linked immunosorbent assay (FAST-ELISA) and the enzyme-linked immunoelectrotransfer blot (EITB) to determine the prevalence of human fascioliasis in the bolivian altiplano. Am J Trop Med Hyg. 1992;46(5):603–609.
- Bjorland J, Bryan RT, Strauss W, et al. An outbreak of acute fascioliasis among aymara Indians in the bolivian altiplano. Clinl Infect Dis. 1995;21(5):1228–1233.
- Mas-Coma S, Valero MA, Bargues MD. Fascioliasis. Adv Exp Med Biol. 2019;1154:71–103.
- Gandhi P, Schmitt EK, Chen CW, et al. Triclabendazole in the treatment of human fascioliasis: a review. Trans R Soc Trop Med Hyg. 2019;113(12):797–804.
- Bui TD, Doanh PN, Saegerman C, et al. Current status of fasciolosis in Vietnam: an update and perspectives. J Helminthol. 2016;90(5):511–522.
- Nguyen THO, Cheong FW, Liew JWK, et al. Seroprevalence of fascioliasis, toxocariasis, strongyloidiasis and cysticercosis in blood samples diagnosed in medic medical center laboratory, ho chi minh city, vietnam in 2012. Parasit Vectors. 2016;9(1):486.
- Esteban JG, Gonzalez C, Curtale F, et al. Hyperendemic fascioliasis associated with schistosomiasis in villages in the nile delta of egypt. Am J Trop Med Hyg. 2003;69(4):429–437.
- Curtale F, Hassanein YAEW, Savioli L. Control of human fascioliasis by selective chemotherapy: design, cost and effect of the first public health, school-based intervention implemented in endemic areas of the nile delta, egypt. Trans R Soc Trop Med Hyg. 2005;99(8):599–609.
- Siles-Lucas M, Becerro-Recio D, Serrat J, et al. Fascioliasis and fasciolopsiasis: current knowledge and future trends. Res Vet Sci. 2021;134:27–35.
- Marcos L, Maco V, Samalvides F, et al. Risk factors for Fasciola hepatica infection in children: a case-control study. Trans R Soc Trop Med Hyg. 2006;100(2):158–166.
- De Bree LC, Bodelier AG, Verburg GP. Fasciola hepatica as a cause of jaundice after chewing khat: a case report. Netherlands J Med. 2013;71(9):478–479.
- Ashrafi K. The status of human and animal fascioliasis in Iran: a narrative review article. Iran J Parasitol. 2015;10(3):306–328.
- Yadegari D, Talaie H, Massoud J. Clinical trail of triclabendazole on human fascioliasis: long term follow up. MJIRI. 1999;13(2):89–91.
- Milas S, Rossi C, Philippart I, et al. Autochthonous human fascioliasis, Belgium. Emerg Infect Dis. 2020;26(1):155–157.
- Sampaio-Silva ML, Da Costa JM, Da Costa AM, et al. Antigenic components of excretory-secretory products of adult Fasciola hepatica recognized in human infections. Am J Trop Med Hyg. 1996;54(2):146–148.
- Arjona R, Riancho JA, Aguado JM, et al. Fascioliasis in developed countries: a review of classic and aberrant forms of the disease. Medicine (Baltimore). 1995;74(1):13–23.
- Marcos L, Maco V, Terashima A, et al. Fascioliasis in relatives of patients with Fasciola hepatica infection in Peru. Rev Inst Med Trop São Paulo. 2005;47(4):219–222.
- Parkinson M, O’Neill SM, Dalton JP. Endemic human fasciolosis in the bolivian altiplano. Epidemiol Infect. 2007;135(4):669–674.
- Curtale F, Hassanein YA, Barduagni P, et al. Human fascioliasis infection: gender differences within school-age children from endemic areas of the nile delta, egypt. Trans R Soc Trop Med Hyg. 2007;101(2):155–160.
- Harrington D, Lamberton PHL, McGregor A. Human liver flukes. Lancet Gastroenterol Hepatol. 2017;2(9):680–689.
- Dawes B. The migration of juvenile forms of Fasciola hepatica L. through the wall of the intestines in the mouse, with some observations on food and feeding. Parasitology. 1963;53(1–2):109–122.
- Ross JG. An abattoir survey of cattle liver infections with Fasciola hepatica. Br Vet J. 1966;122(11):489–494.
- Torres GB, Iwashita AT, Vargas CM, et al. Human fasciolasis and gastrointestinal compromise: study of 277 patients in the Cayetano Heredia National Hospital (1970-2002). Revista de Gastroenterología del Perú. 2004;24(2):143–157.
- Krsak M, Patel NU, Poeschla EM. Case Report: hepatic fascioliasis in a young Afghani woman with severe wheezing, high-grade peripheral eosinophilia, and liver lesions: A brief literature review. Am J Trop Med Hyg. 2019;100(3):588–590.
- Chen MG, Mott KE. Progress in assessment of morbidity due to Fasciola hepatica infection: a review of recent literature. Tropical Dis Bull. 1990;87(4):1–38.
- Kaida T, Beppu T, Hayashi H, et al. Inflammatory liver tumor caused by Fasciola hepatica mimicking intrahepatic cholangiocarcinoma. Anticancer Res. 2020;40(5):2795–2800.
- Aksoy DY, Kerimoglu U, Oto A, et al. Infection with Fasciola hepatica. Clin Microbiol Infect. 2005;11(11):859–861.
- Taghipour A, Zaki L, Rostami A, et al. Highlights of human ectopic fascioliasis: a systematic review. Infectious Diseases. 2019;51(11–12):785–792.
- Micic D, Oto A, Charlton MR, et al. Hiding in the Water. N Engl J Med. 2020;382(19):1844–1849.
- Perrodin S, Walti L, Gottstein B, et al. Fasciola hepatica in a country of low incidence: a tricky diagnosis. Hepatobiliary Surg Nutr. 2019;8(6):597–603.
- Temido H, Oliveira-Santos M, Parente F, et al. Fascioliasis-a rare cause of hepatic nodules. BMJ Case Rep. 2017. doi: 10.1136/bcr-2017-220363. PMCID: PMC5747644
- Preza O, Klapa I, Tsiakalos A, et al. Fascioliasis: a challenging differential diagnosis for radiologists. J Radiol Case Rep. 2019;13(1):11–16.
- Marcos LA, Tagle M, Terashima A, et al. Natural history, clinicoradiologic correlates, and response to triclabendazole in acute massive fascioliasis. Am J Trop Med Hyg. 2008;78(2):222–227.
- Kwok J, Buxbaum JL. Liver Fluke. N Engl J Med. 2019;381(19):e34.
- Shin SH, Hsu A, Chastain HM, et al. Development of two FhSAP2 recombinant-based assays for immunodiagnosis of human chronic fascioliasis. Am J Trop Med Hyg. 2016;95(4):852–855.
- O’Neill SM, Parkinson M, Dowd AJ, et al. Short report: immunodiagnosis of human fascioliasis using recombinant Fasciola hepatica cathepsin L1 cysteine proteinase. Am J Trop Med Hyg. 1999;60(5):749–751.
- Keiser J, Utzinger J. Chemotherapy for major food-borne trematodes: a review. Expert Opin Pharmacother. 2004;5(8):1711–1726.
- WHO. Report of the WHO informal meeting on use of triclabendazole in fascioliasis control 2007. Available from: https://www.who.int/neglected_diseases/preventive_chemotherapy/WHO_CDS_NTD_PCT_2007.1.pdf?ua=1. Accessed in: June 2021.
- Terashima A, Canales M, Maco V, et al. Observational study on the effectiveness and safety of multiple regimens of triclabendazole in human fascioliasis after failure to standard-of-care regimens. Journal of Global Antimicrobial Resistance. 2021;25:264–267.
- Corrales JL, Cwiklinski K, De Marco Verissimo C, et al. Diagnosis of sheep fasciolosis caused by Fasciola hepatica using cathepsin L enzyme-linked immunosorbent assays (ELISA). Vet Parasitol. 2021;298:109517.
- Andrews S. The life cycle of Fasciola hepatica. Fasciolosis. 3 ed.; Wallingford: CABI Publishing. Editor: Dalton JP. 1999. p. 1–29.
- Dixon KE. The structure and histochemistry of the cyst wall of the metacercaria of Fasciola hepatica. L Parasitol. 1965;55:215–226.
- Valero MA, Mas-Coma S. Comparative infectivity of Fasciola hepatica metacercariae from isolates of the main and secondary reservoir animal host species in the bolivian altiplano high human endemic region. Folia Parasitol (Praha). 2000;47(1):17–22.
- Mas-Coma S, Buchon P, Funatsu IR, et al. Donkey fascioliasis within a One Health control action: transmission capacity, field epidemiology, and reservoir role in a human hyperendemic area. Front Vet Sci. 2020;7:591384.
- Dawes B. On the early stages of Fasciola hepatica penetrating into the liver of an experimental host, the mouse: a histological picture. J Helminthol. 1961;35(S1):41–52.
- Burden DJ, Bland AP, Hughes DL, et al. Fasciola hepatica: a technique for the study of gut penetration by juvenile flukes. Parasitology. 1981;83(Pt 2):249–252.
- Tielens AGM. Metabolism. Fasciolosis. 3 ed.; CABI Publishing. Editor: Dalton JP. 1999. p. 277–305.
- Sethadavit M, Meemon K, Jardim A, et al. Identification, expression and immunolocalization of cathepsin B3, a stage-specific antigen expressed by juvenile Fasciola gigantica. Acta Trop. 2009;112(2):164–173.
- Sansri V, Changklungmoa N, Chaichanasak P, et al. Molecular cloning, characterization and functional analysis of a novel juvenile-specific cathepsin L of Fasciola gigantica. Acta Trop. 2013;128(1):76–84.
- Gonzalez-Miguel J, Becerro-Recio D, Siles-Lucas M. Insights into Fasciola hepatica juveniles: crossing the fasciolosis rubicon. Trends Parasitol. 2021;37(1):35–47.
- Dalton JP, Brindley PJ, Donnelly S, et al. The enigmatic asparaginyl endopeptidase of helminth parasites. Trends Parasitol. 2009;25(2):59–61.
- Berasain P, Goni F, McGonigle S, et al. Proteinases secreted by Fasciola hepatica degrade extracellular matrix and basement membrane components. J Parasitol. 1997;83(1):1–5.
- Robinson MW, Corvo I, Jones PM, et al. Collagenolytic activities of the major secreted cathepsin L peptidases involved in the virulence of the helminth pathogen, Fasciola hepatica. PLoS Negl Trop Dis. 2011;5(4):e1012.
- Caffrey CR, Goupil L, Rebello KM, et al. Cysteine proteases as digestive enzymes in parasitic helminths. PLoS Negl Trop Dis. 2018;12(8):e0005840.
- McGonigle L, Mousley A, Marks NJ, et al. The silencing of cysteine proteases in Fasciola hepatica newly excysted juveniles using RNA interference reduces gut penetration. Int J Parasitol. 2008;38(2):149–155.
- Beckham SA, Piedrafita D, Phillips CI, et al. A major cathepsin B protease from the liver fluke Fasciola hepatica has atypical active site features and a potential role in the digestive tract of newly excysted juvenile parasites. Int J Biochem Cell Biol. 2009;41(7):1601–1612.
- Garcia-Campos A, Ravida A, Nguyen DL, et al. Tegument glycoproteins and cathepsins of newly excysted juvenile Fasciola hepatica carry mannosidic and paucimannosidic N-glycans. PLoS Negl Trop Dis. 2016;10(5):e0004688.
- Garcia-Campos A, Baird AW, Mulcahy G. Migration of Fasciola hepatica newly excysted juveniles is inhibited by high-mannose and oligomannose-type N-glycan-binding lectins. Parasitology. 2017;144(13):1708–1717.
- Kendall SB, Parfitt JW. The chemotherapy of fascioliasis. Br Vet J. 1962;118(1):1–10.
- Nansen P, Andersen S, Harmer E, et al. Experimental fascioliasis in the pig. Exp Parasitol. 1972;31(2):247–254.
- Burden DJ, Bland AP, Hammet NC, et al. Fasciola hepatica: migration of newly excysted juveniles in resistant rats. Exp Parasitol. 1983;56(2):277–288.
- Dalton JP, Robinson MW, Mulcahy G, et al. Immunomodulatory molecules of Fasciola hepatica: candidates for both vaccine and immunotherapeutic development. Vet Parasitol. 2013;195(3–4):272–285.
- Hu H, Sun SC. Ubiquitin signaling in immune responses. Cell Res. 2016;26(4):457–483.
- Lammas DA, Duffus WP. The shedding of the outer glycocalyx of juvenile Fasciola hepatica. Vet Parasitol. 1983;12(2):165–178.
- Murphy A, Cwiklinski K, Lalor R, et al. Fasciola hepatica extravellular Vesicles isolated from excretory-secretory products using a gravity flow method modulate dendritic cell phenotype and activity. PLoS Negl Trop Dis. 2020;14(9):e0008626.
- Marcilla A, Trelis M, Cortes A, et al. Extracellular vesicles from parasitic helminths contain specific excretory/secretory proteins and are internalized in intestinal host cells. PloS One. 2012;7(9):e45974.
- Tran N, Ricafrente A, To J, et al. Fasciola hepaticahijacks host macrophage miRNA machinery to modulate early innate immune responses. Sci Rep. 2021;11(1):6712.
- Molina-Hernandez V, Mulcahy G, Perez J, et al. Fasciola hepatica vaccine: we may not be there yet but we’re on the right road. Vet Parasitol. 2015;208(1–2):101–111.
- Dorey A, Cwiklinski K, Rooney J, et al. Autonomous non antioxidant roles for Fasciola hepatica secreted Thioredoxin-1 and Peroxiredoxin-1. Front Cell Infect Microbiol. 2021;11(396). DOI:10.3389/fcimb.2021.667272
- Ruiz-Campillo MT, Molina-Hernández V, Pérez J, et al. Study of peritoneal macrophage immunophenotype in sheep experimentally infected with Fasciola hepatica. Vet Parasitol. 2018;257:34–39.
- Donnelly S, O’Neill SM, Sekiya M, et al. Thioredoxin peroxidase secreted by Fasciola hepatica induces the alternative activation of macrophages. Infect Immun. 2005;73(1):166–173.
- Walsh KP, Brady MT, Finlay CM, et al. Infection with a helminth parasite attenuates autoimmunity through TGF-beta-mediated suppression of Th17 and Th1 responses. J Iimmunol. 2009;183(3):1577–1586.
- Donnelly S, Stack CM, O’Neill SM, et al. Helminth 2-Cys peroxiredoxin drives Th2 responses through a mechanism involving alternatively activated macrophages. FASEB J. 2008;22(11):4022–4032.
- Reddington JJ, Leid RW, Wescott RB. Effect of inoculum on the size and location of Fasciola hepatica subsequently recovered from the livers of rats. Vet Parasitol. 1984;14(1):13–19.
- Presidente PJ, McCraw BM, Lumsden JH. Early pathological changes associated with Fasciola hepatica infections in white-tailed deer. Can J Comp Med Vet Sci. 1974;38(3):271–279. PMC1319868.
- Valero MA, De Renzi M, Panova M, et al. Crowding effect on adult growth, pre-patent period and egg shedding of Fasciola hepatica. Parasitology 2006;133((Pt 4)):453–463.
- Sangster NC, Martínez-Moreno A, Pérez J. Pathology, pathophysiology and clinical aspects. Fasciolosis, V2. Wallingford: CABI Publishing. Editor: Dalton JP; 2021. p145-179.
- Ruiz-Campillo MT, Molina Hernandez V, Escamilla A, et al. Immune signatures of pathogenesis in the peritoneal compartment during early infection of sheep with Fasciola hepatica. Sci Rep. 2017;7(1):2782.
- Cwiklinski K, Robinson MW, Donnelly S, et al. Complementary transcriptomic and proteomic analyses reveal the cellular and molecular processes that drive growth and development of Fasciola hepatica in the host liver. BMC Genomics. 2021;22(1):46.
- Howell RM. Collagenase activity of immature Fasciola hepatica. Nature. 1966;209(5024):713–724.
- McVeigh P, Maule AG, Dalton JP, et al. Fasciola hepatica virulence-associated cysteine peptidases: a systems biology perspective. Microbes Infect. 2012;14(4):301–310.
- Robinson MW, Donnelly S, Hutchinson AT, et al. A family of helminth molecules that modulate innate cell responses via molecular mimicry of host antimicrobial peptides. PLoS Pathog. 2011;7(5):e1002042.
- McCusker P, McVeigh P, Rathinasamy V, et al. Stimulating neoblast-like cell proliferation in juvenile Fasciola hepatica supports growth and progression towards the adult phenotype in vitro. PLoS Negl Trop Dis. 2016;10(9):e0004994.
- Tanaka M, Miyajima A. Liver regeneration and fibrosis after inflammation. Inflamm Regen. 2016;36:19.
- Robinson MW, Harmon C, O’Farrelly C. Liver immunology and its role in inflammation and homeostasis. Cell Mol Immunol. 2016;13(3):267–276.
- Escamilla A, Zafra R, Perez J, et al. Distribution of Foxp3(+) T cells in the liver and hepatic lymph nodes of goats and sheep experimentally infected with Fasciola hepatica. Vet Parasitol. 2016;230:14–19.
- Pacheco IL, Abril N, Zafra R, et al. Fasciola hepatica induces Foxp3 T cell, proinflammatory and regulatory cytokine overexpression in liver from infected sheep during early stages of infection. Vet Res. 2018;49(1):56.
- Valero MA, Perez-Crespo I, Chillon-Marinas C, et al. Fasciola hepatica reinfection potentiates a mixed Th1/Th2/Th17/Treg response and correlates with the clinical phenotypes of anemia. PloS One. 2017;12(3):e0173456.
- Tliba O, Sibille P, Boulard C, et al. Local hepatic immune response in rats during primary infection with Fasciola hepatica. Parasite. 2000;7(1):9–18.
- Chapman CB, Mitchell GF. Proteolytic cleavage of immunoglobulin by enzymes released by Fasciola hepatica. Vet Parasitol. 1982;11(2–3):165–178.
- Carmona C, Dowd AJ, Smith AM, et al. Cathepsin L proteinase secreted by Fasciola hepatica in vitro prevents antibody-mediated eosinophil attachment to newly excysted juveniles. Mol Biochem Parasitol. 1993;62(1):9–17.
- Piedrafita D, Parsons JC, Sandeman RM, et al. Antibody-dependent cell-mediated cytotoxicity to newly excysted juvenile Fasciola hepatica in vitro is mediated by reactive nitrogen intermediates. Parasite Immunol. 2001;23(9):473–482.
- Donnelly S, O’Neill SM, Stack CM, et al. Helminth cysteine proteases inhibit TRIF-dependent activation of macrophages via degradation of TLR3. J Biol Chem. 2010;285(5):3383–3392.
- Falcon CR, Masih D, Gatti G, et al. Fasciola hepatica Kunitz type molecule decreases dendritic cell activation and their ability to induce inflammatory responses. PLoS One. 2014;9(12):e114505.
- Robinson MW, Alvarado R, To J, et al. A helminth cathelicidin-like protein suppresses antigen processing and presentation in macrophages via inhibition of lysosomal vATPase. FASEB J. 2012;26(11):4614–4627.
- Alvarado R, To J, Lund ME, et al. The immune modulatory peptide FhHDM-1 secreted by the helminth Fasciola hepatica prevents NLRP3 inflammasome activation by inhibiting endolysosomal acidification in macrophages. FASEB J. 2017;31(1):85–95.
- Figueroa-Santiago O, Espino AM, Appleton JA. Fasciola hepatica fatty acid binding protein induces the alternative activation of human macrophages. Infect Immun. 2014;82(12):5005–5012.
- Ruiz-Jiménez C, Espino AM. Interaction of Fasciola hepatica Fatty Acid Binding Protein with TLR-2: a preliminary study to understand the immunomodulation mechanisms that exert F. hepatica on the immune system (LB287). FASEB J. 2014;28(S1):LB287.
- Martin I, Cabán-Hernández K, Figueroa-Santiago O, et al. Fasciola hepatica fatty acid binding protein inhibits TLR4 activation and suppresses the inflammatory cytokines induced by lipopolysaccharide in vitro and in vivo. J Immunol. 2015;194(8):3924–3936.
- Ramos-Benitez MJ, Ruiz-Jimenez C, Aguayo V, et al. Recombinant Fasciola hepatica fatty acid binding protein suppresses toll-like receptor stimulation in response to multiple bacterial ligands. Sci Rep. 2017;7(1):5455.
- Rodríguez E, Kalay H, Noya V, et al. Fasciola hepatica glycoconjugates immuneregulate dendritic cells through the dendritic cell-specific intercellular adhesion molecule-3-Grabbing non-integrin inducing T cell anergy. Sci Rep. 2017;7(1):46748.
- Frigerio S, Da Costa V, Costa M, et al. Eosinophils control liver damage by modulating immune responses against Fasciola hepatica. Front Immunol. 2020;11:579801.
- Escamilla A, Bautista MJ, Zafra R, et al. Fasciola hepatica induces eosinophil apoptosis in the migratory and biliary stages of infection in sheep. Vet Parasitol. 2016;216:84–88.
- Serradell MC, Guasconi L, Cervi L, et al. Excretory-secretory products from Fasciola hepatica induce eosinophil apoptosis by a caspase-dependent mechanism. Vet Immunol Immunopathol. 2007;117(3–4):197–208.
- Guasconi L, Serradell MC, Masih DT. Fasciola hepatica products induce apoptosis of peritoneal macrophages. Vet Immunol Immunopathol. 2012;148(3–4):359–363.
- Escamilla A, Perez-Caballero R, Zafra R, et al. Apoptosis of peritoneal leucocytes during early stages of Fasciola hepatica infections in sheep. Vet Parasitol. 2017;238:49–53.
- Fu Y, Chryssafidis AL, Browne JA, et al. Transcriptomic study on ovine immune responses to Fasciola hepaticainfection. PLoS Negl Trop Dis. 2016;10(9):e0005015.
- Garcia-Campos A, Correia CN, Naranjo-Lucena A, et al. Fasciola hepatica infection in cattle: analyzing responses of peripheral blood mononuclear cells (PBMC) using a transcriptomics approach. Front Immunol. 2019;10:2081.
- Ricafrente A, Nguyen H, Tran N, et al. An evaluation of the Fasciola hepatica miRnome predicts a targeted regulation of mammalian innate immune responses. Front Immunol. 2020;11:608686.
- Gajewska A, Smaga-Kozłowska K, Kotomski G. Effect of excretory-secretory products of Fasciola hepatica on the functioning of rat hepatocytes. Medycyna Weterynaryjna-Vet Med Sci Pract. 2006;62(4):459–462.
- Wesolowska A, Gajewska A, Smaga-Kozlowska K, et al. Effect of Fasciola hepatica proteins on the functioning of rat hepatocytes. Parasitol Res. 2012;110(1):395–402.
- Baska P, Norbury LJ, Wisniewski M, et al. Excretory/secretory products of Fasciola hepatica but not recombinant phosphoglycerate kinase induce death of human hepatocyte cells. Acta Parasitol. 2013;58(2):215–217.
- Saleh MA. Circulating oxidative stress status in desert sheep naturally infected with Fasciola hepatica. Vet Parasitol. 2008;154(3–4):262–269.
- Bottari NB, Mendes RE, Lucca NJ, et al. Oxidative stress associated with pathological lesions in the liver of rats experimentally infected by Fasciola hepatica. Exp Parasitol. 2015;159:24–28.
- Da Silva AS, Baldissera MD, Bottari NB, et al. Oxidative stress and changes in adenosine deaminase activity of cattle experimentally infected by Fasciola hepatica. Parasitology 2017;144(4):520–526.
- Rehim WM, Sharaf IA, Hishmat M, et al. Antioxidant capacity in Fasciola hepatica patients before and after treatment with triclabendazole alone or in combination with ascorbic acid (vitamin C) and tocofersolan (vitamin E). Arzneimittel-Forschung. 2003;53(3):214–220.
- Kolodziejczyk L, Siemieniuk E, Skrzydlewska E. Fasciola hepatica: effects on the antioxidative properties and lipid peroxidation of rat serum. Exp Parasitol. 2006;113(1):43–48.
- Kaya S, Sutcu R, Cetin ES, et al. Lipid peroxidation level and antioxidant enzyme activities in the blood of patients with acute and chronic fascioliasis. Inter J Infect Dis. 2007;11(3):251–255.
- Bahrami S, Esmaeilzadeh S, Oryan A. Role of oxidative stress in concomitant occurrence of Fasciola gigantica and leiomyoma in cattle. Vet Parasitol. 2014;203(1–2):43–50.
- Isseroff H, Tunis M, Read CP. Changes in amino acids of bile in Fasciola hepatica infections. Comparative Biochem Physiol B. 1972;41(1):157–163.
- Ertel J, Isseroff H. Proline in fascioliasis: i. Comparative activities of ornithine-delta-transaminase and proline oxidase in Fasciola and in mammalian livers. J Parasitol. 1974;60(4):574–577.
- Isseroff H, Sawma JT, Reino D. Fascioliasis: role of proline in bile duct hyperplasia. Science. 1977;198(4322):1157–1159.
- Wolf-Spengler ML, Isseroff H. Fascioliasis: bile duct collagen induced by proline from the worm. J Parasitol. 1983;69(2):290–294.
- Szabados L, Savoure A. Proline: a multifunctional amino acid. Trends Plant Sci. 2010;15(2):89–97.
- Liang X, Zhang L, Natarajan SK, et al. Proline mechanisms of stress survival. Antioxid Redox Signal. 2013;19(9):998–1011.
- Ishikawa T, Meier-Stephenson V, Heitman SJ. Biliary obstruction caused by the liver fluke, Fasciola hepatica. CMAJ. 2016;188(7):524–526.
- Sinclair KB. Pathogenesis of Fasciola and other liver-flukes. Helminthol Abstracts. 1967;36:115–134.
- Modavi S, Isseroff H. Fasciola hepatica: collagen deposition and other histopathology in the rat host’s bile duct caused by the parasite and by proline infusion. Exp Parasitol. 1984;58(3):239–244.
- Dawes B. Some observations of Fasciola hepatica L during feeding operations in the hepatic parenchyma of the mouse, with notes on the nature of liver damage in this host. Parasitology. 1963;53(1–2):135–143.
- Lopez P, Tuñon MJ, Gonzalez P, et al. Ductular proliferation and hepatic secretory function in experimental fascioliasis. Exp Parasitol. 1993;77(1):36–42.
- Boyer JL. Bile formation and secretion. Compr Physiol. 2013;3(3):1035–1078.
- Miller CM, Howell MJ, Boray JC. Glutathione S-transferases as markers of salicylanilide resistance in isolates of Fasciola hepatica. Int J Parasitol. 1994;24(4):533–542.
- Fernandez V, Estein S, Ortiz P, et al. A single amino acid substitution in isozyme GST mu in triclabendazole resistant Fasciola hepatica (Sligo strain) can substantially influence the manifestation of anthelmintic resistance. Exp Parasitol. 2015;159:274–279.
- Kiger L, Rashid AK, Griffon N, et al. Trematode hemoglobins show exceptionally high oxygen affinity. Biophys J. 1998;75(2):990–998.
- Young ND, Nagarajan N, Lin SJ, et al. The Opisthorchis viverrini genome provides insights into life in the bile duct. Nat Commun. 2014;5(1):4378.
- Valero MA, Santana M, Morales M, et al. Risk of gallstone disease in advanced chronic phase of fascioliasis: an experimental study in a rat model. J Infect Dis. 2003;188(5):787–793.
- Mas-Coma S, Esteban MS, Bargues MD. Epidemiology of human fascioliasis: a review and proposed new classification. Bull World Health Organ. 1999;77(4):340–346.
- Mas-Coma S, Bargues MD, Marty AM, et al. Hepatic trematodiases. In: Meyers WM, Neafie RC, Marty AM, et al., editors. Pathology of infectious diseases, helminthiases. 1. Washington DC: Armed Forces Institute of Pathology and American Registry of Pathology; 2000. p. 69–92.
- Sotillo J, Toledo R, Mulvenna J, et al. Exploiting helminth-host interactomes through Big Data. Trends Parasitol. 2017;33(11):875–888.
- Dowd AJ, McGonigle S, Dalton JP. Fasciola hepatica cathepsin L proteinase cleaves fibrinogen and produces a novel type of fibrin clot. Eur J Biochem. 1995;232(1):241–246.
- Dalton JP, Neill SO, Stack C, et al. Fasciola hepatica cathepsin L-like proteases: biology, function, and potential in the development of first generation liver fluke vaccines. Int J Parasitol. 2003;33(11):1173–1181.
- Ryan S, Shiels J, Taggart CC, et al. Fasciola hepatica-derived molecules as regulators of the host immune response. Front Immunol. 2020;11:2182.
- Ticho AL, Malhotra P, Dudeja PK, et al. Intestinal absorption of bile acids in health and disease. Compr Physiol. 2019;10(1):21–56.
- Morphew RM, Wright HA, LaCourse EJ, et al. Comparative proteomics of excretory-secretory proteins released by the liver fluke Fasciola hepatica in sheep host bile and during in vitro culture ex host. Mol Cell Proteomics. 2007;6(6):963–972.
- Ferre I, Ortega-Mora LM, Rojo-Vazquez FA. Serum and bile antibody responses (IgG and IgA) during subclinical Fasciola hepatica infection in sheep. Vet Parasitol. 1997;68(3):261–267.
- Munita MP, Rea R, Martinez-Ibeas AM, et al. Liver fluke in Irish sheep: prevalence and associations with management practices and co-infection with rumen fluke. Parasit Vectors. 2019;12(1):525.
- Martinez-Sernandez V, Mezo M, Gonzalez-Warleta M, et al. The MF6p/FhHDM-1 major antigen secreted by the trematode parasite Fasciola hepatica is a heme-binding protein. J Biol Chem. 2014;289(3):1441–1456.
- Acosta D, Goni F, Carmona C. Characterization and partial purification of a leucine aminopeptidase from Fasciola hepatica. J Parasitol. 1998;84(1):1–7.
- Espino AM, Hillyer GV. Molecular cloning of a member of the Fasciola hepatica saposin-like protein family. J Parasitol. 2003;89(3):545–552.
- Acosta D, Cancela M, Piacenza L, et al. Fasciola hepatica leucine aminopeptidase, a promising candidate for vaccination against ruminant fasciolosis. Mol Biochem Parasitol. 2008;158(1):52–64.
- Lowther J, Robinson MW, Donnelly SM, et al. The importance of pH in regulating the function of the Fasciola hepatica cathepsin L1 cysteine protease. PLoS Negl Trop Dis. 2009;3(1):e369.
- Toh SQ, Glanfield A, Gobert GN, et al. Heme and blood-feeding parasites: friends or foes? Parasit Vectors. 2010;3:108.
- Dutra FF, Bozza MT. Heme on innate immunity and inflammation. Front Pharmacol. 2014;5:115.
- Martinez-Sernandez V, Perteguer MJ, Mezo M, et al. Fasciola spp: mapping of the MF6 epitope and antigenic analysis of the MF6p/HDM family of heme-binding proteins. PloS One. 2017;12(11):e0188520.
- Kristiansen TZ, Bunkenborg J, Gronborg M, et al. A proteomic analysis of human bile. Mol Cell Proteomics. 2004;3(7):715–728.
- Bernal D, de la Rubia JE, Carrasco-Abad AM, et al. Identification of enolase as a plasminogen-binding protein in excretory-secretory products of Fasciola hepatica. FEBS Lett. 2004;563(1–3):203–206.
- Gomez-Arreaza A, Acosta H, Quinones W, et al. Extracellular functions of glycolytic enzymes of parasites: unpredicted use of ancient proteins. Mol Biochem Parasitol. 2014;193(2):75–81.
- Boukli NM, Delgado B, Ricaurte M, et al. Fasciola hepatica and Schistosoma mansoni: identification of common proteins by comparative proteomic analysis. J Parasitol. 2011;97(5):852–861.
- Gonzalez-Miguel J, Valero MA, Reguera-Gomez M, et al. Numerous Fasciola plasminogen-binding proteins may underlie blood-brain barrier leakage and explain neurological disorder complexity and heterogeneity in the acute and chronic phases of human fascioliasis. Parasitology. 2019;146(3):284–298.
- Calvani NED, Šlapeta J. Fasciola species introgression: just a fluke or something more? Trends Parasitol. 2021;37(1):25–34.
- Agatsuma T, Arakawa Y, Iwagami M, et al. Molecular evidence of natural hybridization between Fasciola hepatica and F. gigantica. Parasitol Int. 2000;49(3):231–238.
- Saijuntha W, Tantrawatpan C, Agatsuma T, et al. Revealing genetic hybridization and DNA recombination of Fasciola hepatica and Fasciola gigantica in nuclear introns of the hybrid fasciola flukes. Mol Biochem Parasitol. 2018;223:31–6. DOI:10.1016/j.molbiopara.2018.06.004.
- Nguyen S, Amer S, Ichikawa M, et al. Molecular identification of Fasciola spp. (Digenea: platyhelminthes) in cattle from Vietnam. Parasite. 2012;19(1):85–89.
- Ichikawa-Seki M, Peng M, Hayashi K, et al. Nuclear and mitochondrial DNA analysis reveals that hybridization between Fasciola hepatica and Fasciola gigantica occurred in China. Parasitology. 2017;144(2):206–213.
- Hanna REB, An JW. In vitro study of the relative importance of bile and carbon dioxide in the activation of Fasciola gigantica metacercariae. Res Vet Sci. 1976;20(3):344–345.
- Hammond JA, Sewell MMH. Experimental infections of cattle with Fasciola gigantica: numbers of parasites recovered after varying periods of infection. Trop Anim Health Prod. 1975;7(2):105–113.
- Molina EC, Skerratt LF. Cellular and humoral responses in liver of cattle and buffaloes infected with a single dose of Fasciola gigantica. Vet Parasitol. 2005;131(1–2):157–163.
- Guralp N, Ozcan C, Simms BT. Fasciola gigantica and fasciolisasis in Turkey. Am J Vet Res. 1964;25(104):196–210.
- Roberts JA, Estuningsih E, Widjayanti S, et al. Resistance of Indonesian Thin Tail sheep against Fasciola gigantica and F. hepatica. Vet Parasitol. 1997;68(1–2):69–78.
- Roberts JA, Estuningsih E, Wiedosari E, et al. Acquisition of resistance against Fasciola gigantica by Indonesian Thin Tail sheep. Vet Parasitol. 1997;73(3–4):215–224.
- Hansen DS, Clery DG, Estuningsih E, et al. Immune responses in Indonesian thin tail and Merino sheep during a primary infection with Fasciola gigantica: lack of a specific IgG2 antibody response is associated with increased resistance to infection in Indonesian sheep. Int J Parasitol. 1999;29(7):1027–1035.
- Raadsma HW, Kingsford NM, Suharyanta STW, et al. Host responses during experimental infection with Fasciola gigantica or Fasciola hepatica in merino sheep I. Comparative immunological and plasma biochemical changes during early infection. Vet Parasitol. 2007;143(3–4):275–286.
- Pleasance J, Raadsma HW, Estuningsih SE, et al. Innate and adaptive resistance of Indonesian Thin Tail sheep to liver fluke: a comparative analysis of Fasciola gigantica and Fasciola hepatica infection. Vet Parasitol. 2011;178(3–4):264–272.
- Pleasance J, Wiedosari E, Raadsma HW, et al. Resistance to liver fluke infection in the natural sheep host is correlated with a type-1 cytokine response. Parasite Immunol. 2011;33(9):495–505.
- Valero MA, Bargues MD, Khoubbane M, et al. Higher physiopathogenicity by Fasciola gigantica than by the genetically close F. hepatica: experimental long-term follow-up of biochemical markers. Trans R Soc Trop Med Hyg. 2016;110(1):55–66#60;italic#62;.#60;/italic#62;
- #60;italic#62;Zhang WY, Moreau E, Hope JC, et al. #60;/italic#62;Fasciola hepatica and Fasciola gigantica: comparison of cellular response to experimental infection in sheep. Exp Parasitol. 2005;111(3):154–159.
- Wiedosari E, Hayakawa H, Copeman B. Host differences in response to trickle infection with Fasciola gigantica in Buffalo, Ongole and Bali calves. Tropical Anim Health Prod. 2006;38:43–53.
- Prasitirat P, Thammasart S, Chompoochan T, et al. The dynamics of antibody titres and faecal egg output in cattle and Buffalo following infection with 500 and 1000 Fasciola gigantica metacercariae. Thai J Vet Med. 1996;26:85–89.
- Molina EC, Gonzaga EA, Sinolinding EO, et al. Differences in susceptibility between cattle and swamp buffaloes to infection with Fasciola gigantica. Trop Anim Health Prod. 2005;37(8):611–616.
- Hu RS, Zhang FK, Elsheikha HM, et al. Proteomic profiling of the liver, hepatic lymph nodes, and spleen of buffaloes infected with Fasciola gigantica. Pathogens 2020;9(12):982.
- Zhang FK, Hu RS, Elsheikha HM, et al. Global serum proteomic changes in water buffaloes infected with Fasciola gigantica. Parasites Vectors. 2019;12(1):281.
- Shi W, Wei ZY, Elsheikha HM, et al. Dynamic expression of cytokine and transcription factor genes during experimental Fasciola gigantica infection in buffaloes. Parasit Vectors. 2017;10(1):602.
- Zhang FK, Guo AJ, Hou JL, et al. Serum levels of cytokines in water buffaloes experimentally infected with Fasciola gigantica. Vet Parasitol. 2017;244:97–101.
- Zhang FK, Hou JL, Guo AJ, et al. Expression profiles of genes involved in TLRs and NLRs signaling pathways of water buffaloes infected with Fasciola gigantica. Mol Immunol. 2018;94:18–26.
- Sheng ZA, Li J, Wang DY, et al. Th2-related cytokines are associated with Fasciola gigantica infection and evasion in the natural host, swamp buffalo. Vet Parasitol. 2019;268:73–80.
- Mei XF, Shi W, Zhang YY, et al. DNA methylation and hydroxymethylation profiles reveal possible role of highly methylated TLR signaling on Fasciola gigantica excretory/secretory products (FgESPs) modulation of Buffalo dendritic cells. Parasit Vectors. 2019;12(1):358.
- Wang SS, Chen D, He JJ, et al. Fasciola gigantica-derived excretory-secretory products alter the expression of mRNAs, miRNAs, lncRNAs, and circRNAs involved in the immune response and metabolism in goat peripheral blood mononuclear cells. Front Immunol. 2021;12:653755.
- Huang SY, Yue DM, Hou JL, et al. Proteomic analysis of Fasciola gigantica excretory and secretory products (FgESPs) interacting with Buffalo serum of different infection periods by shotgun LC-MS/MS. Parasitol Res. 2019;118(2):453–460.
- Hammond JA. Human infection with the liver fluke Fasciola gigantica. Trans R Soc Trop Med Hyg. 1974;68(3):253–254.
- Grange D. Hepatic calcification due to Fasciola gigantica. Arch Surg. 1974;108(1):113–115.
- Itagaki T, Ichinomiya M, Fukuda K, et al. Hybridization experiments indicate incomplete reproductive isolating mechanism between Fasciola hepatica and Fasciola gigantica. Parasitology. 2011;138(10):1278–1284.
- Hayashi K, Ichikawa-Seki M, Mohanta U, et al. Hybrid origin of Asian aspermic Fasciola flukes is confirmed by analyzing two single-copy genes, pepck and pold. J Vet Med Sci. 2018;80(1):98–102.
- Ichikawa-Seki M, Peng MAO, Hayashi KEI, et al. Nuclear and mitochondrial DNA analysis reveals that hybridization between Fasciola hepatica and Fasciola gigantica occurred in China. Parasitology. 2017;144(2):206–213.
- Cwiklinski K, O’Neill SM, Donnelly S, et al. A prospective view of animal and human fasciolosis. Parasite Immunol. 2016;38(9):558–568.