ABSTRACT
PA-X protein arises from a ribosomal frameshift in the PA of influenza A virus (IAV). However, the immune regulatory effect of the PA-X protein of H1N1 viruses on the nasal mucosal system remains unclear. Here, a PA-X deficient H1N1 rPR8 viral strain (rPR8-△PAX) was generated and its pathogenicity was determined. The results showed that PA-X was a pro-virulence factor in mice. Furthermore, it reduced the ability of H1N1 viruses to infect dendritic cells (DCs), the regulator of the mucosal immune system, but not non-immune cells (DF-1 and Calu-3). Following intranasal infection of mice, CCL20, a chemokine that monitors the recruitment of submucosal DCs, was downregulated by PA-X, resulting in an inhibition of the recruitment of CD11b+ DCs to submucosa. It also attenuated the migration of CCR7+ DCs to cervical lymph nodes and inhibited DC maturation with low MHC II and CD40 expression. Moreover, PA-X suppressed the maturation of phenotypic markers (CD80, CD86, CD40, and MHC II) and the levels of secreted pro-inflammatory cytokines (IL-1β, IL-6, and TNF-α) while enhancing endocytosis and levels of anti-inflammatory IL-10 in vitro, suggesting an impaired maturation of DCs that the key step for the activation of downstream immune responses. These findings suggested that the PA-X protein played a critical role in escaping the immune response of nasal mucosal DCs for increasing the virulence of H1N1 viruses.
Introduction
Influenza A virus (IAV) has eight negative-sense RNA segments, which encode at least ten proteins [Citation1], and then several novel viral proteins were found, including PB1-F2, PB1-N40, PA-X, and PB2-S1 [Citation2,Citation3]. Seasonal influenza epidemics are estimated to cause lots of infection deaths annually, with significant social and economic impact [Citation4]. In addition to seasonal outbreaks, influenza pandemics also occur unpredictably at different intervals [Citation4]. Influenza pandemics have occurred every 10–50 y since the 16th century, the worst of which was the 1918 Spanish flu (H1N1 virus) pandemic [Citation5]. Despite the origin of the virus that caused the 1918 pandemic is still debated, all eight RNA segments of the causative virus were derived from avian influenza viruses (AIVs) [Citation6]. The H1N1 influenza virus that caused the 2009 pandemic (pH1N1) is a triple-reassortant virus containing genes from human, swine, and avian viruses that can transmit to humans and pigs [Citation7,Citation8]. Following the initial pandemic, the emerging influenza viruses continue to circulate as IAVs undergo antigenic drifts and antigenic shifts. Given the consequences of the emergence of pandemic strains, enhanced surveillance of IAVs and studies of the mechanisms underlying their pathogenicity are warranted.
The mucosa of the respiratory tract is an important first barrier that protects the host from pathogenic microorganisms and harmful substances [Citation9]. Importantly, mucosal dendritic cells (DCs) play a significant part in monitoring IAV invasion, linking the innate and adaptive immune systems [Citation10]. After DCs take up IAVs from the nasal cavity, they quickly transform from the immature to the mature state and transport IAVs to nearby draining lymph nodes such as cervical lymph nodes (CLNs). They not only present antigens and activate T cells [Citation11] but also amplify the immune response cascade through cytokine networks [Citation12]. The ability of DCs to present viruses increases following the infection of viruses, initiating the immune response that defends against viral infection [Citation13,Citation14]. In early stages of influenza infection, inflammatory DCs can be recruited to the trachea by chemokine CCL2 and activated via SIGN-R1 to recruit natural killer (NK) cells to trachea [Citation15]. On the contrary, human respiratory syncytial virus (RSV) can inhibit dendritic cell maturation, which resulted in the decrease of antigen presentation and T cell activation, and incomplete immunity to RSV reinfections [Citation16]. In addition, the 2009 pH1N1 influenza virus was associated with severe depletion of DC subsets [Citation17]. However, it remains unclear that how intranasally infecting H1N1 viruses regulate the immune function of nasal DCs.
IAVs can easily mutate due to the structural characteristics of their segmented RNA, facilitating immune escape. A new fusion protein named PA-X, which contains 191 amino acids derived from the N-terminus of the PA protein and an open reading frame (X-ORF) formed by a ribosomal frameshift in the PA gene was recently identified [Citation18]. Multiple studies showed that PA-X reduced virulence of H5N1 viruses, which are highly pathogenic (HP) [Citation19]. However, PA-X protein played the contrasting part in low pathogenic (LP) IAVs. Analysis of the H1N1 virus, CA09 (A/California/04/2009), showed that PA-X protein increased the viral replication and pathogenicity in murine lungs [Citation20,Citation21]. PA-X protein also enhanced the pathogenicity of H1N1 strain PR8 (A/Puerto Pico/8/1934) in chicken embryos [Citation22]. In addition, PA-X protein promoted virulence by attenuating the innate immune response induced by H1N2 subtype swine influenza viruses (SIVs) [Citation23]. Regarding the underlying mechanism, PA-X has a global host-shutoff activity that is manifested as a sharp decline in host protein synthesis, but not viral genes, following viral infection [Citation19,Citation24–26]. The gene shutoff by PA-X is mainly associated with inflammation, immune response, and apoptosis [Citation18,Citation19]. PA-X protein enhanced IFN-β mRNA expression and anti-haemagglutinin neutralizing antibodies production in mice infected with H1N1 viruses [Citation20]. Therefore, it is possible that PA-X is an important virulence factor in H1N1 viruses as it regulates host immunity. However, the influence of PA-X protein on the viral pathogenicity and host immunity as well as the detailed mechanisms underlying its function in H1N1 viruses remain unclear.
Here, we constructed H1N1 rPR8 and rPR8-△PAX using reverse genetics technology and used them to evaluate H1N1 pathogenicity in mammals. We also analysed the function of PA-X protein in regulating the nasal mucosal immune system, particularly the crucial component, mucosal DCs. Our findings will help elucidate the infection and immune mechanisms associated with H1N1 viruses.
Materials and methods
Ethics statement
All animal experiments were carried out according to the laboratory animal welfare and ethical guidelines of Jiangsu Administrative Committee for Laboratory Animals, under the authority of Jiangsu Administrative Committee for Laboratory Animals (Permission number: SYXKSU-2017–0044).
Animals
Specific-pathogen-free (SPF) chicken embryos were purchased from Zhejiang Lihua Agricultural Technology Company Limited (Zhejiang, China) and incubated in our laboratory until they were 10 d old. SPF BALB/c and C57BL/6 mice were purchased from Yangzhou University (Center Comparative Medical, Yangzhou, China).
Viruses and cells
H1N1
PR8 strain (A/Puerto Pico/8/1934) [Citation27] was stored in our laboratory. Human embryonic kidney cells (HEK293T), MDCK cells, and Calu-3 cells (alternative models of nasal epithelial cells [Citation28,Citation29]) were cultured in DMEM (Gibco, NY, USA). Douglas Foster-1 (DF-1) cells were cultured in DMEM/F-12 (Gibco, NY, USA). Chicken embryo fibroblast (CEF) cells isolated from embryonated eggs were maintained in Medium 199 (Hyclone, Waltham, USA). All media were supplemented with 10% foetal bovine serum (FBS; Gibco, NY, USA) containing 100 U/mL penicillin and 100 ug/mL streptomycin (Invitrogen, NY, USA). All cells were maintained at 37°C and 5% CO2.
Virus rescue
The gene encoding the PA protein in strain rPR8, X-ORF 5’-UCCUUUCGU-3’, was mutated into 5’-AGUUUCAGA-3’ to develop the knockout PA-X protein following a previously described protocol [Citation18,Citation30]. To obtain rescued viruses based on our established reverse genetics approaches [Citation31], plasmids from the virus were added to dishes plated with HEK293T and MDCK cells and the virus was harvested after most of the cell lesions had fallen off. Mutant viruses were identified using haemagglutinin (HA) titre test, sequencing, and western blotting. Viruses were purified using the density gradient centrifugation as previously reported [Citation32].
Western blotting assay
MDCK cells were infected with rPR8 and rPR8-△PAX strains and cultured. The cells were collected 12 h post infection (h. p. i.), when the multiplicity of infection (MOI) was 10. Proteins were extracted, separated on 12% gels using SDS-PAGE, and transferred onto nitrocellulose membranes (Cytiva, Washington, USA). The blots were blocked using 5% skim milk (Applygen, Beijing, China) and then incubated overnight at 4°C with the following primary antibodies: anti-PA-X (1:1000) (Biorbyt, Cambridge, England), anti-NP (1:3000) (Genetex, Texas, USA), and anti-β-actin (1:10000) (Abcam, Cambridge, England). The membrane was then incubated with horseradish peroxidase (HRP)-conjugated secondary antibodies (1:8000) (Abcam, Cambridge, England). Protein bands were detected using an electrochemiluminescence (ECL) detection system.
Virus replication kinetics
Different cell types, including Calu-3 and DF-1, were incubated at a MOI of 0.01. Virus titres were determined using 50% tissue culture infectious doses (TCID50). CEF cells were infected with supernatants, which were harvested every 12 h for 72 h [Citation31]. HA assay was used to identify influenza virus-positive wells [Citation33]. TCID50 values were calculated using the method of Reed and Muench [Citation34].
Pathogenicity study in mice
Once the rPR8 and rPR8-△PAX infection doses were 101-106 EID50/50 μL, clinical symptoms and body weight changes in six-week-old SPF BALB/c mice were recorded, and survival noted to detect 50% mouse lethal dose (MLD50) (n = 5). The lungs were extracted and fixed with paraformaldehyde for histopathological observation 5 d post-infection (d. p. i.) (n = 3). Sections were randomly numbered (after scrambling the group numbers) and photographed. Each photograph was scored for pathology by two trained, double-blinded pathologists, following a previously described method [Citation35]. For virus titres, grind each sample and take 0.1 mL of supernatant after centrifugation at 6,800 rpm for 10 min [Citation36]. Chicken embryos were inoculated in a 1:10 dilution, with four embryos per concentration, and observed for 72 h. Viral titres were calculated as described previously [Citation34].
In vitro DCs culturing
Murine bone marrow-derived DCs were isolated and cultured as previously described [Citation37]. The femurs and tibias of C57BL/6 mice were aseptically harvested, washed repeatedly until they turned white, and the washing fluid collected. The cells were maintained in complete RPMI 1640 medium containing 10% FBS, 100 U/mL penicillin, 100 µg/mL streptomycin, and 10 ng/mL cytokines (IL-4 and GM-CSF, Peprotech, Rocky Hill, USA). After culturing for 60 h, the medium was replaced with fresh complete medium. Mature DCs with a purity greater than 90% were harvested on the fifth day and used in subsequent experiments.
Analysis of the infectivity to DCs in vitro
When the MOI was 0.5 (at 6, 48, and 72 h p. i.), the expression of NP of infected DCs was measured using flow cytometry (FACS) after treating with fixed and permeabilized kits (BD biosciences, NY, USA). FITC-NP antibodies were purchased from Abcam (Cambridge, England).
Measuring the DCs migration into the nasal mucosa
When rPR8 and rPR8-△PAX infection doses were 107 EID50/50 μL (at 3 h. p. i.) (n = 3), the brain and nasal-associated lymphoid tissue (NALT) of six-week-old SPF C57BL/6 mice were removed, and single nasal cells were detached using 0.5% type XIV protease, 0.1% collagenase type IV, and 0.1% hyaluronidase, which were from Sigma-Aldrich (Missouri, USA). A total of 107 cells were collected using a 100-mesh sieve [Citation27]. Cells were mixed with APC-Cy7-FVS (used to identify living/dead cells), PerCP-Cy5.5-CD11c, PE-CD103, APC-CD11b, and their respective isotypes, and detected using FACS.
Cryosection and confocal observation
When the infection doses of rPR8 and rPR8-△PAX was 107 EID50/50 μL at 3 h. p. i. (n = 3), the nasal cavity of six-week-old SPF C57BL/6 mice were separated, placed in a 4% paraformaldehyde solution. After 12 h, the paraformaldehyde solution was discarded and the nasal tissue put into a decalcification solution. After 7 d, the tissue was embedded in OCT embedding medium (California, USA), and cut into frozen sections. For immunofluorescence staining, the samples were placed in 0.4% Triton X-100 and incubated for 5 min before adding 5% bovine serum albumin for 1 h. Armenian hamster anti-CD11c primary antibody (1:500) was added and mixed with rabbit anti-CCL20 (MIP-3α) pAb (1:500) and mouse anti-CD11b mAb (1:500) overnight and then incubated with goat anti-Armenian hamster IgG (H+L) Alexa Fluor 649 (1:1000), goat anti-rabbit IgG (H+L) Alexa Fluor 594 (1:1000), and rabbit anti-mouse IgG (H+L) Alexa Fluor 488 (1:1000) for 1 h. DAPI was added and the samples incubated for 5 min. Primary antibodies and DAPI were purchased from Abcam (Cambridge, England), and secondary antibodies were purchased from MultiSciences (Hangzhou, China). A Leica TCS SP8 STED confocal microscope (Leica Microsystems, Wetzlar, Germany) was used to observe the cryosections and Image-Pro Plus 6.0 was used to analyse the data [Citation27].
Chemokine expression in vitro
CCL20 expression on Calu-3 cells was analysed using qRT-PCR as previously described [Citation38], when the infection was 0.01 MOI for 6 and 24 h. In brief, after using the RNA extraction kit (Vazyme, Nanjing, China) to extract the RNA of Calu-3 cells according to the instructions, the CCL20 gene in the RNA was amplified with the designed CCL20 gene primers. Primer sequences (5′‑3′) were as follows: CCL20 (F: ACTGAGGAGACGCACAATATAT; R: TGTACCAAGAGTTTGCTCCTGG). GAPDH (F: GTCTCCTCTGACTTCAACAGCG; R: ACCACCCTGTTGCTGTAGCCAA).
Migration assay of DCs in vitro
When DCs were infected with the infection dose of 0.5 MOI for 24 h, cells were diluted to 1 × 106 cells/mL. A 0.6 mL volume of diluted CCL19 (final concentration 200 ng/mL) was added to a 24-well plate, the chamber (5 μm pore size; Corning, NY, USA) placed into the plate, and diluted DCs added to the chamber. After incubation at 37°C for 4 h, cells on the basal side were counted. A control group was set up consisting of 0.6 mL RPMI 1640 medium without CCL19 added to the lower chamber and 0.1 mL of uninfected DCs added to the upper chamber [Citation37].
Migration ability of the infected DCs to CLNs and their phenotype levels
rPR8 and rPR8-△PAX infection doses were 107 EID50/50 μL, at 6 h. p. i., the CLNs of six-week-old SPF C57BL/6 mice were extracted and placed on a sterile plate containing PBS and passed through a 100-mesh sieve [Citation39]. A total of 107/100 μL cells were collected and mixed with APC-Cy7-FVS (used for identifying living/dead cells), APC-CD11c, PE-Cy7-CCR7, and their respective isotypes. DCs were incubated with FITC-NP to detect their infectivity in the CLNs. To detect DC phenotypes, DCs were mixed with PE-CD40 and FITC-MHC II and detected using FACS.
Analysis of endocytosis, phenotypes, activation, and migration of DCs
When the MOI was 0.5, the morphology of DCs in different groups was observed under an optical microscope at 24 h. p. i. A positive control consisting of 100 ng/mL lipopolysaccharide (LPS)-stimulated DCs was included. LPS was obtained from Sigma-Aldrich (Missouri, USA). DCs were randomly selected and their lengths measured. The morphology index was calculated from 30 DCs randomly selected from five separate experiments, with six cells from each experiment (morphological index = longest axis/shortest axis) [Citation40]. To determine endocytosis, cells were incubated with 1 mg/mL FITC-dextran (Sigma-Aldrich, Missouri, USA) with LPS as a positive control at 37℃ for 30 min [Citation40] and detected via FACS. Phenotypes, activation, and migration of DCs were determined by FACS after incubating the cells with various antibodies, including PE-CD80, FITC-CD86, PE-CD40, FITC-MHC II, PerCP-Cy5.5-CD69, and PE-CCR7 (BD Biosciences, NY, USA).
Detection of cytokines
When the MOI was 0.5 (at 24 h p. i.), the levels of IL-1β, IL-6, TNF-α, and IL-10 from the supernatants of DCs were measured using ELISA kits (Multisciences, Hangzhou, China) with the manufacturer’s instructions [Citation38].
Statistical analysis
Data are expressed as mean ± standard deviation (mean ± SD) by GraphPad Prism 9 software (San Diego, CA). Unpaired two-sided student’s t-test was used to measure statistical significance between the two groups, and one-way ANOVA was used to test the significance of differences among groups. Analyses were conducted using SPSS 17.0. *P < 0.05, **P < 0.01.
Results
PA-X protein of H1N1 viruses enhanced pathogenicity in mammals
First, we rescued the recombinant virus, rPR8-△PAX, lacking the PA-X protein according to the strategy shown in . To determine the stability of rescued viruses, chicken embryos were inoculated for five consecutive passages. The sequencing results were correct (no other mutations were generated), and the HA titre was stable and higher than 10 Log2 (Figure S1, Table S1). Western blotting results showed that PA-X protein expression in rPR8-△PAX was significantly downregulated compared with PA-X expression in rPR8 (). Viral replication ability was also compared in avian original cells (DF-1 cells), as well as mammalian cells (Calu-3 cells). Virus growth curves showed that the replication levels of rPR8 in DF-1 cells had an upward trend compared with the replication levels of rPR8-△PAX, particularly in later stages of infection (). For Calu-3 cells, a replacement model for nasal epithelial cells [Citation28,Citation29], during the whole infection period the replication level of rPR8 was significantly higher than rPR8-△PAX (P < 0.01) (). Mice were intranasally inoculated at the dose of 101-106 EID50 in 50 μL PBS with each virus to assess the influence on pathogenicity in vivo (). Weight loss and mortality results showed that mice died when inoculated with 103 EID50/50 μL of rPR8 (, Ff,h), while mice inoculated with rPR8-△PAX started to die once they were challenged with 104 EID50/50 μL of the virus (, i). The MLD50 of rPR8 was 103.68 EID50/50 μL, while that of rPR8-△PAX was 104.37 EID50/50 μL, indicating an enhanced virulence of the PR8 strain possessing the PA-X protein. Similarly, the virus replication results showed that the viral EID50 titre of rPR8 was higher than that of rPR8-△PAX (P < 0.05) on 5 d. p. i. (), indicating increased rPR8 viral load in the lungs. Histopathological analysis based on H&E staining showed that rPR8 caused severe interstitial pneumonia with massive haemorrhage and extensive infiltration of inflammatory cells. However, the pathological changes in rPR8-△PAX-infected lungs were alleviated (, l). In summary, PA-X protein of H1N1 viruses enhanced the mammalian pathogenicity.
Figure 1. In vitro and in vivo analysis of biological characteristics of rescued viruses. (a) Schematic diagram of H1N1 PA-X-deficient viruses. The red letters represent nucleotides at the mutation sites. (b) PA-X, NP, and β-actin expression was measured using western blotting assay (MOI of 10 at 12 h p. i.). (c-d) Growth curves of rPR8 and rPR8-△PAX viruses in DF-1 (c) and Calu-3 (d) cells with an infection dose of 0.01 MOI at 72 h. p. i. Analysis was based on unpaired two-sided student’s t-test. (e-l) Six-week-old SPF BALB/c mice were infected with rPR8 and rPR8-△PAX (101-106 EID50/50 μL doses). Body weights and survival were recorded in rPR8-infected (f, h) and rPR8-△PAX-infected (g, i) mice over a 14-d period (n = 5). (j) Virus replication in lungs (n = 3). (k) H&E staining of lung sections (n = 3). Scale bar = 100 μm. (l) Histopathological scores of lungs on 5 d. p. i. (n = 3). Data are presented as mean ± SD. One-way ANOVA was used to measure statistical significance. *P <0.05, **P <0.01. MOI, multiplicity of infection; SPF, specific-pathogen-free; h. p. i., hours post infection; d. p. i., days post infection; ANOVA, analysis of variance.
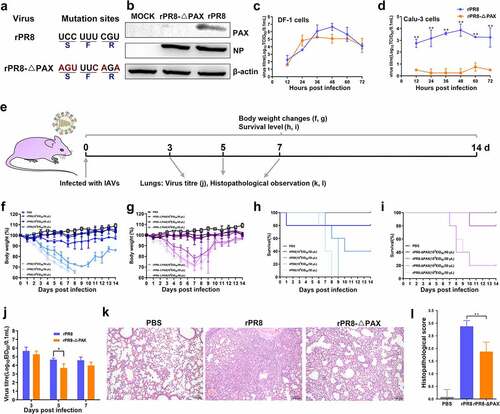
PA-X protein of H1N1 viruses inhibited the viral infection ability to mucosal DCs
NP protein expression was detected using FACS, and compared with rPR8-△PAX, the ability of rPR8 to infect DCs was reduced, particularly 6 and 48 h. p. i. (P < 0.05) (, b). In vivo studies showed that the number of mucosal CCR7+ CD11c+ DCs migrating to CLNs decreased after mice were intranasally infected with rPR8 (, d); meanwhile, the level of NP protein in these migrated DCs was also decreased in comparison with rPR8-△PAX group (P < 0.05) (, f). In conclusion, the PA-X protein of H1N1 viruses inhibited the ability of viruses to infect the mucosal DCs.
Figure 2. Determining the ability of viruses to infect DCs. (a, b) NP expression on DCs was detected at 6, 48, and 72 h. p. i. (MOI of 0.5). (c-f) When the infection dose was 107 EID50 in 50 μL PBS, the infectivity of DCs in murine CLNs was detected by FACS. (c) G1 represents CCR7+ CD11c+ DCs in living cells. (d) FACS statistic of (c), the proportion of CCR7+ CD11c+ DCs. (e) is from G1 for analysing the level of NP protein. (f) FACS statistic of (e) based on the MFI. Data are presented as mean ± SD. One-way ANOVA was used to measure statistical significance. *P <0.05, **P <0.01. DCs, dendritic cells; MOI, multiplicity of infection; h. p. i, hours post infection; PBS, phosphate buffered saline; CLNs, cervical lymph nodes; FACS, flow cytometry; MFI, mean fluorescence intensity; ANOVA, analysis of variance.
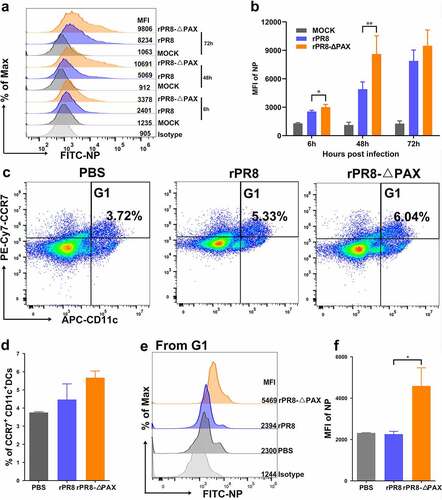
PA-X protein of H1N1 subtype IAVs inhibited CCL20 expression to reduce the DC mobilization to the nasal submucosa
Analysis of rPR8 and rPR8-△PAX strains at 3 h. p. i. (infection dose of 107 EID50/50 μL) using FACS demonstrated that the number of DCs recruited to the nasal mucosa of rPR8-infected mice was lower, in particular, both CD11b+ and CD103+ subtypes of DCs were dramatically reduced compared with rPR8-△PAX group (P < 0.05) (). Notably, more CD11b+ CD11c+ DCs were mobilized compared with CD103+ CD11c+ DCs. Furthermore, observation under a confocal microscope revealed that rPR8-infected nasal epithelial cells secreted less chemokine CCL20 to recruit CD11b+ CD11c+ DCs to the nasal submucosa in comparison to the rPR8-△PAX group (P<0.01) (), in line with in vitro results which showed that the rPR8 virus possessing PA-X protein significantly downregulated the expression of CCL20 compared with rPR8-△PAX at both 6 and 24 h. p. i. (P < 0.01) (). Collectively, PA-X protein of H1N1 viruses inhibited the expression of CCL20 in the nasal mucosa, subsequently suppressing the ability to rapidly mobilize DCs, the key cells involved in initiating mucosal immune responses against IAVs.
Figure 3. In vivo recruitment of DCs into the nasal mucosa. (a-e) Once infection reached 107 EID50 in 50 μL PBS in mice, the number of DCs recruited to the nasal mucosa was measured. (a-b) Recruitment of DCs into the nasal mucosa was measured using FACS at 3 h. p. i.. (a) G1 represents CD11c+ DCs from living cells. (b) G2 or G3 from G1 represent CD11b+CD11c+or CD103+ CD11c+ DCs, respectively. (c-e) FACS statistic of (a) and (b), the proportion of CD11c+ DCs (c), CD11b+CD11c+ DCs (d), and CD103+CD11c+ DCs (e). (f-h) Confocal microscopic examination of murine nasal mucosa following intranasal infection. Quantification of CCL20 (g) or CD11b+ CD11c+ (h) positive cells in nasal epithelial cells (n= 3). Nuclei (blue), CCL20 (pink), CD11c (red), CD11b (green). Scale bar=10 μm. (i, j) Transcriptional levels of CCL20 in Calu-3 cells measured at 6 (i) and 24 (j) h. p. i. when the MOI was 0.01. Data are presented as mean ± SD. One-way ANOVA was used to measure statistical significance. *P < 0.05, **P < 0.01. DCs, dendritic cells; FACS, flow cytometry; h. p. i., hours post infection; MOI, multiplicity of infection; ANOVA, analysis of variance.
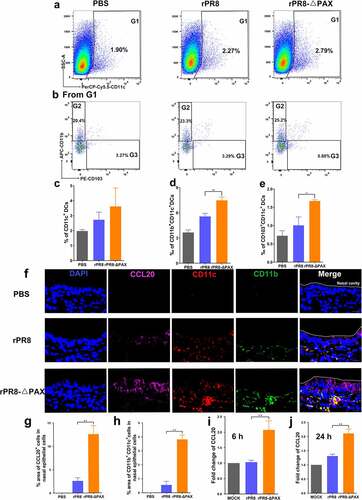
PA-X protein of H1N1 viruses repressed the DC maturation and migration to nearby draining lymph nodes
CCR7 is a migration marker that guides DCs to nearby draining lymph nodes [Citation41]. CCR7 expression in rPR8-infected DCs with an infection dose of 0.5 MOI at 24 h. p. i. was significantly downregulated compared with expression in rPR8-△PAX-infected DCs (P < 0.05) (, b). Furthermore, a transwell system was established to simulate the migration capability of DCs towards nearby draining lymph nodes. As shown in , d, the number of rPR8-infected DCs migrating toward CCL19 was reduced markedly (P < 0.01). Agreed with the results in vitro, FACS results demonstrated the number of CCR7+ CD11c+ DCs migrating to CLNs of the mice infected with rPR8 was decreased compared with rPR8-△PAX group (, f). In the rPR8 infected group, MHC II expression was significantly downregulated (P < 0.01) in the migrated DCs. Similar trends were observed when the other phenotypic marker, CD40, was analysed (, h). Collectively, PA-X protein of H1N1 viruses decreased the ability of DCs to migrate to CLNs and inhibited the phenotypic maturation of DCs.
Figure 4. In vitro and in vivo analysis of the ability of DCs to migrate to CLNs. DCs were infected with rPR8 or rPR8-△PAX (MOI = 0.5) in vitro. (a, b) FACS analysis of CCR7 expression in the samples collected at 24 h. p. i. (c, d) CCL19, a chemokine secreted from draining lymph nodes, was added to the lower chamber and the infected DCs were added to the upper chamber. The number of migrating DCs was then counted. (e-h) Six-week-old SPF C57BL/6 mice were intranasally infected with rPR8 or rPR8-△PAX viruses (107 EID50 in 50 μL PBS), the DCs in murine CLNs was detected by FACS at 6 h. p. i. (e) G1 represents CCR7+ CD11c+ DCs from living cells. (f) FACS statistic of (e), the percent of CCR7+ CD11c+ DCs. (g) is from G1 for analysing the MFI levels of MHC II and CD40 on the CCR7+ CD11c+ DCs. (h) FACS statistic of (g). Data are presented as mean ± SD. One-way ANOVA was used to measure statistical significance. *P <0.05, **P <0.01. h. p. i., hours post infection; DCs, dendritic cells; CLNs, cervical lymph nodes; SPF, specific-pathogen-free; PBS, phosphate buffered saline; FACS, flow cytometry; MOI, multiplicity of infection; ANOVA, analysis of variance; MFI, mean fluorescence intensity.
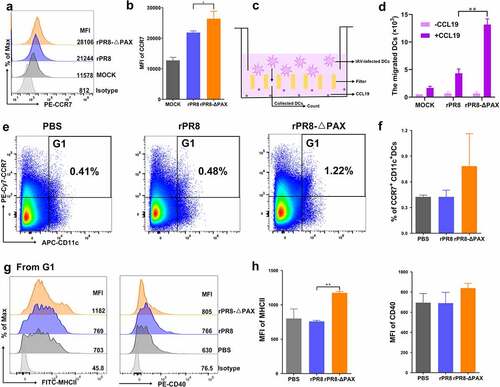
PA-X protein of H1N1 viruses enhanced endocytosis and suppressed phenotypic maturation of DCs
Mature DCs form long extensions much easier than immature DCs [Citation40]. We found that the cell shape index of DCs infected with the rPR8 strain possessing the PA-X protein was lower than that of the rPR8-△PAX strain (P < 0.05) where PA-X protein was knocked down (, b). Compared with mature DCs, immature DCs can take up antigens more efficiently through endocytosis capacity [Citation42]. Our results showed that rPR8 increased the endocytosis of FITC-labelled dextran in DCs (P < 0.01) (, d), suggesting that DCs hardly developed into their mature state. The phenotypic maturation of DCs is characterized by CD80, CD86, CD40, and MHC II [Citation38]; therefore, we inoculated DCs with PR8 or rPR8-△PAX with the infection dose of 0.5 MOI at 24 h. p. i., and detected the expression of phenotypic markers by FACs. Compared with rPR8-△PAX, the CD80, CD86, CD40, and MHC II expression of rPR8 were significantly decreased (P < 0.01) (, f), indicating that PA-X protein of H1N1 viruses inhibited the phenotypic maturation of DCs.
Figure 5. The morphological changes, endocytosis, and phenotypic maturation of DCs following infection in vitro. DCs were infected with rPR8 or rPR8-△PAX at a dose of 0.5 MOI. (a) the morphological characteristic of DCs were analysed at 24 h. p. i., with LPS as a positive control. Scale bar = 20 μm. (b) the results of the cellular shape indices (major axis/minor axis) (n = 30, 30 DCs were randomly selected from 5 separate experiments, 6 cells from each experiment). (c, d) Endocytosis by DCs was detected at 24 h. p. i. using FACS, with LPS as a positive control. (e, f) the MFI of phenotypic markers on DCs was measured using FACS at 24 h. p. i.. Data are presented as mean ± SD from one of three independent experiments. One-way ANOVA was used to measure statistical significance. *P <0.05, **P <0.01. DCs, dendritic cells; MOI, multiplicity of infection; h. p. i., hours post infection; LPS, lipopolysaccharide; FACS, flow cytometry; MFI, mean fluorescence intensity.
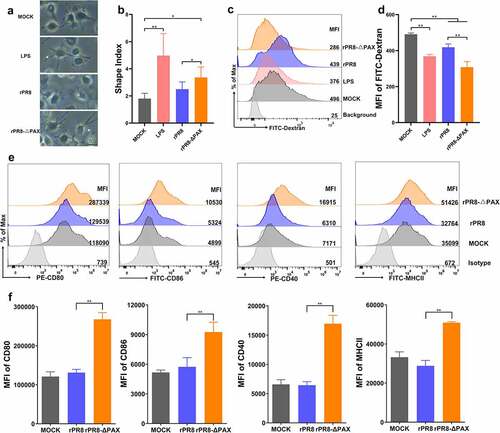
PA-X protein of H1N1 viruses inhibited the pro-inflammatory cytokine response of DCs
When DCs were infected with the dose of 0.5 MOI for 24 h, CD69 expression was enhanced, indicating that DCs were activated (P < 0.01) (, b). Furthermore, the levels of secreted IL-1β, IL-6, and TNF-α in rPR8-infected DCs were dramatically lower compared with their levels in rPR8-△PAX-infected cells (P < 0.01), whereas IL-10 secretion was significantly higher (P < 0.05) (). Collectively, PA-X protein of H1N1 viruses inhibited the pro-inflammatory cytokine response of DCs.
Figure 6. Cytokine responses in DCs. (a, b) CD69 expression was detected using FACS once the MOI reached 0.5 in vitro. (c) DC supernatants were collected at 24 h. p. i., and then the levels of secreted cytokines (IL-1β, IL-6, TNF-α, and IL-10) were measured using the ELISA kits. Data are presented as mean ± SD from one of three independent experiments. One-way ANOVA was used to measure statistical significance. *P <0.05, **P <0.01. DCs, dendritic cells; FACS, flow cytometry; MOI, multiplicity of infection; h. p. i., hours post infection.
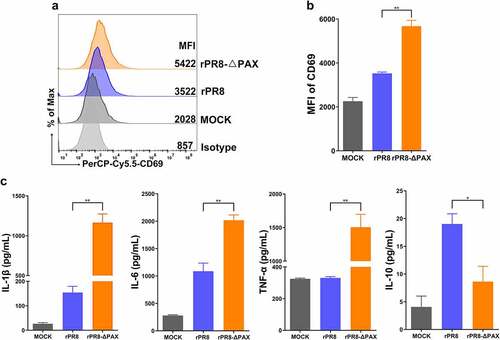
Discussion
Here, our study analysed the immune escape functions of PA-X protein on the innate immunity of mammalian hosts from the perspective of mucosal DCs, which laid the foundation for understanding the pathogenic mechanism of H1N1 viruses. Our findings suggested that PA-X protein of H1N1 viruses (PR8) increased the infection to the virus-productive cells (DF-1 and Calu-3) but not immune cells (DCs). Moreover, PA-X protein inhibited the CCL20 secretion from mucosal ECs and thereby retarded the recruitment of CD11b+ subtype DCs to the submucosa. Meanwhile, PA-X protein attenuated the migration of CCR7+ DCs to CLNs and further impaired the maturation of DCs for the suppression of downstream immune responses. Finally, the viral pathogenicity was enhanced in mice based on the immune escape mechanism of PA-X ().
Figure 7. PA-X protein of H1N1 influenza virus inhibited the immune functions of nasal mucosal DCs for increasing virulence. PA-X enhanced H1N1 influenza virus to infect ECs (I-1) but reduced the ability to infect DCs (I-2). (II) CCL20, a chemokine that monitors the recruitment of DCs to the submucosa, was downregulated by PA-X, resulting in an inhibition of the DC recruitment. (III) PA-X attenuated the migration of CCR7+ DCs to CLNs for suppression of downstream immune responses. (Ⅳ) PA-X impaired the maturation of DCs in the CLNs by downregulating the phenotypic markers and the levels of secreted pro-inflammatory cytokines while enhancing endocytosis and anti-inflammatory IL-10 levels. (Ⅴ) Based on above immune escape mechanism, the pathogenicity of H1N1 rPR8 strain was finally enhanced in mice. TJs, tight junctions; CLNs, cervical lymph nodes; DCs, dendritic cells; ECs, epithelial cells.
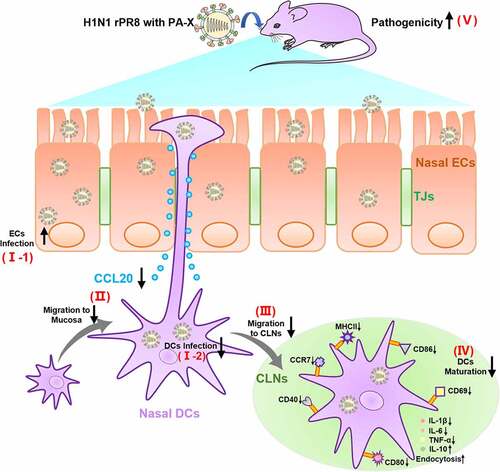
Multiple functions of PA-X protein in the pathogenicity and host immunity have been reported [Citation43]. Previous studies found no differences in pathogenicity of H1N1 virus in mice infected with H1N1 PR8 viruses possessing PA-X protein within 48 h [Citation24], although other studies have shown that PA-X protein can increase the virulence of PR8 in chicken embryos [Citation22]. However, the virulence induced by the PA-X protein of PR8 viruses in mice has not been fully elucidated. Therefore, a systematic study of pathogenicity in mice was carried out using the MLD50, lung titres, and histopathological observations. Our murine pathogenicity assay indicated that the PA-X protein of PR8 H1N1 viruses acts as a virulence factor, consistent with results from previous analyses of Ca09 (A/California/04/2009) and H1N2 SIVs [Citation20,Citation21,Citation23]. In contrast, other studies have shown that the PA-X protein is a negative regulator of virulence in some HP strains such as H5N1 viruses [Citation19], suggesting that PA-X may play different roles in virulence. DCs are “sentinels” of pathogens and leaders of the innate immune response [Citation32]. PA-X protein of H1N1 viruses inhibited DC infection both in vitro and in vivo but increased its replication in DF-1 and Calu-3 cells and viral pathogenicity in mice, indicating that viral replication differed in immune and non-immune cells. One possible reason is that DCs, as professional antigen-presenting cells (APCs), have more complex internal structures than non-immune cells, including a strong lysosomal system that may impact viral replication [Citation44]. Another possible explanation for this discrepancy was the host shutoff activity of the PA-X protein, which impaired the antiviral responses by inhibiting host protein synthesis [Citation19]. Non-immune cells are the targets of infection and perform the productive infection. Therefore, the host shutoff activity could turn off other host genes, but not virus genes in the viral productive cells. However, in our study, it was speculated that the PA-X protein not only shut down the host gene expression but also the viral gene expression in DCs, which might reduce the activation of DCs for suppressing immunity, and obtain a long-term replication in productive non-immune cells. In addition, the number of NP-expressed DCs that migrated to CLNs decreased for inhibiting the activation of downstream immune responses through cascade amplification and then enhancing infection and pathogenicity through delayed immune responses.
DCs constituted the first line of defence of the mucosal immunity [Citation45]. On one hand, antigens stimulate the secretion of a series of cytokines that regulate innate immunity [Citation38]. On the other hand, DCs recognize chemokines such as CCL20 that are secreted by mucosal epithelial cells facilitating them to move to the submucosal regions, extend their dendrites to take up pathogenic microorganisms, and then quickly migrate to nearby lymph nodes to present antigens to T and B lymphocytes, inducing an adaptive immune response [Citation32]. DCs that mediate cytotoxic T cell (CTL) responses against IAV infection are divided into CD103+ and CD11b+ DCs [Citation46]. Lack of CD103+ DCs significantly increases mortality in mice [Citation11,Citation47]. CD11b-expressing DCs are associated with inflammatory stimuli and are present in the airways during immune response activation [Citation48–50]. Our data indicated that PA-X protein of H1N1 viruses decreased CCL20 secretion in the nasal mucosa, reducing DC recruitment to the submucosa, particularly CD11b+ DCs. Subsequently, the virus hijacked DCs by inhibiting CCR7 expression, reducing the maturation and migration of DCs to CLNs. PA-X protein of H1N1 viruses can shutoff the host activity, inhibiting the innate immune response in both nasal epithelial cells and mucosal DCs and facilitating viral immune escape. Some pathogens have also evolved mechanisms to hijack DCs to favour their infection. For example, human cytomegalovirus (HCMV) can inhibit DC signalling and T cell proliferation, resulting in its long-term persistence [Citation51]. Hepatitis B virus (HBV) can utilize the C-type lectin receptor (CLR) pathway to hijack DC subsets and evade the immune control [Citation52]. Nonstructural protein 2 (NS2) of IAV inhibits the maturation and antigen-presenting capacity of DCs, leading to influenza virus epidemics [Citation53].
The transition from immature to mature DCs is crucial for the downstream cascade of immune responses [Citation54]. Immature DCs (iDCs) have a strong ability to take up and process antigens, although low levels of CD80, CD86, CD40, and MHC II are expressed on the cell surface [Citation55]. Once pathogen is taken up, iDCs are transformed into mature DCs that are highly capable of stimulating T-cell proliferation and differentiation via upregulation of MHC II and co-stimulatory molecules (CD80 and CD86) [Citation56]. Another study found that if the CD40 function of DCs was inhibited, T cells would be inhibited as well [Citation57]. Our data demonstrated that PA-X downregulated the phenotypic markers of DCs, which was different fromHBV vaccine that can upregulate the expression of MHC II and CD86, thereby enhancing the effect of immunotherapy in order to perform anti-virus functions [Citation58]. The reduction of phenotype markers by the PA-X protein of H1N1 PR8 viruses may prevent the activation and maturation of DCs and subsequently inhibit the downstream immune cascade, thereby the immune escape is achieved.
Mature DCs can secrete cytokines under pathogenic stimulation, promoting the systemic immune responses and maintaining the immune homoeostasis. CD69 is an early activation marker expressed following DC activation that can activate T-cells [Citation59]. CD69-induced signalling can be adjusted not only to APCs but also to the immune response of cytokines and other metabolites in peripheral cells [Citation60]. IL-1β, a key cytokine involved in inflammatory response, is essential for host protection [Citation61]. IL-6, a significant regulator of the proliferation and survival of T cells, is significantly elevated after influenza infection, and T cells play a significant part in respiratory virus elimination [Citation62]. TNF-α can also regulate immune cells, induce cellular inflammatory responses, and inhibit virus replication [Citation63]. IL-10, as an anti-inflammatory cytokine, can inhibit the antigen uptake function of APCs [Citation64]. Our data indicated that PA-X protein of H1N1 viruses inhibited the DC activation by downregulating CD69 and impaired the cytokine response via the downregulation of pro-inflammatory cytokines (IL-1β, IL-6, and TNF-α) and upregulating anti-inflammatory cytokine (IL-10), implying a functional maturation of DCs was suppressed by PA-X protein. Infection with H1N1 PR8 virus affects the maturation of DCs, preventing the mobilization of diverse immune cells to participate in viral clearance or activation of the downstream specific immunity, giving the virus a great opportunity to replicate. Similarly, the transmissible gastroenteritis virus (TGEV) can reduce the maturation ability of intestinal mucosal DCs, thus weakening their ability to present viral antigens to T cells and allowing the virus to infect epithelial cells in large numbers [Citation65]. In addition, one possible explanation for the discrepancy between these cytokines is that the endonuclease activity of PA-X protein was selective [Citation26] and IL-10 might escape the selective host-shutoff activity.
In summary, our results demonstrated PA-X protein disabled H1N1 viruses in stimulating nasal DCs, which might contribute to enhance virulence in mammals. This work would be helpful to reveal the synergistic regulation ability of PA-X protein on different host cells for suitable virulence.
Data availability
All data are available from the authors upon reasonable request.
Supplemental Material
Download MS Word (203.4 KB)Disclosure statement
No potential conflict of interest was reported by the author(s).
Supplementary material
Supplemental data for this article can be accessed online at https://doi.org/10.1080/21505594.2022.2139474
Additional information
Funding
References
- Taubenberger JK, Morens DM. Influenza: the once and future pandemic. Public Health Rep. 2010;125(3):16–26.
- Klemm C, Boergeling Y, Ludwig S, et al. Immunomodulatory nonstructural proteins of influenza a viruses. Trends Microbiol. 2018;26(7):624–636.
- Yamayoshi S, Watanabe M, Goto H, et al. Identification of a novel viral protein expressed from the PB2 segment of influenza a virus. J Virol. 2016;90(1):444–456.
- Dave K, Lee PC. Global geographical and temporal patterns of seasonal influenza and associated climatic factors. Epidemiol Rev. 2019;41(1):51–68.
- Pavia A. One hundred years after the 1918 pandemic: new concepts for preparing for influenza pandemics. Curr Opin Infect Dis. 2019;32(4):365–371.
- Reid AH, Taubenberger JK, Fanning TG. Evidence of an absence: the genetic origins of the 1918 pandemic influenza virus. Nat Rev Microbiol. 2004;2(11):909–914.
- Cao B, Li XW, Mao Y, et al. Clinical features of the initial cases of 2009 pandemic influenza a (H1N1) virus infection in China. N Engl J Med. 2009;361(26):2507–2517. DOI:10.1056/NEJMoa0906612
- Swine-Origin Influenza AVIT N, Dawood FS, Jain S, et al. Emergence of a novel swine-origin influenza a (H1N1) virus in humans. N Engl J Med. 2009;360:2605–2615.
- Smole U, Schabussova I, Pickl WF, et al. Murine models for mucosal tolerance in allergy. Semin Immunol. 2017;30:12–27.
- Qu W, Li N, Yu R, et al. Cationic DDA/TDB liposome as a mucosal vaccine adjuvant for uptake by dendritic cells in vitro induces potent humoural immunity. Artif Cells Nanomed Biotechnol. 2018;46(sup1):852–860. DOI:10.1080/21691401.2018.1438450
- GeurtsvanKessel CH, Willart MA, van Rijt LS, et al. Clearance of influenza virus from the lung depends on migratory langerin+cd11b− but not plasmacytoid dendritic cells. J Exp Med. 2008;205(7):1621–1634. DOI:10.1084/jem.20071365
- Ding X, Yang W, Shi X, et al. TNF receptor 1 mediates dendritic cell maturation and CD8 T cell response through two distinct mechanisms. J Immunol. 2011;187(3):1184–1191. DOI:10.4049/jimmunol.1002902
- Smed-Sorensen A, Chalouni C, Chatterjee B, et al. Influenza a virus infection of human primary dendritic cells impairs their ability to cross-present antigen to CD8 T cells. PLoS Pathog. 2012;8(3):e1002572. DOI:10.1371/journal.ppat.1002572
- Le Nouen C, Hillyer P, Winter CC, et al. Low CCR7-mediated migration of human monocyte derived dendritic cells in response to human respiratory syncytial virus and human metapneumovirus. PLoS Pathog. 2011;7(6):e1002105. DOI:10.1371/journal.ppat.1002105
- Palomino-Segura M, Perez L, Farsakoglu Y, et al. Protection against influenza infection requires early recognition by inflammatory dendritic cells through C-type lectin receptor SIGN-R1. Nat Microbiol. 2019;4(11):1930–1940. DOI:10.1038/s41564-019-0506-6
- Munir S, Le Nouen C, Luongo C, et al. Nonstructural proteins 1 and 2 of respiratory syncytial virus suppress maturation of human dendritic cells. J Virol. 2008;82(17):8780–8796.
- Lichtner M, Mastroianni CM, Rossi R, et al. Severe and persistent depletion of circulating plasmacytoid dendritic cells in patients with 2009 pandemic H1N1 infection. PLoS One. 2011;6(5):e19872. DOI:10.1371/journal.pone.0019872
- Jagger BW, Wise HM, Kash JC, et al. An overlapping protein-coding region in influenza a virus segment 3 modulates the host response. Science. 2012;337(6091):199–204. DOI:10.1126/science.1222213
- Hu J, Mo Y, Wang X, et al. PA-X decreases the pathogenicity of highly pathogenic H5N1 influenza a virus in avian species by inhibiting virus replication and host response. J Virol. 2015;89(8):4126–4142. DOI:10.1128/JVI.02132-14
- Hayashi T, MacDonald LA, Takimoto T. Influenza a virus protein PA-X contributes to viral growth and suppression of the host antiviral and immune responses. J Virol. 2015;89(12):6442–6452.
- Lee J, Yu H, Li Y, et al. Impacts of different expressions of PA-X protein on 2009 pandemic H1N1 virus replication, pathogenicity and host immune responses. Virology. 2017;504:25–35.
- Hussain S, Turnbull ML, Wise HM, et al. Mutation of influenza a virus PA-X decreases pathogenicity in chicken embryos and can increase the yield of reassortant candidate vaccine viruses. J Virol. 2019;93(2):18–e01551. DOI:10.1128/JVI.01551-18
- Xu G, Zhang X, Liu Q, et al. PA-X protein contributes to virulence of triple-reassortant H1N2 influenza virus by suppressing early immune responses in swine. Virology. 2017;508:45–53.
- Rigby RE, Wise HM, Smith N, et al. PA-X antagonises MAVS-dependent accumulation of early type I interferon messenger RNAs during influenza a virus infection. Sci Rep. 2019;9(1):7216.
- Nogales A, Rodriguez L, DeDiego ML, et al. Interplay of PA-X and NS1 proteins in replication and pathogenesis of a temperature-sensitive 2009 pandemic H1N1 influenza a virus. J Virol. 2017;91(17):91.
- Gaucherand L, Porter BK, Levene RE, et al. The influenza a virus endoribonuclease PA-X usurps host mRNA processing machinery to limit host gene expression. Cell Rep. 2019;27(3):776–92 e7. DOI:10.1016/j.celrep.2019.03.063
- Qin T, Ma S, Miao X, et al. Mucosal vaccination for influenza protection enhanced by catalytic immune-adjuvant. Adv Sci (Weinh). 2020;7(18):2000771. DOI:10.1002/advs.202000771
- Witschi C, Mrsny RJ. In vitro evaluation of microparticles and polymer gels for use as nasal platforms for protein delivery. Pharm Res. 1999;16(3):382–390.
- Amidi M, Romeijn SG, Borchard G, et al. Preparation and characterization of protein-loaded N-trimethyl chitosan nanoparticles as nasal delivery system. J Control Release. 2006;111(1–2):107–116.
- Gao H, Sun Y, Hu J, et al. The contribution of PA-X to the virulence of pandemic 2009 H1N1 and highly pathogenic H5N1 avian influenza viruses. Sci Rep. 2015;5(1):8262. DOI:10.1038/srep08262
- Qin T, Zhu J, Ma R, et al. Compatibility between haemagglutinin and neuraminidase drives the recent emergence of novel clade 2.3.4.4 H5Nx avian influenza viruses in China. Transbound Emerg Dis. 2018;65(6):1757–1769. DOI:10.1111/tbed.12949
- Qin T, Yin Y, Yu Q, et al. CpG oligodeoxynucleotides facilitate delivery of whole inactivated H9N2 influenza virus via transepithelial dendrites of dendritic cells in nasal mucosa. J Virol. 2015;89(11):5904–5918. DOI:10.1128/JVI.00296-15
- Jonges M, Liu WM, van der Vries E, et al. Influenza virus inactivation for studies of antigenicity and phenotypic neuraminidase inhibitor resistance profiling. J Clin Microbiol. 2010;48(3):928–940. DOI:10.1128/JCM.02045-09
- Reed LJ, Muench H. A simple method of estimating fifty percent endpoints. Am J Epidemiol. 1938;27(3):493–497.
- Gauger PC, Vincent AL, Loving CL, et al. Enhanced pneumonia and disease in pigs vaccinated with an inactivated human-like (δ-cluster) H1N2 vaccine and challenged with pandemic 2009 H1N1 influenza virus. Vaccine. 2011;29(15):2712–2719. DOI:10.1016/j.vaccine.2011.01.082
- Qin T, Ma R, Yin Y, et al. Catalytic inactivation of influenza virus by iron oxide nanozyme. Theranostics. 2019;9(23):6920–6935. DOI:10.7150/thno.35826
- Yin Y, Xu N, Shi Y, et al. Astaxanthin protects dendritic cells from lipopolysaccharide-induced immune dysfunction. Mar Drugs. 2021;19(6):346–361. DOI:10.3390/md19060346
- Chen S, Miao X, Huangfu D, et al. H5N1 avian influenza virus without 80–84 amino acid deletion at the NS1 protein hijacks the innate immune system of dendritic cells for an enhanced mammalian pathogenicity. Transbound Emerg Dis. 2021;68(4):2401–2413. DOI:10.1111/tbed.13904
- Choi J, Pant A, Medikonda R, et al. Sustained localized delivery of immunotherapy to lymph nodes reverses immunosuppression and increases long-term survival in murine glioblastoma. Oncoimmunology. 2021;10(1):1940673. DOI:10.1080/2162402X.2021.1940673
- Yin Y, Qin T, Yu Q, et al. Bursopentin (BP5) from chicken bursa of fabricius attenuates the immune function of dendritic cells. Amino Acids. 2014;46(7):1763–1774.
- Alvarez D, Vollmann EH, von Andrian UH. Mechanisms and consequences of dendritic cell migration. Immunity. 2008;29(3):325–342.
- Reise, Reis E Sousa C, Sousa C. Dendritic cells in a mature age. Nat Rev Immunol. 2006;6(6):476–483.
- Hu J, Ma C, Liu X. PA-X: a key regulator of influenza a virus pathogenicity and host immune responses. Med Microbiol Immunol. 2018;207(5–6):255–269.
- Chen X, Liu S, Goraya MU, et al. Host immune response to influenza a virus infection. Front Immunol. 2018;9:320.
- Clarkson BD, Heninger E, Harris MG, et al. Innate-adaptive crosstalk: how dendritic cells shape immune responses in the CNS. Adv Exp Med Biol. 2012;946:309–333.
- Reizis B. Plasmacytoid dendritic cells: development, regulation, and function. Immunity. 2019;50(1):37–50.
- Ballesteros-Tato A, Leon B, Lund FE, et al. Temporal changes in dendritic cell subsets, cross-priming and costimulation via CD70 control CD8(+) T cell responses to influenza. Nat Immunol. 2010;11(3):216–224.
- van Rijt LS, Jung S, Kleinjan A, et al. In vivo depletion of lung CD11c+ dendritic cells during allergen challenge abrogates the characteristic features of asthma. J Exp Med. 2005;201(6):981–991. DOI:10.1084/jem.20042311
- Sung SS, Fu SM, Rose CE Jr., et al. A major lung CD103 (α E)-β 7 integrin-positive epithelial dendritic cell population expressing langerin and tight junction proteins. J Immunol. 2006;176(4):2161–2172.
- Medoff BD, Seung E, Hong S, et al. Cd11b+ myeloid cells are the key mediators of Th2 cell homing into the airway in allergic inflammation. J Immunol. 2009;182(1):623–635. DOI:10.4049/jimmunol.182.1.623
- Sinclair J. Manipulation of dendritic cell functions by human cytomegalovirus. Expert Rev Mol Med. 2008;10:e35.
- Ouaguia L, Dufeu-Duchesne T, Leroy V, et al. Hepatitis B virus exploits C-type lectin receptors to hijack cDc1s, cDc2s and pDcs. Clin Transl Immunology. 2020;9(12):e1208. DOI:10.1002/cti2.1208
- Lin J, Cao Y, Shah AU, et al. Inhibition of the antigen-presenting ability of dendritic cells by non-structural protein 2 of influenza a virus. Vet Microbiol. 2022;267:109392.
- Banchereau J, Steinman RM. Dendritic cells and the control of immunity. Nature. 1998;392(6673):245–252.
- Min WP, Zhou D, Ichim TE, et al. Inhibitory feedback loop between tolerogenic dendritic cells and regulatory T cells in transplant tolerance. J Immunol. 2003;170(3):1304–1312. DOI:10.4049/jimmunol.170.3.1304
- Jensen SS, Gad M. Differential induction of inflammatory cytokines by dendritic cells treated with novel TLR-agonist and cytokine based cocktails: targeting dendritic cells in autoimmunity. J Inflamm (Lond). 2010;7(1):37.
- Alnaeeli M, Penninger JM, Teng YT. Immune interactions with CD4+ T cells promote the development of functional osteoclasts from murine CD11c+ dendritic cells. J Immunol. 2006;177(5):3314–3326.
- Akbar SK, Horiike N, Onji M. Prognostic importance of antigen-presenting dendritic cells during vaccine therapy in a murine hepatitis B virus carrier. Immunology. 1999;96(1):98–108.
- Kimura MY, Hayashizaki K, Tokoyoda K, et al. Crucial role for CD69 in allergic inflammatory responses: cD69-Myl9 system in the pathogenesis of airway inflammation. Immunol Rev. 2017;278(1):87–100.
- Cibrian D, Sanchez-Madrid F. CD69: from activation marker to metabolic gatekeeper. Eur J Immunol. 2017;47(6):946–953.
- Sarajlic M, Neuper T, Fohrenbach Quiroz KT, et al. IL-1β Induces SOCS2 expression in human dendritic cells. Int J Mol Sci. 2019;20(23):20. DOI:10.3390/ijms20235931
- Longhi MP, Wright K, Lauder SN, et al. Interleukin-6 is crucial for recall of influenza-specific memory CD4 T cells. PLoS Pathog. 2008;4(2):e1000006. DOI:10.1371/journal.ppat.1000006
- Kostinov MP, Akhmatova NK, Khromova EA, et al. Cytokine profile in human peripheral blood mononuclear leukocytes exposed to immunoadjuvant and adjuvant-Free vaccines against influenza. Front Immunol. 2020;11:1351.
- WKE I, Hoshi N, Shouval DS, et al. Anti-inflammatory effect of IL-10 mediated by metabolic reprogramming of macrophages. Science. 2017;356(6337):513–519.
- Zhao S, Gao Q, Qin T, et al. Effects of virulent and attenuated transmissible gastroenteritis virus on the ability of porcine dendritic cells to sample and present antigen. Vet Microbiol. 2014;171(1–2):74–86. DOI:10.1016/j.vetmic.2014.03.017