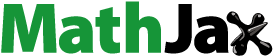
ABSTRACT
Human respiratory syncytial virus (hRSV) is a major cause of acute lower respiratory tract infections in children under the age of two as well as in the elderly and immunocompromised worldwide. Despite its discovery over 60 years ago and the global impact on human health, limited specific and effective prophylactic or therapeutic options have been available for hRSV infections. Part of the lack of treatment options is attributed to the legacy of vaccine failure in the 1960s using a formalin-inactivated RSV (FI-RSV), which led to enhancement of disease post exposure to hRSV infection and hampered subsequent development of vaccine candidates. Recent FDA approval of a vaccine for older adults and impending approval for a maternal vaccine are major advancements but leaves children between 6 months and 5 years of age unprotected. Part of this limitation can be attributed to a lack of complete understanding of the factors that contribute to hRSV pathogenesis. The nonstructural proteins NS1 and NS2 are multifunctional virulence factors that are unique to hRSV and that play critical roles during hRSV infection, including antagonizing interferon (IFN) signalling to modulate host responses to hRSV infection. However, the molecular mechanisms by which the nonstructural proteins mediate their IFN inhibitory functions have not been completely defined. Current progress on the characterization of NS1 and NS2 during infection provides deeper insight into their roles. Furthermore, reverse genetics systems for hRSV provide a viable strategy to generate attenuated viruses by introduction of select mutations while maintaining immunogenicity required to elicit a long-term protective response. Here we will review the current state of knowledge of the nonstructural proteins, their contributions to RSV pathogenesis, and their potential as targets for therapeutic development.
Disclaimer
As a service to authors and researchers we are providing this version of an accepted manuscript (AM). Copyediting, typesetting, and review of the resulting proofs will be undertaken on this manuscript before final publication of the Version of Record (VoR). During production and pre-press, errors may be discovered which could affect the content, and all legal disclaimers that apply to the journal relate to these versions also.hRSV disease
Human respiratory syncytial virus (hRSV) is a human pathogen that circulates seasonally as many other respiratory viruses. hRSV spreads readily through respiratory droplets and can cause mild symptoms in an average adult similar to the common cold, such as runny nose, coughing, and fever. In some cases, hRSV infection in the upper respiratory tract can lead to spread and more severe symptoms in the lower respiratory tract, especially in children under the age of 2 and adults older than 65 years of age. Severe symptoms include wheezing, difficulty breathing within 3 to 7 days after initial exposure, bronchiolitis, inflammation, and pneumonia (Citation1–3). Severity of disease is compounded by comorbidities such as chronic lung or heart disease and weakened immune systems. In the United States, hRSV poses a significant public health burden with socioeconomic impact. The Centers for Disease Control and Prevention (CDC) estimates that hRSV causes hospitalizations of over 60,000 children under the age of 5 and 180,000 adults over the age of 65 and deaths up to 500 children and 14,000 older adults (Citation4–12). These numbers are likely greater when considering the number of undiagnosed or unreported cases as well as global hospitalization and mortality rates for hRSV infections (Citation13, Citation14).
Viral genome
hRSV is a pleiomorphic enveloped virus, with spherical and filamentous forms (Citation15–18), that belongs to the Pneumoviridae family of nonsegmented, negative sense RNA viruses (NNSVs). hRSV along with bovine respiratory syncytial virus (bRSV) and murine pneumonia virus (MPV) (formerly pneumonia virus of mice or PVM) are in the Orthopneumovirus genus whereas human metapneumovirus (HMPV) is in the Metapneumovirus genus (Citation15, Citation19–21). hRSV is further classified into two subtypes A and B (A1, A2, B1, and B2). The prototypical genome of hRSV is approximately 15.2 kilobases (strain A2) encoding for 10 genes and 11 open reading frames (ORFs) in the order of 3’-NS1-NS2-N-P-M-SH-G-F-M2-L-5’ (). Like other NNSVs, replication of the negative-sense genome in cytoplasmic inclusion bodies requires generation of a positive-sense antigenome that serves as a template for RNA synthesis (Citation22, Citation23). Nucleoprotein (N) encapsidates the viral RNA genome and is part of the viral replication complex that is bridged by phosphoprotein (P) to the large RNA-dependent RNA polymerase (L) (Citation24–27). L contains domains required for RNA synthesis including an RdRp domain, an mRNA capping domain, and a methyltransferase domain. Recent structures of hRSV L bound to tetrameric P revealed that different regions in L interact with a conformationally dynamic P ().
Transcription proceeds by a sequential start-stop mechanism that results in a gradient of viral mRNA abundance from the 3’ end to the 5’ end () (Citation28). The switch from replication to transcription involves cofactor M2-1, which binds to N as well as RNA and functions as an anti-terminator and processivity factor (Citation29–32); M2-2 likely is involved in the switch from transcription to replication (Citation33). The first two genes that are proximal to the promoter and transcribed in great abundance are nonstructural 1 and 2, which encode for the NS1 and NS2 proteins that function as interferon (IFN) antagonists. This is followed by N, P, matrix (M), small hydrophobic (SH), glycoprotein (G), fusion (F), M2, and L. M is required for assembly along with G, F, and SH, which are surface transmembrane glycoproteins that function in attachment and entry (Citation34, Citation35).
Viral entry
hRSV infects ciliated epithelial cells in the upper respiratory tract and can spread to bronchiolar epithelium and alveolar cells in the lower respiratory system, including type I pneumocytes (Citation36, Citation37). Viral entry involves attachment to the cell membrane and fusion of the viral and cell membranes. Attachment to the cell is largely mediated by the G glycoprotein, which is genetically diverse between hRSV A and B subtypes. G is highly glycosylated and attaches to heparan sulfate proteoglycans (HSPGs) that are present on immortalized cells (Citation38, Citation39). However, HSPGs are absent in pulmonary cells that are infected by hRSV. Subsequent studies identified that CX3C chemokine receptor (CX3CR1), which is expressed in ciliated lung epithelial cells, is important for interactions with hRSV G and for viral attachment (Citation40). F glycoprotein, the sequence of which is highly conserved compared to G, is also involved in attachment and can interact with nucleolin (Citation41), epidermal growth factor receptor (EGFR) (Citation42), insulin-like growth factor-1 receptor (IGF1R) (Citation43), and intercellular adhesion molecule-1 (ICAM-1) (Citation44). However, hRSV F primarily facilitates fusion of viral and host membranes through interactions with other host receptors. After F is processed and trimerized, F undergoes conformational changes from a prefusion form to a postfusion form that allows insertion of F into the host membrane (). SH forms an ion channel and may be necessary to inhibit TNF-α mediated apoptosis; however, the importance of hRSV SH is less clear as SH is not required to form an infectious virion (Citation45–48). The presence of host receptors vary by cell type and elucidating the mechanism of host cell attachment by RSV F and G remains an open topic in the field that may involve a two-step fusion event or micropinocytosis followed by endosomal fusion and is reviewed elsewhere (Citation49).
IFN signaling in response to viral infection
Type I IFN signaling stimulates host innate immune responses and is a self-defense mechanism to combat viral infection and to prevent viral spread (Citation50, Citation51). The RIG-I like receptors (RLR) RIG-I and MDA5 are cytosolic pattern recognition receptors (PRR) that sense pathogen associated molecular patterns (PAMPS), which include viral RNAs (Citation52–59). Activation of RLRs results in the association of several adapter molecules, such as MAVS (also referred to IPS-1/Cardif/Visa), TRAF, and TRIF, that then recruits downstream signaling kinases TBK1, and
(). These activated kinases can subsequently phosphorylate the transcription factors IRF3 and IRF7, which dimerize and translocate into the nucleus to induce Type I IFN production, mainly IFNα/β. IFNα/β can then act in an autocrine or paracrine manner and signal through binding to the Type I IFN receptor (IFNAR1/2) leading to stimulation of the JAK-STAT signaling pathway. This activates the critical transcription factors STAT1 and STAT2, which homodimerizes or heterodimerizes and binds to IRF9, and localizes in the nucleus to induce transcription of IFN-stimulated response elements (ISRE), or antiviral genes, to establish an antiviral state (Citation60–62).
hRSV encoded IFN antagonists
Early studies of the hRSV nonstructural proteins revealed that NS1 and NS2 function as IFN antagonists, especially during the early stages of infection given their proximity to the 3’ UTR (Citation63, Citation64). NS1 and NS2 are found only in RSV (human, bovine, ovine, caprine, pneumovirus of mouse) and not in any of the other Mononegavirales viruses, including the closely related HMPV. The viral genome of the related paramyxoviruses or filoviruses lacks the NS1 and NS2 counterparts but encodes for accessory proteins, such as the V, W, and C proteins in paramyxoviruses or VP24 and VP35 in filoviruses, that have critical roles in antagonizing interferon (IFN) responses (Citation65, Citation66). Comparison of the NS1 and NS2 sequences reveal little sequence similarity to these accessory proteins and approximately 12% identity between NS1 and NS2, most notably in the last four residues encoding Asp-Leu-Asn-Pro. The functional significance of this motif is not clear, although there may be a role for these residues in mediating protein-protein interactions (Citation67).
Recombinant human RSVs (hRSVs) where deletion of NS1, NS2, or both genes (𝚫NS1, 𝚫NS2, 𝚫NS1/NS2) significantly increased IFNβ mRNA levels compared to wildtype hRSV infected cells, suggesting that both NS1 and NS2 suppressed IFN signaling (Citation68–72), similar to that observed for bovine NS1 and NS2 (Citation73, Citation74). NS1 and NS2 encode for proteins with approximate molecular weights of 15 and 14 kDa, respectively (Citation75–77), but are not considered to be part of the mature virion(Citation78), hence their designation as nonstructural proteins. It is not fully understood why RSV requires two proteins to mediate this specific function. However, recent studies provide evidence that NS1 and NS2 are multifunctional proteins that likely target different molecules in the IFN signaling pathway to suppress IFN production and responses cooperatively and potently during hRSV infection to facilitate viral pathogenesis ().
Cytosolic NS1-mediated inhibition of the IFN signaling pathway
Given that stimulation of IFN production depends on PRR activity, it is not surprising then that hRSV targets this crucial first step in the pathway, likely using multiple mechanisms. RIG-I is a cytoplasmic PRR that exists in a phosphorylated and autoinhibited conformation. Binding of viral RNA PAMPS to its C-terminal domain relieves autoinhibition and allows for the dephosphorylation and ubiquitination of the tandem N-terminal caspase activation and recruitment domain (CARD) and C-terminal domain (Citation79–81). A recent study showed that NS1 appears to decrease the ubiquitination of the CARD domains of RIG-I with increasing levels of NS1(Citation82). Coimmunoprecipitation assays revealed that NS1 precipitated with the SPRY domain of TRIM25, an E3-ubiquitin ligase that conjugates K63 ubiquitin chains on several lysine residues in RIG-I CARDs including Lys172 that is important for RIG-I activation (Citation83–85). NS1 can also colocalize with MAVS, which is mitochondrial associated, in A549 cells infected with hRSV A2 (Citation82, Citation86). NS1 also coimmunoprecipitates MAVS, suggesting that NS1 may be interfering with RIG-I signaling by binding to MAVS to further inhibit PRR activity (Citation82). NS1 localization at the mitochondria may be enhanced by the presence of NS2 and the formation of a heteromer (Citation67). The presence of NS1 on the mitochondria is consistent with a proteomics study that identified several mitochondrial proteins associated with NS1 (Citation87). NS1 may further suppress IFN signaling by facilitating the degradation of molecules in this pathway, including RIG-I, IRF3, and IRF7 (Citation88) as well as TRAF3 and , although there are contrasting reports on this (Citation88–90). NS1 association with MAVS on the mitochondria along with NS2 can potentially serve as the formation site of a viral “degradasome” containing many IFN signaling proteins (Citation88).
Suppression of RIG-I signaling by NS1 likely contributes to the decreased activation of several molecules downstream of RIG-I, including adapter protein TRAF3 and kinase IKKe that are important for the activation and phosphorylation of the transcription factor IRF3 (Citation73, Citation90). IRF3 appears to be targeted independently by NS1 as transfection of NS1 coimmunoprecipitates IRF3 and interferes with IRF3 binding to its coactivator CBP and therefore prevents IRF3 translocation into the nucleus and activation of the IFNβ promoter (Citation69, Citation89).
NS1 not only impacts IFN production, but also IFN responses. RSV infection inhibits signaling through the JAK/STAT pathway and specifically targets STAT2 (Citation91). NS1 has been shown to decrease STAT2 levels by facilitating its proteasomal degradation. Addition of protease inhibitors, mainly MG132, partially reduced STAT2 degradation (Citation89, Citation90, Citation92–94). NS1 also may be mediating degradation of STAT2 by forming an E3 ligase complex along with Cul2 and elongin C that targets STAT2 for K48-linked polyubiquitination and its subsequent degradation (Citation92, Citation95). In addition, hRSV NS1 can promote proteasome-dependent degradation of 2’-5’ oligoadenylate synthase-like protein (OASL), which is an IFN-inducible antiviral protein, further suggesting that there are multiple steps or cellular antiviral proteins that NS1 inhibits to evade cellular antiviral innate immune responses (Citation96).
Nuclear NS1-mediated inhibition of the IFN signaling pathway
Although NS1 targets the function of many cytosolic host proteins, several subsequent studies suggested that NS1 may have a regulatory role in nucleus even though hRSV replicates in the cytoplasm. NS1 was shown to partially localize in the nucleus of transfected 293T and A549 cells (Citation67, Citation69, Citation87, Citation90, Citation97–100). NS1 was shown to coimmunoprecipitate with histone H2B and stimulate its ubiquitination, potentially through an E3 ligase containing Elongin C and Cul2 (Citation92, Citation99), and results in HOX B5 and B6 gene expression in human bronchial epithelial cells. A subsequent proteomics study used EGFP-tagged NS1 to selectively precipitate interacting proteins in 293T cells and identified several nuclear associated proteins (Citation87). Among these were components involved in transcriptional regulation, including cyclin C, RNA polymerase II, and several subunits of Mediator complex, which are part of the preinitiation complex required for RNA polymerase II transcription. This was also verified in other independent studies, including one using StreptII-HA-tagged NS1 that identified 13 other protein subunits of Mediator complex, including Med1, Med14, and Med25, as an interactor of NS1 (Citation100), one using three different screens (BioID, MAPPIT, and KISS) (Citation101), and a yeast two-hybrid screen (Citation102).
Consistent among these studies, are findings indicating that NS1 partially localizes in the nucleus. However, it was not clear whether this occurs in the context of hRSV infection. A recent study demonstrated that NS1 is present in the cytoplasm and in the nucleus of primary human tracheobronchial epithelial cells (hTBECs) when infected with hRSV (Citation100). This was further supported in hRSV infected human monocytic-derived dendritic cells as well as in the A549 cell line (Citation100). Moreover, when airway epithelial cells were treated with KPT-335, an inhibitor of exportin XPO1-mediated cargo transport from the nucleus, NS1 accumulated in the nucleus, suggesting that NS1 is not diffusing through the nuclear pore (Citation100). NS1 was found to be chromatin associated, and chromatin immunoprecipitation revealed that NS1 binds to regulatory elements (specifically promoters and enhancers) along with Mediator complex and suppresses the transcription of interferon stimulated genes, including IFIT2, IFIT3, and OAS1 (Citation100). This finding is consistent with earlier observations that suppression of antiviral gene expression is mediated by NS1 (Citation96, Citation97, Citation103, Citation104). For example, NS1 may potentially inhibit JAK/STAT signaling by upregulating the gene expression of the suppressor of cytokine signaling proteins that are negative feedback inhibitors of JAK activity (Citation103). Transfection of A549 cells with NS1, and in combination with NS2, appears to increase suppressor of cytokine signaling 1 and 3 (SOCS1 and SOCS3) expression, leading to lower levels of antiviral 2,5-OAS1 and MxA expression (Citation105–107). Furthermore, NS1 appears to interfere with SOD2 expression in response to hRSV infection (Citation103). SOD2 is a mitochondrial protein that is upregulated in response to oxidative stress stimulated by viral infection to promote cell survival and viral pathogenesis. While NS1 does not appear to directly bind to SOD2, infection of A549 cells with a recombinant hRSV virus lacking NS1 results in higher expression of SOD2 compared to mock infected cells, suggesting that NS1 modulates SOD2 expression.
Structural basis for NS1 function
Biochemical and biophysical characterization of NS1 have started to elucidate the structural basis for the many roles of NS1. Several studies revealed that NS1 behaves as a monomer in solution (Citation63, Citation78, Citation97, Citation108). However, other studies noted a potential for NS1 to form dimers (Citation78). The X-ray crystal structure of recombinant NS1 showed that NS1 is a monomer comprised of a beta sandwich flanked by three alpha helices () (Citation97). Notably, the third alpha helix appears to be extended away from the core of the protein, suggesting some conformational flexibility and perhaps explaining its potential to oligomerize and aggregate (Citation109). However, this alpha helix also contains several hydrophobic residues that are solvent accessible in the crystal structure and that is more likely to be involved in protein-protein interactions. Atomistic computer simulations also suggest that this C-terminal helix is more stable in the context of the NS1 structure than the isolated helix alone (Citation97). The 4 terminal Asp-Leu-Asn-Pro residues in NS1 that is common to both NS1 and NS2 are unstructured. A search for structural homologs revealed that NS1 adopts a similar structural fold to the N-terminal domain of the RSV matrix protein, with a backbone RMSD of 4.15 Å over 88 residues, despite having low sequence similarity (Citation97). NS1 contains an additional strand in the beta sandwich as well as an additional alpha helix at its C-terminus that is lacking in either subdomain of the hRSV matrix (M) protein (Citation110). Interestingly, prior studies of M protein showed that M partially localizes to the nucleus early during hRSV infection (Citation34, Citation111–113) like many matrix proteins from other negative strand RNA viruses (Citation114–116). Given the similarity in structure to hRSV M, it is not clear if the NS1 core is important for mediating entry into the nucleus since NS1 is lacking a canonical nuclear localization sequence. Mutation in the C-terminal helix does not affect nuclear localization of NS1 (Citation100).
However, mutational studies on the NS1 C-terminal helix did result in a loss of IFNβ inhibition in vitro as well as a loss of suppression of dendritic cell maturation (Citation97), suggesting that this unique helix is critical for NS1-mediated IFN antagonism and modulation of immune responses. Recombinant viruses containing NS1 mutations in the C-terminal helix displayed attenuated viral replication rates in A549 cells and resulted in significant changes in gene expression when compared to WT hRSV infection (Citation97, Citation117, Citation118). RNA-seq analysis of A549 cells transfected with the NS1 C-terminal helix Y125A mutant revealed significantly lower levels of gene expression of several antiviral genes, including IFIT2, IFIT3, IRF2, ISG20, and OAS1 (Citation100). While ChIP-qPCR showed that the NS1 Y125A mutant bound to Mediator complex at levels comparable to NS1 wildtype (WT), NS1 Y125A also displayed reduced capacity to inhibit ISRE reporter activity in vitro (Citation100). Collectively, results from these studies suggest that NS1 likely displaces one or more transcription factors that interact with Mediator complex to regulate transcription of antiviral gene expression and that this is mediated through interactions with the NS1 C-terminal helix. Interestingly, NS1 may be similar to other viral factors that target Mediator to stimulate transcriptional activation of viral genes (Citation119–121). Additional studies support that the NS1 C-terminal helix binds directly to Med25 and prevents interactions between transcriptional activators and Mediator complex in vitro (Citation100–102). The mechanistic details of this interaction or with other Mediator subunits have yet to be determined. However, these findings do not also rule out the possibility that other regions in NS1 are important for facilitating additional interactions given its multifunctional nature.
NS2-mediated inhibition of the IFN signaling pathway
Like NS1, NS2 is a small nonstructural protein that functions to suppress IFN signaling. Early studies showed that recombinant viruses where the NS2 gene is deleted (𝚫NS2) are viable but have slower growth kinetics, suggesting that NS2 is not critical for hRSV replication (Citation71). Infection of cells with recombinant 𝚫NS2 deletion viruses resulted in increased IFNβ mRNA levels; however, the levels were not as high as compared to a recombinant virus with a 𝚫NS1 gene deletion or 𝚫NS1/𝚫NS2 gene deletions (Citation69–72). Altogether, these results suggested that NS2 may be functionally redundant with NS1 and may not be as potent of an IFN antagonist compared to NS1.
Subsequent studies demonstrated that NS2 targets different molecules in the IFNβ signaling pathway than NS1. In airway epithelial cells, RSV infection induces the expression of the PRR RIG-I during the early phase of infection. siRNA knockdown of RIG-I inhibited the subsequent activation of transcription factors IRF3 and (Citation122). Expression of NS2 inhibits RIG-I mediated IRF3 activation when stimulated with a 5’ppp RNA (Citation64). Subsequent coimmunoprecipitation experiments revealed that NS2 can bind directly to RIG-I, likely through interactions with the N-terminal CARD domains (Citation64, Citation123). While RIG-I appears to have a greater role in response to hRSV infection, more recent studies suggest that the other cytosolic RIG-I-like receptor MDA5 may also contribute to host responses. MDA5 expression appears to be upregulated in hRSV-infected infants (Citation124) and MDA5 colocalizes with MAVS and hRSV genomic RNA (Citation125). Consistent with this, NS2 was also shown to bind to MDA5 through the N-terminal CARDs in coimmunoprecipitation and in vitro pulldown assays (Citation123). However, there is no binding to MAVS or the MAVS CARD, suggesting that NS2 prevents IFN signaling at the level of the PRRs (Citation123). Additional studies demonstrated that NS2 binding reduces the overall levels of ubiquitination of RIG-I and MDA5, indicating that NS2 binding prevents RLR activation and signaling () (Citation123).
NS2 also targets molecules downstream of the PRRs. Expression of NS2 decreased STAT2 expression levels when transfected into human tracheobronchial epithelial cells (Citation93, Citation126). Furthermore, RNAi of NS2 expression in cells infected with RSV resulted in a loss of STAT2 inhibition, supporting a role of NS2 in STAT2 degradation that may be mediated by the N- and C-termini of NS2 (Citation67). Moreover, a 𝚫NS2 deletion virus lost the ability to suppress expression of STAT2 and PKR, an antiviral gene, when compared to WT or a 𝚫NS1 deletion virus (Citation93). This suggests that STAT2 inhibition is uniquely attributed to NS2. Interestingly, introduction of select mutations into NS2 and recombinant hRSVs resulted in an overall decrease in cellular ubiquitination, including of STAT2 proteins. In addition, these NS2 mutations resulted in a recombinant hRSV that is attenuated with decreased replication and growth kinetics in A549 cells, indicating a potential correlation between NS2 expression, ubiquitination, and antagonism of innate immune signaling that contributes to viral replication. (Citation94). NS2 may also contribute to the degradation of STAT2 as part of a larger complex along with NS1 (Citation67, Citation88) and with other host proteins, such as MAP1B, an adaptor and scaffold protein (Citation67). The molecular mechanisms through which NS2 mediates these functions require further investigation.
Structural basis for NS2 function
Based upon sequence alignments of the hRSV nonstructural proteins, there is little sequence similarity between NS2 and NS1, which is consistent with NS2 displaying functions that are distinct from NS1. Early biochemical characterization of NS2 revealed that recombinantly expressed NS2 behaved primarily as a monomeric protein that was unstable with a short half-life (Citation78). However, a more recent study demonstrated that recombinantly expressed NS2 is well-behaved and can be purified to homogeneity (Citation123). Recombinant NS2 exists as a monomer in solution when analyzed by size-exclusion chromatography coupled to multi-angle light scattering. Using this material, the X-ray crystal structure of NS2 was solved to 2.8 Å resolution (). This structure revealed that NS2 is comprised of a single globular domain with a mixed alpha/beta fold formed by four alpha helices followed by a three-stranded, antiparallel beta sheet (Citation123). Comparison of the NS2 structure to other available structures in the Protein Data Bank (PDB) did not yield any hits with significant structural homology, including hRSV NS1. The C-terminal Asp-Leu-Asn-Pro motif that is common between the two nonstructural proteins are missing electron density, suggesting that this region is disordered and/or dynamic. Further analysis using hydrogen-deuterium exchange coupled to mass-spectrometry (HDX-MS) revealed that much of the NS2 structure also appears to be conformationally dynamic (Citation123). High levels of deuterium uptake are observed for a significant portion of the molecule, particularly at the N-terminus and loops connecting secondary structural elements. The N-terminal region of NS2 becomes protected from deuterium exchange upon bind to the RIG-I CARD domains. These results were validated through mutational analysis of the NS2 N-terminal residues. NS2 mutants display a loss of binding to RIG-I and reduced suppression of IFNβ mRNA levels (Citation123). Thus, one mechanism by which NS2 inhibits IFN signaling is by binding the PRRs RIG-I and MDA5 and preventing their activation and sustained downstream signaling, effectively limiting host immune responses to hRSV infection.
Additional roles of NS proteins in RSV infection
While activities related to IFN inhibition by the hRSV NS proteins have been well documented, the NS proteins are multifunctional and have additional roles beyond antagonism of innate immune responses. Deletion of the NS1 gene, either alone or in combination with the NS2 gene, was associated with reduced virus replication (Citation68–72). A subsequent study revealed that substitution of NS1 and NS2 with parainfluenza virus 5V protein cannot replace the functions of NS1 and NS2 in viral replication even in IFN-deficient Vero cells, indicating that NS1 and/or NS2 are important for viral replication (Citation127). The importance of the NS proteins in facilitating viral replication may be due in part to a role in inhibiting apoptosis and activating pro-survival pathways such as PI3K/AKT. Knockdown of one or both RSV NS proteins by siRNA promoted early apoptosis and NS-dependent suppression of apoptosis was observed in Vero cells, indicating that such inhibition is not related to type I IFN production (Citation128). In addition, the NS proteins suppressed TNF-induced apoptosis, which enhanced RSV growth (Citation128). RSV NS1 may also limit apoptosis by suppressing miR-24 expression, thereby inducing the transcription factor KLF6 expression to promote TGF-β-mediated cell cycle arrest, which facilitates RSV replication (Citation129).
The nonstructural proteins also have roles in modulating host adaptive immunity. Infection with 𝚫NS2 deletion or 𝚫NS1/2 deletion RSVs results in increased levels of hRSV-specific pulmonary CD8(+) cytotoxic T lymphocytes, indicating that the NS2 protein, and not NS1, suppresses viral replication and the cytotoxic T cell response through inhibition of type I IFN responses in a mouse model (Citation68). Furthermore, autophagy in dendritic cells (DC) may play a role in hRSV infection that is facilitated by NS2 through interactions with Beclin-1 (Citation130). In contrast, other studies suggest that NS1 protein inhibits dendritic cell (DC) maturation as 𝚫NS1 deletion or 𝚫NS1/2 deletion RSVs or mutation of NS1 protein results in increased expression levels of DC maturation markers and cytokines (Citation97, Citation131). Decreased antigen presentation and T-lymphocyte activation may be related to the IFN antagonism mediated by the NS1 protein (Citation131). However, subsequent studies demonstrated that NS1 protein suppresses activation and proliferation of CD103+ CD8+ T cells and affects the polarization of CD4+ T cells by co-cultivation of human DC and T cells. These effects were found to be associated with reduced maturation of dendritic cells caused by the NS1 protein and not due to suppressed IFN production, suggesting that the NS proteins have effects on the adaptive immune response independent on their IFN antagonist activities (Citation132).
Interestingly, NS2 has also been implicated in promoting epithelial cell shedding of RSV infected ciliated cells in the airway epithelium, perhaps through interactions with the cytoskeleton, leading to distal airway obstruction (Citation2). These results suggest that NS2 may play a role in promoting airway obstruction and therefore enhanced spread of infection, although the precise mechanisms by which NS2 protein mediates this remain to be defined (Citation2).
Vaccines
Early studies in the 1960s using a vaccine containing formalin-inactivated RSV failed to protect young children against natural exposure to hRSV infection and resulted in enhanced disease (Citation133–135), which hampered the development of vaccines to hRSV. Subsequent attempts focused on developing live-attenuated vaccines, including cold-passaging or chemical mutagenesis, but were found to have varying levels of attenuation in naïve or exposed children and adults making this method of vaccine development unreliable (Citation136). Additional attempts with the advent of reverse genetics for hRSV (Citation137–139) included gene deletions of NS1 and/or NS2 (and other genes such as M2-2) to elicit increased IFN responses by removal of their IFN antagonist activities. Not surprisingly, these deletion viruses resulted in over attenuated RSVs that failed to replicate sufficiently and elicit a durable response. Many advancements have been made since these initial attempts 60 years ago that have emerged from an increased understanding of the structure and function of F. As neutralizing antibodies target the prefusion conformation of F, this conformation has been the focus of several vaccine developments (Citation13, Citation14, Citation140–142), including particle-based and maternal vaccines. The recent FDA approved Arexvy uses a prefusion conformation of F that is stabilized by mutations as a particle-based vaccine for older adults; other vaccines in late-stage clinical trials also use a similar platform. While protection is now available for older adults and likely for children under 6 months of age (through maternal vaccination), this still leaves a gap in protection for children between 6 months and 5 years of age. Because particle-based vaccines may lead to vaccine enhanced disease in young children, additional studies and development of other modalities, including RNA and live-attenuated vaccines, are needed. Recent advances in our understanding of multifunctional nature of NS1 and NS2 coupled to the availability of reverse genetics systems for RSV provides additional opportunities to develop new tools to enhance our understanding of hRSV infections and to better assess the efficacy of candidates. The X-ray crystal structures of NS1 and NS2 provide high resolution insights into the molecular basis for viral-host interactions. Use of these detailed structural descriptions and defined immune evasion mechanisms presents an opportunity for rational approach to identifying critical interfaces or residues on NS1 and NS2 that are important for modulating host responses. This also provides an alternative method of fine-tuning attenuation by combining mutations in RSV vaccine candidates while maintaining a desired level of immunogenicity and that can be tested in different infection models. Thus, having multiple strategies will provide many advantages towards developing protection and reducing the global morbidity and mortality burden of hRSV infections.
Availability of data
Data sharing is not applicable to this article as no new data were created or analyzed in this study.
Author contributions
D.W.L. wrote the initial draft with input from T.N.M. and J.P. T.N.M., J.P., and D.W.L. edited the final draft.
Figure 1. hRSV genome and viral proteins. Organization of the hRSV RNA genome (A2 strain). The viral genome contains 10 genes encoding for 11 proteins. The M2 gene contains two ORFs encoding for M2-1 and M2-2 proteins. Shown above and below the genome are representative structures available for the different hRSV proteins (NS1, PDB 5VJ2; NS2, PDB 7LDK; N, PDB 2WJ8; P, PDB 6PZK; M, PDB 2QP; SH, PDB 2NB7 and 2NB8; G, PDB 6BLH; F, PDB 4JHW and 3RRR; M2-1, PDB 4C3D; and L, 6PZK). For SH, NMR coordinates are not available for the transmembrane helix and is represented as a rounded rectangle. NS1, nonstructural protein 1; NS2, nonstructural protein 2; N, nucleoprotein; P, phosphoprotein; M, matrix; SH, small hydrophobic; G, glycoprotein; F, fusion glycoprotein; M2-1, transcription processivity factor; M2-2, replication co-factor; L, large polymerase.
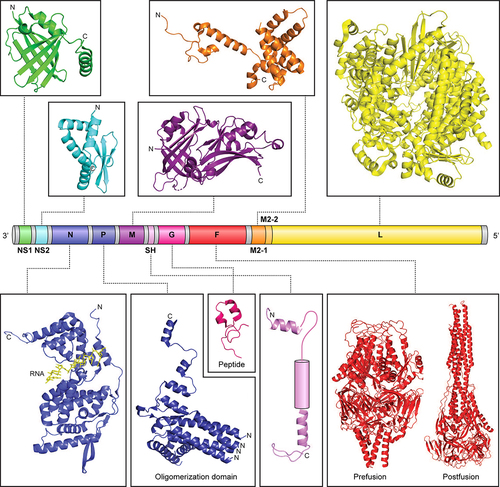
Figure 2. hRSV replication cycle. Simplified schematic of the different stages of the viral replication cycle. 1, Several host factors are involved in attachment of hRSV to the host membrane that is cell type dependent. 2, Entry may involve endocytosis or micropinocytosis of the virion followed by fusion of viral and host cell membranes. 3, Replication and transcription occurs in inclusion bodies where the negative strand genome serves as template for generating the antigenome. The negative strand genome is also transcribed by a sequential start-stop mechanism that results in a gradient of mRNAs from the 3’ to the 5’ end. 4, After viral proteins are generated, some like NS1 and M partially localize to the nucleus and are assembled along with the viral genome to produce new infectious viral particles.
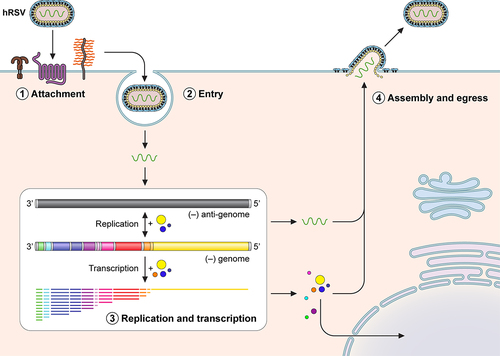
Figure 3. NS1 and NS2 antagonize multiple targets in the IFNα/β signaling pathway. Simplified representation of the IFNα/β induction and response signaling pathway. Cytosolic pattern recognition receptors, including RIG-I and MDA5, detect viral pathogen associated molecular patterns and stimulate the induction of IFNα/β. IFNα/β stimulates other downstream signaling pathways to activate the transcription of IFN-stimulated genes (ISG). hRSV NS1 and NS2 inhibit the function of different molecules at points indicated on the pathway. 5’OH, 5’-hydroxyl; 5’ppp, 5’-triphosphate; 5’m7Gppp, 5’-7-methylguanosine cap; PP1α/γ, protein phosphatase 1α/γ; RIG-I, retinoic inducible gene-I; MDA5, melanoma differentiation-associated protein 5; TRIM25, tripartite motif-containing protein 25; PACT, protein activator of PKR; LGP2, laboratory of genetics and physiology 2; MAVS, mitochondrial antiviral-signaling protein; NF-κB, nuclear factor kappa-light-chain-enhancer of activated B cells; TRAF3, TNF receptor associated factor 3; TANK, TRAF family member-associated NF-κB activator; IκB, inhibitor of NF-κB; IKK, IκB kinase; TBK-1, TANK-binding kinase 1; IRF, interferon regulatory factor; IFN, interferon; IFNAR1/2, IFN alpha and beta receptor subunit 1/2; JAK1, Janus kinase1; Tyk2, non-receptor tyrosine kinase 2; STAT, signal transducer and activator or transcription; ISRE, IFN stimulated response elements; Ub, ubiquitin; P, phosphorylated.
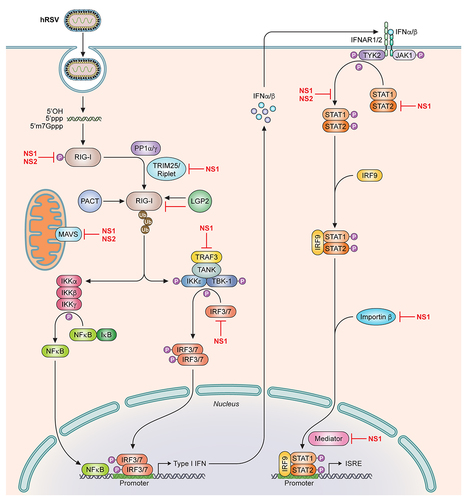
Acknowledgements
We thank J. Sullivan, D. DeFord, and members of the Leung and Amarasinghe laboratories for support. We acknowledge support from NIH R01AI140758 and R01AI159678 to D.W.L.
Additional information
Funding
References
- Johnson JE, Gonzales RA, Olson SJ, et al. 2007. The histopathology of fatal untreated human respiratory syncytial virus infection. Mod Pathol 20:108–119. 1 10.1038/modpathol.3800725
- Liesman RM, Buchholz UJ, Luongo CL, et al. 2014. RSV-encoded NS2 promotes epithelial cell shedding and distal airway obstruction. J Clin Invest 124:2219–2233. 5 10.1172/JCI72948
- Linder KA, Malani PN 2017. Respiratory Syncytial Virus. JAMA 317:98. 1 10.1001/jama.2016.17882
- McLaughlin JM, Khan F, Schmitt HJ, Agosti Y, Jodar L, Simoes EAF, Swerdlow DL. 2022. Respiratory Syncytial Virus-Associated Hospitalization Rates among US Infants: A Systematic Review and Meta-Analysis. J Infect Dis 225:1100–1111.
- Branche AR, Saiman L, Walsh EE, Falsey AR, Sieling WD, Greendyke W, Peterson DR, Vargas CY, Phillips M, Finelli L. 2022. Incidence of Respiratory Syncytial Virus Infection Among Hospitalized Adults, 2017-2020. Clin Infect Dis 74:1004–1011.
- Falsey AR, Hennessey PA, Formica MA, et al. 2005. Respiratory syncytial virus infection in elderly and high-risk adults. N Engl J Med 352:1749–1759. 17 10.1056/NEJMoa043951
- Hall CB, Weinberg GA, Iwane MK, et al. 2009. The burden of respiratory syncytial virus infection in young children. N Engl J Med 360:588–598. 6 10.1056/NEJMoa0804877
- Hansen CL, Chaves SS, Demont C, et al. 2022. Mortality Associated With Influenza and Respiratory Syncytial Virus in the US, 1999-2018. JAMA Netw Open 5:e220527. 2 10.1001/jamanetworkopen.2022.0527
- Matias G, Taylor R, Haguinet F, Schuck-Paim C, Lustig R, Shinde V. 2014. Estimates of mortality attributable to influenza and RSV in the United States during 1997-2009 by influenza type or subtype, age, cause of death, and risk status. Influenza Other Respir Viruses 8:507–15.
- Rha B, Curns AT, Lively JY, Campbell AP, Englund JA, Boom JA, Azimi PH, Weinberg GA, Staat MA, Selvarangan R, Halasa NB, McNeal MM, Klein EJ, Harrison CJ, Williams JV, Szilagyi PG, Singer MN, Sahni LC, Figueroa-Downing D, McDaniel D, Prill MM, Whitaker BL, Stewart LS, Schuster JE, Pahud BA, Weddle G, Avadhanula V, Munoz FM, Piedra PA, Payne DC, Langley G, Gerber SI. 2020. Respiratory Syncytial Virus-Associated Hospitalizations Among Young Children: 2015-2016. Pediatrics 146.
- Thompson WW, Shay DK, Weintraub E, et al. 2003. Mortality associated with influenza and respiratory syncytial virus in the United States. JAMA 289:179–186. 2 10.1001/jama.289.2.179
- Widmer K, Zhu Y, Williams JV, et al. 2012. Rates of hospitalizations for respiratory syncytial virus, human metapneumovirus, and influenza virus in older adults. J Infect Dis 206:56–62. 1 10.1093/infdis/jis309
- Mazur NI, Higgins D, Nunes MC, Melero JA, Langedijk AC, Horsley N, Buchholz UJ, Openshaw PJ, McLellan JS, Englund JA, Mejias A, Karron RA, Simoes EA, Knezevic I, Ramilo O, Piedra PA, Chu HY, Falsey AR, Nair H, Kragten-Tabatabaie L, Greenough A, Baraldi E, Papadopoulos NG, Vekemans J, Polack FP, Powell M, Satav A, Walsh EE, Stein RT, Graham BS, Bont LJ, Respiratory Syncytial Virus Network F. 2018. The respiratory syncytial virus vaccine landscape: lessons from the graveyard and promising candidates. Lancet Infect Dis 18:e295–e311.
- Mazur NI, Terstappen J, Baral R, et al. 2023. Respiratory syncytial virus prevention within reach: the vaccine and monoclonal antibody landscape. Lancet Infect Dis 23:e2–e21. 1 10.1016/S1473-3099(22)00291-2
- Ke Z, Dillard RS, Chirkova T, et al. 2018. The Morphology and Assembly of Respiratory Syncytial Virus Revealed by Cryo-Electron Tomography. Viruses 10. 8 446 10.3390/v10080446
- Bachi T 1988. Direct observation of the budding and fusion of an enveloped virus by video microscopy of viable cells. J Cell Bio 107:1689–1695. 5 10.1083/jcb.107.5.1689
- Bachi T, Howe C 1973. Morphogenesis and ultrastructure of respiratory syncytial virus. J Virol 12:1173–1180. 5 10.1128/jvi.12.5.1173-1180.1973
- Santangelo PJ, Bao G 2007. Dynamics of filamentous viral RNPs prior to egress. Nucleic Acids Res 35:3602–3611. 11 10.1093/nar/gkm246
- Chanock R, Finberg L 1957. Recovery from infants with respiratory illness of a virus related to chimpanzee coryza agent (CCA). II. Epidemiologic aspects of infection in infants and young children. Am j hyg 66:291–300. 3 10.1093/oxfordjournals.aje.a119902
- Chanock RM 1957. Recovery of a new type of myxovirus from infants with croup. Ann N Y Acad Sci 67:287–295. 8 10.1111/j.1749-6632.1957.tb46052.x
- Rima B, Collins P, Easton A, Fouchier R, Kurath G, Lamb RA, Lee B, Maisner A, Rota P, Wang L, Ictv Report C. 2017. ICTV Virus Taxonomy Profile: Pneumoviridae. J Gen Virol 98:2912–2913.
- Galloux M, Risso-Ballester J, Richard CA, Fix J, Rameix-Welti MA, Eleouet JF. 2020. Minimal Elements Required for the Formation of Respiratory Syncytial Virus Cytoplasmic Inclusion Bodies In Vivo and In Vitro. mBio 11.
- Risso-Ballester J, Galloux M, Cao J, et al. 2021. A condensate-hardening drug blocks RSV replication in vivo. Nature 595:596–599. 7868 10.1038/s41586-021-03703-z
- Cao D, Gao Y, Roesler C, et al. 2020. Cryo-EM structure of the respiratory syncytial virus RNA polymerase. Nat Commun 11:368. 1 10.1038/s41467-019-14246-3
- Gilman MSA, Liu C, Fung A, Behera I, Jordan P, Rigaux P, Ysebaert N, Tcherniuk S, Sourimant J, Eleouet JF, Sutto-Ortiz P, Decroly E, Roymans D, Jin Z, McLellan JS. 2019. Structure of the Respiratory Syncytial Virus Polymerase Complex. Cell 179:193–204 e14.
- Te Velthuis AJW, Grimes JM, Fodor E 2021. Structural insights into RNA polymerases of negative-sense RNA viruses. Nat Rev Microbiol 19:303–318. 5 10.1038/s41579-020-00501-8
- Cao D, Gao Y, Liang B 2021. Structural Insights into the Respiratory Syncytial Virus RNA Synthesis Complexes. Viruses 13. 5 834 10.3390/v13050834
- Collins PL, Fearns R, Graham BS. 2013. Respiratory syncytial virus: virology, reverse genetics, and pathogenesis of disease. Curr Top Microbiol Immunol 372:3–38.
- Grosfeld H, Hill MG, Collins PL 1995. RNA replication by respiratory syncytial virus (RSV) is directed by the N, P, and L proteins; transcription also occurs under these conditions but requires RSV superinfection for efficient synthesis of full-length mRNA. J Virol 69:5677–5686. 9 10.1128/jvi.69.9.5677-5686.1995
- Fearns R, Collins PL 1999. Role of the M2-1 transcription antitermination protein of respiratory syncytial virus in sequential transcription. J Virol 73:5852–5864. 7 10.1128/JVI.73.7.5852-5864.1999
- Richard CA, Rincheval V, Lassoued S, et al. 2018. RSV hijacks cellular protein phosphatase 1 to regulate M2-1 phosphorylation and viral transcription. PLOS Pathog 14:e1006920. 3 10.1371/journal.ppat.1006920
- Rincheval V, Lelek M, Gault E, et al. 2017. Functional organization of cytoplasmic inclusion bodies in cells infected by respiratory syncytial virus. Nat Commun 8:563. 1 10.1038/s41467-017-00655-9
- Blanchard EL, Braun MR, Lifland AW, et al. 2020. Polymerase-tagged respiratory syncytial virus reveals a dynamic rearrangement of the ribonucleocapsid complex during infection. PLOS Pathog 16:e1008987. 10 10.1371/journal.ppat.1008987
- Ghildyal R, Mills J, Murray M, et al. 2002. Respiratory syncytial virus matrix protein associates with nucleocapsids in infected cells. J Gen Virol 83:753–757. 4 10.1099/0022-1317-83-4-753
- Mitra R, Baviskar P, Duncan-Decocq RR, Patel D, Oomens AG. 2012. The human respiratory syncytial virus matrix protein is required for maturation of viral filaments. J Virol 86:4432–43.
- Fretaud M, Descamps D, Laubreton D, et al. 2021. New Look at RSV Infection: Tissue Clearing and 3D Imaging of the Entire Mouse Lung at Cellular Resolution. Viruses 13. 2 201 10.3390/v13020201
- Zhang L, Peeples ME, Boucher RC, et al. 2002. Respiratory syncytial virus infection of human airway epithelial cells is polarized, specific to ciliated cells, and without obvious cytopathology. J Virol 76:5654–5666. 11 10.1128/JVI.76.11.5654-5666.2002
- Feldman SA, Audet S, Beeler JA 2000. The fusion glycoprotein of human respiratory syncytial virus facilitates virus attachment and infectivity via an interaction with cellular heparan sulfate. J Virol 74:6442–6447. 14 10.1128/JVI.74.14.6442-6447.2000
- Techaarpornkul S, Collins PL, Peeples ME. 2002. Respiratory syncytial virus with the fusion protein as its only viral glycoprotein is less dependent on cellular glycosaminoglycans for attachment than complete virus. Virology 294:296–304. 2 10.1006/viro.2001.1340
- Johnson SM, McNally BA, Ioannidis I, et al. 2015. Respiratory Syncytial Virus Uses CX3CR1 as a Receptor on Primary Human Airway Epithelial Cultures. PLOS Pathog 11:e1005318. 12 10.1371/journal.ppat.1005318
- Tayyari F, Marchant D, Moraes TJ, et al. 2011. Identification of nucleolin as a cellular receptor for human respiratory syncytial virus. Nat Med 17:1132–1135. 9 10.1038/nm.2444
- Currier MG, Lee S, Stobart CC, et al. 2016. EGFR Interacts with the Fusion Protein of Respiratory Syncytial Virus Strain 2-20 and Mediates Infection and Mucin Expression. PLOS Pathog 12:e1005622. 5 10.1371/journal.ppat.1005622
- Griffiths CD, Bilawchuk LM, McDonough JE, et al. 2020. IGF1R is an entry receptor for respiratory syncytial virus. Nature 583:615–619. 7817 10.1038/s41586-020-2369-7
- Behera AK, Matsuse H, Kumar M, et al. 2001. Blocking intercellular adhesion molecule-1 on human epithelial cells decreases respiratory syncytial virus infection. Biochem Biophys Res Commun 280:188–195. 1 10.1006/bbrc.2000.4093
- Bukreyev A, Whitehead SS, Murphy BR, et al. 1997. Recombinant respiratory syncytial virus from which the entire SH gene has been deleted grows efficiently in cell culture and exhibits site-specific attenuation in the respiratory tract of the mouse. J Virol 71:8973–8982. 12 10.1128/jvi.71.12.8973-8982.1997
- Fuentes S, Tran KC, Luthra P, et al. 2007. Function of the respiratory syncytial virus small hydrophobic protein. J Virol 81:8361–8366. 15 10.1128/JVI.02717-06
- Gan SW, Tan E, Lin X, Yu D, Wang J, Tan GM, Vararattanavech A, Yeo CY, Soon CH, Soong TW, Pervushin K, Torres J. 2012. The small hydrophobic protein of the human respiratory syncytial virus forms pentameric ion channels. J Biol Chem 287:24671–89.
- Li Y, To J, Verdia-Baguena C, et al. 2014. Inhibition of the human respiratory syncytial virus small hydrophobic protein and structural variations in a bicelle environment. J Virol 88:11899–11914. 20 10.1128/JVI.00839-14
- Battles MB, McLellan JS 2019. Respiratory syncytial virus entry and how to block it. Nat Rev Microbiol 17:233–245. 4 10.1038/s41579-019-0149-x
- Hijano DR, Vu LD, Kauvar LM, et al. 2019. Role of Type I Interferon (IFN) in the Respiratory Syncytial Virus (RSV) Immune Response and Disease Severity. Front Immunol 10:566. 10.3389/fimmu.2019.00566
- Mukherjee S, Lukacs NW. 2013. Innate immune responses to respiratory syncytial virus infection. Curr Top Microbiol Immunol 372:139–54.
- Ivashkiv LB, Donlin LT 2014. Regulation of type I interferon responses. Nat Rev Immunol 14:36–49. 1 10.1038/nri3581
- Kawai T, Akira S 2006. Innate immune recognition of viral infection. Nat Immunol 7:131–137. 2 10.1038/ni1303
- Kawai T, Akira S. 2007. Antiviral signaling through pattern recognition receptors. J Biochem 141:137–45.
- Bowie AG, Unterholzner L 2008. Viral evasion and subversion of pattern-recognition receptor signalling. Nat Rev Immunol 8:911–922. 12 10.1038/nri2436
- Brennan K, Bowie AG 2010. Activation of host pattern recognition receptors by viruses. Curr Opin Microbiol 13:503–507. 4 10.1016/j.mib.2010.05.007
- Brubaker SW, Bonham KS, Zanoni I, et al. 2015. Innate immune pattern recognition: a cell biological perspective. Annu Rev Immunol 33:257–290. 1 10.1146/annurev-immunol-032414-112240
- Wu J, Chen ZJ 2014. Innate immune sensing and signaling of cytosolic nucleic acids. Annu Rev Immunol 32:461–488. 1 10.1146/annurev-immunol-032713-120156
- Leung DW 2019. Mechanisms of Non-segmented Negative Sense RNA Viral Antagonism of Host RIG-I-Like Receptors. J Mol Biol 431:4281–4289. 21 10.1016/j.jmb.2019.06.002
- Honda K, Takaoka A, Taniguchi T. 2006. Type I interferon [corrected] gene induction by the interferon regulatory factor family of transcription factors. Immunity 25:349–60.
- Pichlmair A, Reis e Sousa C. 2007. Innate recognition of viruses. Immunity 27:370–83.
- Stetson DB, Medzhitov R 2006. Type I interferons in host defense. Immunity 25:373–381. 3 10.1016/j.immuni.2006.08.007
- Ling Z, Tran KC, Arnold JJ, et al. 2008. Purification and characterization of recombinant human respiratory syncytial virus nonstructural protein NS1. Protein Expr Purif 57:261–270. 2 10.1016/j.pep.2007.09.017
- Ling Z, Tran KC, Teng MN 2009. Human respiratory syncytial virus nonstructural protein NS2 antagonizes the activation of beta interferon transcription by interacting with RIG-I. J Virol 83:3734–3742. 8 10.1128/JVI.02434-08
- Chambers R, Takimoto T 2009. Antagonism of innate immunity by paramyxovirus accessory proteins. Viruses 1:574–593. 3 10.3390/v1030574
- Messaoudi I, Amarasinghe GK, Basler CF 2015. Filovirus pathogenesis and immune evasion: insights from Ebola virus and Marburg virus. Nat Rev Microbiol 13:663–676. 11 10.1038/nrmicro3524
- Swedan S, Andrews J, Majumdar T, et al. 2011. Multiple functional domains and complexes of the two nonstructural proteins of human respiratory syncytial virus contribute to interferon suppression and cellular location. J Virol 85:10090–10100. 19 10.1128/JVI.00413-11
- Kotelkin A, Belyakov IM, Yang L, et al. 2006. The NS2 protein of human respiratory syncytial virus suppresses the cytotoxic T-cell response as a consequence of suppressing the type I interferon response. J Virol 80:5958–5967. 12 10.1128/JVI.00181-06
- Spann KM, Tran KC, Chi B, Rabin RL, Collins PL. 2004. Suppression of the induction of alpha, beta, and lambda interferons by the NS1 and NS2 proteins of human respiratory syncytial virus in human epithelial cells and macrophages [corrected]. J Virol 78:4363–9.
- Spann KM, Tran KC, Collins PL. 2005. Effects of nonstructural proteins NS1 and NS2 of human respiratory syncytial virus on interferon regulatory factor 3, NF-kappaB, and proinflammatory cytokines. J Virol 79:5353–62.
- Teng MN, Collins PL 1998. Identification of the respiratory syncytial virus proteins required for formation and passage of helper-dependent infectious particles. J Virol 72:5707–5716. 7 10.1128/JVI.72.7.5707-5716.1998
- Teng MN, Whitehead SS, Bermingham A, et al. 2000. Recombinant respiratory syncytial virus that does not express the NS1 or M2-2 protein is highly attenuated and immunogenic in chimpanzees. J Virol 74:9317–9321. 19 10.1128/JVI.74.19.9317-9321.2000
- Bossert B, Marozin S, Conzelmann KK 2003. Nonstructural proteins NS1 and NS2 of bovine respiratory syncytial virus block activation of interferon regulatory factor 3. J Virol 77:8661–8668. 16 10.1128/JVI.77.16.8661-8668.2003
- Schlender J, Bossert B, Buchholz U, et al. 2000. Bovine respiratory syncytial virus nonstructural proteins NS1 and NS2 cooperatively antagonize alpha/beta interferon-induced antiviral response. J Virol 74:8234–8242. 18 10.1128/JVI.74.18.8234-8242.2000
- Collins PL, Wertz GW. 1985. Nucleotide sequences of the 1B and 1C nonstructural protein mRNAs of human respiratory syncytial virus. Virology 143:442–451. 2 10.1016/0042-6822(85)90384-8
- Elango N, Satake M, Venkatesan S 1985. mRNA sequence of three respiratory syncytial virus genes encoding two nonstructural proteins and a 22K structural protein. J Virol 55:101–110. 1 10.1128/jvi.55.1.101-110.1985
- Johnson PR, Collins PL. 1989. The 1B (NS2), 1C (NS1) and N proteins of human respiratory syncytial virus (RSV) of antigenic subgroups A and B: sequence conservation and divergence within RSV genomic RNA. J Gen Virol 70 (Pt 6):1539–47.
- Evans JE, Cane PA, Pringle CR 1996. Expression and characterisation of the NS1 and NS2 proteins of respiratory syncytial virus. Virus Res 43:155–161. 2 10.1016/0168-1702(96)01327-5
- Brisse M, Ly H 2019. Comparative Structure and Function Analysis of the RIG-I-Like Receptors: RIG-I and MDA5. Front Immunol 10:1586. 10.3389/fimmu.2019.01586
- Thoresen D, Wang W, Galls D, et al. 2021. The molecular mechanism of RIG-I activation and signaling. Immunol Rev 304:154–168. 1 10.1111/imr.13022
- Wu B, Hur S 2015. How RIG-I like receptors activate MAVS. Curr Opin Virol 12:91–98. 10.1016/j.coviro.2015.04.004
- Ban J, Lee NR, Lee NJ, et al. 2018. Human Respiratory Syncytial Virus NS 1 Targets TRIM25 to Suppress RIG-I Ubiquitination and Subsequent RIG-I-Mediated Antiviral Signaling. Viruses 10 12 716 10.3390/v10120716
- Gack MU, Kirchhofer A, Shin YC, et al. 2008. Roles of RIG-I N-terminal tandem CARD and splice variant in TRIM25-mediated antiviral signal transduction. Proc Natl Acad Sci U S A 105:16743–16748. 43 10.1073/pnas.0804947105
- Gack MU, Shin YC, Joo CH, et al. 2007. TRIM25 RING-finger E3 ubiquitin ligase is essential for RIG-I-mediated antiviral activity. Nature 446:916–920. 7138 10.1038/nature05732
- Kowalinski E, Lunardi T, McCarthy AA, et al. 2011. Structural basis for the activation of innate immune pattern-recognition receptor RIG-I by viral RNA. Cell 147:423–435. 2 10.1016/j.cell.2011.09.039
- Boyapalle S, Wong T, Garay J, Teng M, San Juan-Vergara H, Mohapatra S, Mohapatra S., Tripp, R. 2012. Respiratory syncytial virus NS1 protein colocalizes with mitochondrial antiviral signaling protein MAVS following infection. PLoS One 7:e29386. 2 10.1371/journal.pone.0029386
- Wu W, Tran KC, Teng MN, et al. 2012. The interactome of the human respiratory syncytial virus NS1 protein highlights multiple effects on host cell biology. J Virol 86:7777–7789. 15 10.1128/JVI.00460-12
- Goswami R, Majumdar T, Dhar J, Chattopadhyay S, Bandyopadhyay SK, Verbovetskaya V, Sen GC, Barik S. 2013. Viral degradasome hijacks mitochondria to suppress innate immunity. Cell Res 23:1025–1042. 8 10.1038/cr.2013.98
- Ren J, Liu T, Pang L, et al. 2011. A novel mechanism for the inhibition of interferon regulatory factor-3-dependent gene expression by human respiratory syncytial virus NS1 protein. J Gen Virol 92:2153–2159. 9 10.1099/vir.0.032987-0
- Swedan S, Musiyenko A, Barik S 2009. Respiratory syncytial virus nonstructural proteins decrease levels of multiple members of the cellular interferon pathways. J Virol 83:9682–9693. 19 10.1128/JVI.00715-09
- Ramaswamy M, Shi L, Monick MM, et al. 2004. Specific inhibition of type I interferon signal transduction by respiratory syncytial virus. Am J Respir Cell Mol Biol 30:893–900. 6 10.1165/rcmb.2003-0410OC
- Elliott J, Lynch OT, Suessmuth Y, et al. 2007. Respiratory syncytial virus NS1 protein degrades STAT2 by using the Elongin-Cullin E3 ligase. J Virol 81:3428–3436. 7 10.1128/JVI.02303-06
- Ramaswamy M, Shi L, Varga SM, Barik S, Behlke MA, Look DC. 2006. Respiratory syncytial virus nonstructural protein 2 specifically inhibits type I interferon signal transduction. Virology 344:328–339. 2 10.1016/j.virol.2005.09.009
- Whelan JN, Tran KC, van Rossum DB, Teng MN. 2016. Identification of Respiratory Syncytial Virus Nonstructural Protein 2 Residues Essential for Exploitation of the Host Ubiquitin System and Inhibition of Innate Immune Responses. J Virol 90:6453–6463.
- Straub CP, Lau WH, Preston FM, et al. 2011. Mutation of the elongin C binding domain of human respiratory syncytial virus non-structural protein 1 (NS1) results in degradation of NS1 and attenuation of the virus. Virol J 8:252. 1 10.1186/1743-422X-8-252
- Dhar J, Cuevas RA, Goswami R, Zhu J, Sarkar SN, Barik S. 2015. 2’-5’-Oligoadenylate Synthetase-Like Protein Inhibits Respiratory Syncytial Virus Replication and Is Targeted by the Viral Nonstructural Protein 1. J Virol 89:10115–9.
- Chatterjee S, Luthra P, Esaulova E, et al. 2017. Structural basis for human respiratory syncytial virus NS1-mediated modulation of host responses. Nat Microbiol 2:17101. 9 10.1038/nmicrobiol.2017.101
- Munday DC, Emmott E, Surtees R, et al. 2010. Quantitative proteomic analysis of A549 cells infected with human respiratory syncytial virus. Mol & Cell Proteomics 9:2438–2459. 11 10.1074/mcp.M110.001859
- Tan YR, Peng D, Chen CM, et al. 2013. Nonstructural protein-1 of respiratory syncytial virus regulates HOX gene expression through interacting with histone. Mol Biol Rep 40:675–679. 1 10.1007/s11033-012-2107-9
- Pei J, Beri NR, Zou AJ, et al. 2021. Nuclear-localized human respiratory syncytial virus NS1 protein modulates host gene transcription. Cell Rep 37:109803. 2 10.1016/j.celrep.2021.109803
- Van Royen T, Sedeyn K, Moschonas GD, et al. 2022. An Unexpected Encounter: Respiratory Syncytial Virus Nonstructural Protein 1 Interacts with Mediator Subunit MED25. J Virol 96:e0129722. 19 10.1128/jvi.01297-22
- Dong J, Basse V, Bierre M, et al. 2022. Respiratory Syncytial Virus NS1 Protein Targets the Transactivator Binding Domain of MED25. J Mol Biol 434:167763. 19 10.1016/j.jmb.2022.167763
- Hastie ML, Headlam MJ, Patel NB, et al. 2012. The human respiratory syncytial virus nonstructural protein 1 regulates type I and type II interferon pathways. Mol & Cell Proteomics 11:108–127. 5 10.1074/mcp.M111.015909
- Ribaudo M, Barik S 2017. The nonstructural proteins of Pneumoviruses are remarkably distinct in substrate diversity and specificity. Virol J 14:215. 1 10.1186/s12985-017-0881-7
- Moore EC, Barber J, Tripp RA 2008. Respiratory syncytial virus (RSV) attachment and nonstructural proteins modify the type I interferon response associated with suppressor of cytokine signaling (SOCS) proteins and IFN-stimulated gene-15 (ISG15). Virol J 5:116. 1 10.1186/1743-422X-5-116
- Xu X, Zheng J, Zheng K, Hou Y, Zhao F, Zhao D. 2014. Respiratory syncytial virus NS1 protein degrades STAT2 by inducing SOCS1 expression. Intervirology 57:65–73. 2 10.1159/000357327
- Zheng J, Yang P, Tang Y, Pan Z, Zhao D. 2015. Respiratory Syncytial Virus Nonstructural Proteins Upregulate SOCS1 and SOCS3 in the Different Manner from Endogenous IFN Signaling. J Immunol Res 2015:738547.
- Pretel E, Camporeale G, de Prat-Gay G., Pastore, A. 2013. The non-structural NS1 protein unique to respiratory syncytial virus: a two-state folding monomer in quasi-equilibrium with a stable spherical oligomer. PLoS One 8:e74338. 9 10.1371/journal.pone.0074338
- Conci J, Alvarez-Paggi D, de Oliveira GAP, Pagani TD, Esperante SA, Borkosky SS, Aran M, Alonso LG, Mohana-Borges R, Prat-Gay G. 2019. Conformational Isomerization Involving Conserved Proline Residues Modulates Oligomerization of the NS1 Interferon Response Inhibitor from the Syncytial Respiratory Virus. Biochemistry 58:2883–2892.
- Forster A, Maertens GN, Farrell PJ, et al. 2015. Dimerization of matrix protein is required for budding of respiratory syncytial virus. J Virol 89:4624–4635. 8 10.1128/JVI.03500-14
- Ghildyal R, Ho A, Dias M, et al. 2009. The respiratory syncytial virus matrix protein possesses a Crm1-mediated nuclear export mechanism. J Virol 83:5353–5362. 11 10.1128/JVI.02374-08
- Ghildyal R, Ho A, Wagstaff KM, Dias MM, Barton CL, Jans P, Bardin P, Jans DA. 2005. Nuclear import of the respiratory syncytial virus matrix protein is mediated by importin beta1 independent of importin alpha. Biochemistry 44:12887–95.
- Li HM, Ghildyal R, Hu M, Tran KC, Starrs LM, Mills J, Teng MN, Jans DA. 2021. Respiratory Syncytial Virus Matrix Protein-Chromatin Association Is Key to Transcriptional Inhibition in Infected Cells. Cells 10. Cells 10 10 2786 10.3390/cells10102786
- He FB, Khan H, Huttunen M, Kolehmainen P, Melen K, Maljanen S, Qu M, Jiang M, Kakkola L, Julkunen I. 2021. Filovirus VP24 Proteins Differentially Regulate RIG-I and MDA5-Dependent Type I and III Interferon Promoter Activation. Front Immunol 12:694105.
- Wang YE, Park A, Lake M, et al. 2010. Ubiquitin-regulated nuclear-cytoplasmic trafficking of the Nipah virus matrix protein is important for viral budding. PLOS Pathog 6:e1001186. 11 10.1371/journal.ppat.1001186
- Xu W, Edwards MR, Borek DM, et al. 2014. Ebola virus VP24 targets a unique NLS binding site on karyopherin alpha 5 to selectively compete with nuclear import of phosphorylated STAT1. Cell Host Microbe 16:187–200. 2 10.1016/j.chom.2014.07.008
- Dave KA, Norris EL, Bukreyev AA, et al. 2014. A comprehensive proteomic view of responses of A549 type II alveolar epithelial cells to human respiratory syncytial virus infection. Mol & Cell Proteomics 13:3250–3269. 12 10.1074/mcp.M114.041129
- Martinez I, Lombardia L, Garcia-Barreno B, et al. 2007. Distinct gene subsets are induced at different time points after human respiratory syncytial virus infection of A549 cells. J Gen Virol 88:570–581. 2 10.1099/vir.0.82187-0
- Mittler G, Stuhler T, Santolin L, Uhlmann T, Kremmer E, Lottspeich F, Berti L, Meisterernst M. 2003. A novel docking site on Mediator is critical for activation by VP16 in mammalian cells. EMBO J 22:6494–504.
- Rovnak J, Quackenbush SL, Maury W 2022. Exploitation of the Mediator complex by viruses. PLOS Pathog 18:e1010422. 4 10.1371/journal.ppat.1010422
- Vojnic E, Mourao A, Seizl M, et al. 2011. Structure and VP16 binding of the Mediator Med25 activator interaction domain. Nat Struct Mol Biol 18:404–409. 4 10.1038/nsmb.1997
- Liu P, Jamaluddin M, Li K, et al. 2007. Retinoic acid-inducible gene I mediates early antiviral response and Toll-like receptor 3 expression in respiratory syncytial virus-infected airway epithelial cells. J Virol 81:1401–1411. 3 10.1128/JVI.01740-06
- Pei J, Wagner ND, Zou AJ, et al. 2021. Structural basis for IFN antagonism by human respiratory syncytial virus nonstructural protein 2. Proc Natl Acad Sci US A 118. 118 10 10.1073/pnas.2020587118
- Scagnolari C, Midulla F, Pierangeli A, et al. 2009. Gene expression of nucleic acid-sensing pattern recognition receptors in children hospitalized for respiratory syncytial virus-associated acute bronchiolitis. Clin Vaccine Immunol 16:816–823. 6 10.1128/CVI.00445-08
- Lifland AW, Jung J, Alonas E, et al. 2012. Human respiratory syncytial virus nucleoprotein and inclusion bodies antagonize the innate immune response mediated by MDA5 and MAVS. J Virol 86:8245–8258. 15 10.1128/JVI.00215-12
- Lo MS, Brazas RM, Holtzman MJ 2005. Respiratory syncytial virus nonstructural proteins NS1 and NS2 mediate inhibition of Stat2 expression and alpha/beta interferon responsiveness. J Virol 79:9315–9319. 14 10.1128/JVI.79.14.9315-9319.2005
- Tran KC, He B, Teng MN. 2007. Replacement of the respiratory syncytial virus nonstructural proteins NS1 and NS2 by the V protein of parainfluenza virus 5. Virology 368:73–82. 1 10.1016/j.virol.2007.06.017
- Bitko V, Shulyayeva O, Mazumder B, Musiyenko A, Ramaswamy M, Look DC, Barik S. 2007. Nonstructural proteins of respiratory syncytial virus suppress premature apoptosis by an NF-kappaB-dependent, interferon-independent mechanism and facilitate virus growth. J Virol 81:1786–95.
- Bakre A, Wu W, Hiscox J, Spann K, Teng MN, Tripp RA. 2015. Human respiratory syncytial virus non-structural protein NS1 modifies miR-24 expression via transforming growth factor-beta. J Gen Virol 96:3179–91.
- Chiok K, Pokharel SM, Mohanty I, Miller LG, Gao SJ, Haas AL, Tran KC, Teng MN, Bose S., Shenk, T. 2022. Human Respiratory Syncytial Virus NS2 Protein Induces Autophagy by Modulating Beclin1 Protein Stabilization and ISGylation. mBio 13:e0352821. 1 10.1128/mbio.03528-21
- Munir S, Le Nouen C, Luongo C, et al. 2008. Nonstructural proteins 1 and 2 of respiratory syncytial virus suppress maturation of human dendritic cells. J Virol 82:8780–8796. 17 10.1128/JVI.00630-08
- Munir S, Hillyer P, Le Nouen C, et al. 2011. Respiratory syncytial virus interferon antagonist NS1 protein suppresses and skews the human T lymphocyte response. PLOS Pathog 7:e1001336. 4 10.1371/journal.ppat.1001336
- Fulginiti VA, Eller JJ, Sieber OF, et al. 1969. Respiratory virus immunization. I. A field trial of two inactivated respiratory virus vaccines; an aqueous trivalent parainfluenza virus vaccine and an alum-precipitated respiratory syncytial virus vaccine. Am J Epidemiol 89:435–448. 4 10.1093/oxfordjournals.aje.a120956
- Kapikian AZ, Mitchell RH, Chanock RM, et al. 1969. An epidemiologic study of altered clinical reactivity to respiratory syncytial (RS) virus infection in children previously vaccinated with an inactivated RS virus vaccine. Am J Epidemiol 89:405–421. 4 10.1093/oxfordjournals.aje.a120954
- Kim HW, Canchola JG, Brandt CD, Pyles G, Chanock RM, Jensen K, Parrott RH. 1969. Respiratory syncytial virus disease in infants despite prior administration of antigenic inactivated vaccine. Am J Epidemiol 89:422–34.
- Karron RA, Buchholz UJ, Collins PL. 2013. Live-attenuated respiratory syncytial virus vaccines. Curr Top Microbiol Immunol 372:259–84.
- Collins PL, Hill MG, Camargo E, et al. 1995. Production of infectious human respiratory syncytial virus from cloned cDNA confirms an essential role for the transcription elongation factor from the 5’ proximal open reading frame of the M2 mRNA in gene expression and provides a capability for vaccine development. Proc Natl Acad Sci U S A 92:11563–11567. 25 10.1073/pnas.92.25.11563
- Moore ML, Chi MH, Luongo C, et al. 2009. A chimeric A2 strain of respiratory syncytial virus (RSV) with the fusion protein of RSV strain line 19 exhibits enhanced viral load, mucus, and airway dysfunction. J Virol 83:4185–94.
- Stobart CC, Hotard AL, Meng J, Moore ML. 2016. Reverse Genetics of Respiratory Syncytial Virus. Methods Mol Biol 1442:141–53.
- Gilman MS, Castellanos CA, Chen M, Ngwuta JO, Goodwin E, Moin SM, Mas V, Melero JA, Wright PF, Graham BS, McLellan JS, Walker LM. 2016. Rapid profiling of RSV antibody repertoires from the memory B cells of naturally infected adult donors. Sci Immunol 1.
- McLellan JS, Chen M, Leung S, et al. 2013. Structure of RSV fusion glycoprotein trimer bound to a prefusion-specific neutralizing antibody. Science 340:1113–1117. 6136 10.1126/science.1234914
- Ngwuta JO, Chen M, Modjarrad K, Joyce MG, Kanekiyo M, Kumar A, Yassine HM, Moin SM, Killikelly AM, Chuang GY, Druz A, Georgiev IS, Rundlet EJ, Sastry M, Stewart-Jones GB, Yang Y, Zhang B, Nason MC, Capella C, Peeples ME, Ledgerwood JE, McLellan JS, Kwong PD, Graham BS. 2015. Prefusion F-specific antibodies determine the magnitude of RSV neutralizing activity in human sera. Sci Transl Med 7:309ra162.