ABSTRACT
O’nyong-nyong virus (ONNV) is a neglected mosquito-borne alphavirus belonging to the Togaviridae family. ONNV is known to be responsible for sporadic outbreaks of acute febrile disease and polyarthralgia in Africa. As climate change increases the geographical range of known and potential new vectors, recent data indicate a possibility for ONNV to spread outside of the African continent and grow into a greater public health concern. In this review, we summarise the current knowledge on ONNV epidemiology, host–pathogen interactions, vector-virus responses, and insights into possible avenues to control risk of further epidemics. In this review, the limited ONNV literature is compared and correlated to other findings on mainly Old World alphaviruses. We highlight and discuss studies that investigate viral and host factors that determine viral-vector specificity, along with important mechanisms that determine severity and disease outcome of ONNV infection.
Introduction
Arthropod-borne viruses (arboviruses) are viruses transmitted by blood feeding insects. They represent a highly prevalent and significant category of established, emerging, and re-emerging pathogens worldwide. Notable examples of these viruses include dengue, yellow fever, Zika, Mayaro virus (MAYV), and Chikungunya virus (CHIKV) [Citation1]. During transmission, these viruses alternate between competent vertebrate hosts, competent vectors, and in some cases, zoonotic reservoir species. With 3.9 billion people living in at-risk subtropical and tropical climate regions, arboviruses pose a global threat that disproportionately burdens socioeconomically vulnerable communities [Citation2,Citation3]. Worryingly, the affected areas appear to be expanding with increased endemic transmissions being reported in countries such as France [Citation4] and Italy [Citation5].
In March 2022, the World Health Organization (WHO) launched the Global Arbovirus Initiative, a collaborative effort focusing on monitoring, preparing for, and reducing the occurrence of arbovirus outbreaks [Citation6,Citation7]. This was in response to the expanding distribution of vectors such as Aedes [Citation8], Culex [Citation9], and Anopheles [Citation10] mosquito genera, and the growing and impending threat they pose to global health. This expansion, exacerbated by climate change, increases the proximity between reservoir species and humans, increasing the opportunities for transmission to humans. With few effective licenced vaccines or FDA approved treatments for these diseases, improving arbovirus surveillance and investment in fundamental and translational research on these neglected tropical vector-borne diseases is sorely needed. One such under-studied arbovirus is the alphavirus, O’nyong-nyong virus (ONNV).
The alphavirus genus of the Togaviridae family encompasses a group of important arboviruses with a wide geographic distribution and therefore distinct evolutionary trajectories. ONNV is closely related to other alphaviruses in the Semliki Forest antigenic complex consisting of other members, such as the Semliki Forest virus (SFV), MAYV, and CHIKV. Originally, alphaviruses were divided into antigenic groups based on cross-reactivity from serological assays but are now organised into seven clades based on genomic sequence comparisons and divided into “Old World” and “New World” alphaviruses depending on their geographic distribution [Citation11]. Old world alphaviruses are primarily known for their characteristic arthralgia symptoms in contrast to the “New World” alphaviruses causing more neurovirulent pathologies [Citation12,Citation13].
The name “O’nyong-nyong” was coined by the Acholi people of East Africa to mean “very painful weakening of joints” [Citation14] and is reminiscent of the meaning of “Chikungunya” in the Kimakonde language (spoken in Tanzania and Mozambique), meaning “that which bends up.” Beyond a name describing their symptoms, these viruses share close genetic similarity. Comparisons between the CHIKV strain 37,997 and ONNV strain SG650 revealed a total of 89% of genome conservation, with a sequence identity ranging between 77% and 85% [Citation15]. In addition, phylogenetic analyses show a close evolutionary relationship between ONNV and CHIKV compared to other human-relevant alphaviruses (). Despite this genetic and geographic proximity, ONNV and CHIKV have unique competent vector species, with transmission occurring via anopheline and aedine mosquitoes, respectively.
Figure 1. Phylogenetic tree of ONNV and other related alphaviruses. Maximum likelihood phylogenetic tree generated using alphavirus E1 sequences obtained from NCBI databases (GenBank accession numbers included in each sequence name). Sequences were aligned with MUSCLE (v3.8.31) configured for highest accuracy (default settings), ambiguous regions were removed with Gblocks (v0.91b) and HKY85 substitution model used. Only approximate likelihood-ratio tests (aLrts) higher than 0.1 are displayed.
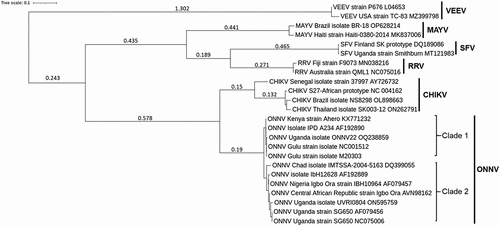
Epidemiology
The 1959 Uganda outbreak, where ONNV was first isolated, had more than 2 million cases and found ONNV spreading eastwards during its 3-year course [Citation14]. Since then, ONNV has been reported in the literature in multiple countries across West, East, and Central Africa (). In Africa, ONNV and CHIKV share overlapping epidemiological territories (at least 14 countries confirmed) stretching from Somalia in the east through to Senegal in the West () alongside many other well-known mosquito-borne diseases, such as Malaria and dengue, that manifest similar acute symptoms. This, combined with high serological cross reactivity to CHIKV, likely has led to the underrepresentation of reported ONNV cases, suggesting the virus has a much larger disease burden than recorded [Citation25]. Retrospective studies, including comparative plaque neutralisation assays between CHIKV and ONNV, have revealed a high incidence rate of 45% to 68% in the local populations [Citation22,Citation26]. More recent serology studies suggest current or recent circulation in coastal Kenya and Uganda [Citation24,Citation26].
Figure 2. Epidemiology of ONNV. Map depicting African territories with recorded outbreaks of CHIKV (coloured), ONNV (stripped) or both (coloured stripped). Individual studies reporting on ONNV outbreaks or endemic circulation are highlighted in number red dots, with details corresponding to the table provided. CHIKV endemic areas are taken from WHO ([Citation7]).
![Figure 2. Epidemiology of ONNV. Map depicting African territories with recorded outbreaks of CHIKV (coloured), ONNV (stripped) or both (coloured stripped). Individual studies reporting on ONNV outbreaks or endemic circulation are highlighted in number red dots, with details corresponding to the table provided. CHIKV endemic areas are taken from WHO ([Citation7]).](/cms/asset/791598a4-8351-4ece-89d9-843abcedfde4/kvir_a_2355201_f0002_oc.jpg)
Table 1. Reported and confirmed ONNV cases as of 2023.
Serological data collected during these outbreaks in Uganda and Kenya found that both Anopheles species: A. funestus and A. gambiae, were able to maintain ONNV for at least 20 and 13 days, respectively, identifying these mosquitoes as the primary vectors for this virus [Citation27]. Anopheline mosquitoes are known to be the vector for the Plasmodium parasite, thus it is unsurprising to find an overlapping distribution between O’nyong nyong fever and malaria [Citation28,Citation29]. Interestingly, a recent meta-analysis assessing potential malaria and CHIKV co-infection rates concluded that between 0% and 10% of CHIKV cases were co-infected with malaria [Citation30]. Curiously, all but one study in the meta-analysis were from African countries, and co-infection detection was higher when the diagnostic techniques used were serology based rather than nucleic acid based. Due to the serological cross-reactivity of the two alphaviruses [Citation23], it is plausible that ONNV could have been the co-infecting virus rather than CHIKV.
Co-infections with malaria and alphaviruses have potential implications for the epidemiology and surveillance of both diseases. Recent studies in mice showed that co-infection CHIKV-Plasmodium reduced CHIKV and malaria symptoms [Citation31], while ONNV-Plasmodium reduced ONNV pathology [Citation29]. This would contribute to the underestimation of malaria and alphavirus burden, since co-infected individuals would less likely be symptomatic. During the 1959–1962 epidemic, there was an unusual drop of malaria transmission [Citation32] which correlated with high O’nyong-nyong fever prevalence, suggesting that either co-transmission is unfavourable and unlikely in nature or that co-infection could reduce malaria burden during co-transmission [Citation29].
At the time of this review, ONNV has not been reported outside of Africa, with the exception of some sporadic imported cases in Germany [Citation33] and Canada [Citation17]. Fortunately, no local transmission of ONNV was reported following these importation events, but one such case or adaptation of the virus to a new local vector could trigger an explosive outbreak, exemplified by the events of the La Reunion epidemic that followed CHIKV adaptation to Aedes albopictus in 2005–2006 with a single alanine to valine mutation at position 226 in the E1 envelope glycoprotein [Citation34]. In addition, globalisation and climate change are altering the geographic distribution and ecological niche of virus compatible mosquito populations [Citation35], further increasing the chances of imported cases outside of Africa to establish local transmission events. To assess this risk, recent studies have started to evaluate the vector competence of non-African mosquitoes to ONNV and identified new potential vectors such as the South-East Asian Anopheles stephensi [Citation36]. The combination of these factors makes the spread of ONNV to other parts of the world a distinct possibility.
Transmission
Like most alphaviruses, ONNV alternates between arthropod and vertebrate hosts, establishing long-term persistent infections in mosquitoes and short-term acute infections in vertebrates following transmission via a blood meal [Citation27]. Interestingly, to date, no vertebrate host other than humans has been identified to carry ONNV in the wild [Citation37]. Other alphaviruses such as CHIKV and MAYV, however, have several natural reservoirs and maintain sylvatic transmission cycles in non-human monkeys and primates, respectively, indicating a possibility of an unidentified ONNV equivalent [Citation38–40].
ONNV was the only known mosquito-borne human alphaviral disease transmitted by Anopheles mosquitoes [Citation41,Citation42] until the recent study showing potential Mayaro virus transmission by anophelines [Citation43]. Specifically, its transmission is restricted to anopheline species such as Anopheles funestus, gambiae, and stephensi mosquitoes [Citation36,Citation44]. This raises interesting questions on vector competence and why ONNV is restricted to the African continent when anophelines are present worldwide (recently reviewed in [Citation15]). On the virus side, no clear evidence has emerged to ascertain a specific viral determinant that would associate with Anopheles transmission. A study showed that the full ONNV structural protein region was necessary to allow a CHIKV-ONNV chimera to successfully infect Anopheles mosquitoes [Citation45]. More recent chimera experiments have suggested that nsP3 specifically could be responsible for its vector specificity [Citation42]. The exact mechanism and function of the highly variable domain of nsP3 remains to be further elucidated, but it would be reasonable to hypothesize that differential host factor binding between CHIKV and ONNV could explain the observed phenotype [Citation42,Citation46].
Viral organisation and replication
Belonging to the alphavirus genus in the Togaviridae family, ONNV viral particles are enveloped with a 65–70 nm diameter size (). They contain a single-stranded, capped, and poly-adenylated positive-sense RNA genome of ~11.8 kb [Citation47,Citation48] (). Few studies have focused specifically on ONNV infection and replication mechanism, but we know that most alphaviridae have a similar replication cycle () since they share a highly conserved replication machinery.
Figure 3. Overview of ONNV. (a) TEM images of purified ONNV particles taken at 2 different magnifications (courtesy of Malleret, B. and Lee, E.Q.H.). (b) Schematic of the ONNV genome, which consists of a single-stranded positive sense RNA with 2 reading frames, the first encodes nsP1–4, this is followed by a sub genomic sequence that encodes for the viral structural proteins (Capsid, envelope 1–3 and membrane associated 6K protein). (c) Diagram describing the replication cycle of ONNV. Once ONNV releases its genetic material in the host cell, the nsP1–4 proteins are translated, nsP2 then proceeds to cleave the viral polymerase nsP4 which goes on to generate complementary negative RNA strands for rapid viral RNA replication. nsP2 then later cleaves both nsP1 and nsP3 to form the late viral replicase machinery needed for structural protein synthesis and virion assembly utilising host post-translational modifications via ER-golgi apparatus.
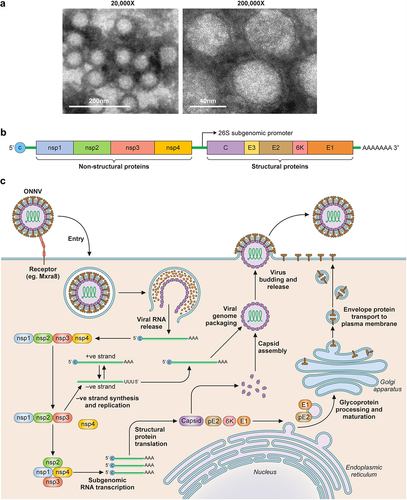
Alphaviruses, via their envelope proteins [Citation49–51], can use a wide range of host cell surface molecules for attachment and entry [Citation52]. Known examples of cellular attachment factors include heparan sulphate, which has been shown to impact the attachment of cell culture adapted CHIKV and SINV, as well as, naturally circulating Eastern Equine Encephalitis Virus (EEEV, strain FL91–4679) [Citation53,Citation54], C-type lectins [Citation55–57], or receptors such as the phosphatidylserine receptor TIM-1 [Citation58,Citation59] and matrix remodelling associated protein 8 (Mxra8) [Citation60] Zhang [Citation61]. This list has since expanded to include members of the low-density receptor (LDLR) family, including the low-density lipoprotein receptor class A domain containing 3 (LDLRAD3), very low-density lipoprotein receptor (VLDLR), and apolipoprotein E receptor 2 (ApoER2) for various alphaviruses such as VEEV, SFV, and SINV, but ONNV was not assessed in these studies [Citation62–65]. It should be noted that at the time of this review no specific receptor or host molecule has been shown to be solely responsible for alphavirus binding and/or entry. For example, HEK293T cells, despite lacking Mxra8 expression, remain highly permissible to several alphaviruses such as CHIKV (Western Africa strain 37,997 and clinical isolate Aruba -15,801,654), ONNV (Ahero strain), SINV (strain EgAr 339), VEEV (Trinidad Donkey strain), EEEV (strain H178/99), and WEEV (strain H160/99) [Citation66,Citation67]. In our hands, HEK293T are also highly permissive to CHIKV (La Reunion 2006-OPY1) and ONNV (isolate IMTSSA/5163) [Citation68,Citation69]. Though it must be noted that for these studies, infectious clone rescued viruses were amplified at least once through C6/36 or Vero cells prior to experimentation.
After viral attachment, entry occurs via receptor-mediated endocytosis and/or clathrin-mediated endocytosis [Citation60,Citation70] followed by membrane fusion during acidification of the endosome [Citation71–73]. This causes a conformational change in the virion envelope glycoproteins to initiate membrane fusion [Citation74,Citation75]. Crystal structure studies of Sindbis virus (SINV) E2-E1 proteins performed at low pH show that acidification results in the dissociation of the E2 protein from E1, allowing the E1 proteins to form homotrimers on the surface of the virus [Citation76]. These trimers then assemble to form ring-like structures that become a pore to facilitate membrane fusion [Citation77]. After membrane fusion, the capsid core can disassemble and release the viral genome in the cytoplasm where it can be translated as an mRNA.
Like other alphaviruses, ONNV genome contains two open reading frames (ORFs) (). The first ORF codes for components of the viral replicase, also called non-structural proteins (nsP), translated as an nsP1-to-3 (major product) or nsP1-to-4 (minor product) polyprotein by ribosomal read-through of an opal stop codon between nsP3 and nsP4, a process that seems to increase infectivity in anopheline mosquitoes [Citation78–80]. nsP2 is the viral protease and responsible for proteolytic cleavage in cis and trans of the viral non-structural polyprotein which can then assemble with viral mRNA and initiate replication [Citation81]. The resulting replicase complexes are then transported to the plasma membrane, resulting in invaginations called viral spherules that become viral replication factories producing genomic RNA of both sense and anti-sense, as well as, subgenomic RNA [Citation82]. The second open reading frame can then be translated from this subgenomic RNA, as a polyprotein that codes for the structural proteins consisting of either the viral capsid protein (CP), envelope proteins (E) E3, E2, 6K, and E1 or by ribosomal frameshift as a CP-E3-E2 TransFrame protein (TF) [Citation83], and allows for viral particle assembly at the plasma membrane.
Role of non-structural proteins
Molecular studies have teased out the specific and distinct roles these nsPs play during viral replication. The nsP1 protein is shown to function as a membrane anchor that tethers the replication complex in the viral “spherules” during replication at the plasma membrane [Citation84]. nsP2 is a viral protease that processes the nsP1234 polyprotein into its functional state [Citation78,Citation85] and a helicase for RNA replication [Citation86]. Further structural studies also suggest that CHIKV nsP2 can target small peptide substrates, indicating that it could potentially also interact with other host or viral proteins [Citation87]. The role of nsP3 is the least defined and conserved of the nsPs in the alphavirus proteome, though certain experiments have shown it is important for vector specificity [Citation42,Citation88], manipulating cellular responses through host protein interactions [Citation89] and enhancing viral RNA replication [Citation90]. Given the myriad of host proteins it is known to interact with, there is an invested interest in disrupting nsP3 as a potential anti-alphavirus target or to generate vaccine candidates (discussed below). Lastly, the nsP4 is a highly conserved RNA-dependent polymerase that replicates the viral RNA genome and maintains the 3' poly-A tail [Citation81,Citation91].
Clinical manifestation and pathogenicity
Once bitten by an infected mosquito, ONNV generally has an incubation period of 8 days before symptoms start to manifest () [Citation92]. Infections by ONNV are typically self-limiting and present with fever, rash, distinctive muscle and debilitating joint pain that can result in polyarthralgia. Interestingly, ONNV demonstrates no acute oedema in joint pathology [Citation93], which is unique from other related arthritogenic alphavirus such CHIKV [Citation94] and MAYV cases [Citation95]. Studies have, however, described lymphadenopathies as one of the other unique manifestations of ONNV infection [Citation14,Citation37,Citation96]. Due to a lack of longitudinal studies, the long-term impact of ONNV infection is difficult to evaluate, but chronic symptoms of arthritogenic alphaviruses are known to greatly impact quality of life and productivity due to long-term incapacitating polyarthralgia [Citation97,Citation98]. The recent CHIKV worldwide outbreak allowed researchers to better define long-term symptoms of CHIKV, with 60% of confirmed patients showing long-lasting arthritis symptoms that can last for years [Citation99].
Figure 4. Clinical manifestation of ONNV infection. Diagram showing the general disease progression of ONNV. After being bitten, the virus typically takes ~8 days before the patient becomes symptomatic. This progresses to the acute phase (days 8–15) with generalised symptoms such as fever, rashes, and headaches. In rare cases, ONNV infections will cause lymphoedema and retro-orbital pain, joint pain might also begin to manifest during this time. If not self-limiting, the disease will progress to enhanced pain and inflammation in the joints (targeting extremities) which in rare cases can last for several months (chronic phase).
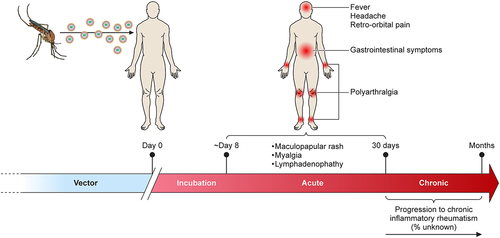
Encephalitis symptoms have not typically been linked with arthritogenic alphaviruses in the past; however, they should not be underestimated. CHIKV infections have been described in some cases with neuropathological symptoms such as seizures, meningoencephalopathy, myelitis, and choroiditis going back to the 1960s [Citation100–106]. Analysis of the La Reunion CHIKV epidemic demonstrated an increase in neurological complications such as Guillain-Barré syndrome [Citation107]. A study conducted between 2015 and 2017 in Pakistan suggested that CHIKV confirmed that patients were just as likely to present neurological complications as “New World” West Nile virus patients [Citation108]. A meta-analysis of CHIKV literature identified 856 cases of neurological disease, with the most common pathology described as encephalopathy [Citation109]. More alarmingly, in March 2023 WHO reported that “the apparent higher proportion of acute meningoencephalitis attributed to Chikungunya in Paraguay is of concern” due to an unusually high incidence of neurovirulence symptoms [Citation110]. Despite this, in vitro studies on CHIKV’s ability to infect brain endothelial cells are still under debate, with human brain microvascular endothelial cells appearing to be susceptible, while primary brain endothelial cells were CHIKV-resistant [Citation111,Citation112].
In the case of ONNV, cases of neurological manifestations have yet to be observed. However, an in vitro study investigating the immunomodulation of the peripheral nervous system of Schwann cells post-ONNV infection demonstrated its capacity to infect, replicate, and induce an inflammatory immune host response [Citation113]. The authors noted the strong similarities with CHIKV-associated Guillain-Barré syndrome model previously described [Citation107]. They further hypothesized that the infection and inflammation was enabled by the expression of the alphavirus receptor Mxra8 on Schwann cells. Additionally, ONNV was found to be infectious in the brain and skeletal muscle of A129 (IFNα/βR KO) mice [Citation114]. Collectively, like for CHIKV, the possibility of neuropathogenesis complications in ONNV infections cannot be underestimated.
No ONNV infections have been reported to be fatal as of 2023. However, should it spread, the potential for this tropical virus to present a substantial socioeconomic burden is evident. This highlights the importance of implementing prospective longitudinal studies to identify, track and study ONNV infected individuals in endemic regions to build a clearer understanding of its clinical manifestations.
Mechanisms of pathogenesis
The typical animal model to study arthritogenic alphavirus mechanisms of pathology are immune competent mice models due to their ability to effectively recapitulate the self-limiting arthritis, tenosynovitis, and myositis seen in human hosts [Citation115]. After initial subcutaneous inoculation, the virus begins on-site replication and is eventually transported to nearby draining lymph nodes. This was observed in Ross River Virus (RRV) infections, indicating that this transportation is likely mediated through infected migrating antigen-presenting immune cells (APCs) such as dendritic cells or monocytes/macrophages, providing a means of dissemination throughout the body [Citation116]. The infection then triggers leukocyte infiltration into the muscles, bones, and articular tissues, causing inflammation which manifests into the classic acute symptoms of arthralgia and muscle pain [Citation117–119]. More generally, it appears that as arthritogenic alphaviruses replicate within the joints and muscles, a positive feedback loop between localised virus-associated cytopathy and immune cell proinflammatory responses further amplifies the pathology [Citation120]. Experiments with CHIKV in mouse and immunocompetent macaques show that the virus establishes long-term reservoirs in joint-associated tissues, lymph nodes, the liver, and spleen – additionally believed to be the primary sites of viral replication and dissemination [Citation121,Citation122]. Various cell types, encompassing those within joints, bones, and muscles, along with immune cells present in the synovium of infected tissues, have been identified as susceptible to infection by arthritogenic alphaviruses. In the case of ONNV, in vitro studies have demonstrated a significantly higher susceptibility in dermal fibroblasts and synoviocytes than endothelial cells and skeletal muscle cells [Citation29]. Further histopathological assessments in vivo in mice have corroborated this, with notable muscle degradation, synovitis, and tenosynovitis surrounding the tendon capsule [Citation123].
Host immune responses
It has become increasingly clear that ONNV pathology, much like other “Old World” alphaviruses, is greatly attributed to host-driven immunopathology [Citation124]. Within each case of viral infection, it is the unique host-pathogen interplay that dictates the outcomes of disease. In the aim of developing effective and targeted therapeutics towards these arboviruses, understanding these mechanisms and interactions remains essential.
Pattern recognition
At a cellular level, the innate immune response operates on the primary activation of host pattern recognition receptors (PRRs) by pathogen-associated molecular patterns (PAMPs) to trigger downstream antimicrobial responses, including the induction of the type-I interferon response and pro-inflammatory cytokine expression [Citation125]. At the frontline of this are the membrane-bound Toll-Like Receptors (TLRs). Alphavirus genomes, being single-strand RNA viruses, are recognised by endosomal TLR7 and TLR8 [Citation126,Citation127], during the events of viral entry and/or uptake of cellular debris. This was corroborated with RRV infection studies in TLR7/MyD88 deficient mice [Citation128]. Furthermore, the dsRNA sensor TLR3 has been linked to arthritogenic alphavirus pathogenicity, although conflicting reports exist regarding its impact on CHIKV pathogenesis. One study found that mice deficient in TLR3 exhibited heightened viraemia, increased virus dissemination, infiltration of pro-inflammatory myeloid cells, and an inability to effectively clear the virus when exposed to CHIKV challenge [Citation129]. Another in vivo study, however, found TLR3 irrelevant to controlling viraemia [Citation119]. Single nucleotide polymorphisms of all three TLRs (TLR7, TLR8, TLR3) were found associated with differential severities of CHIKV disease in infected patients [Citation119,Citation130]. Specifically concerning ONNV, exploration of such genome-wide associations remains uncharted territory. Given conflicting results even within a single virus, there is a pressing need for further investigation into the significance of these PRRs in mediating both antiviral and immunopathogenic responses to alphavirus infections on a virus-specific basis. However, it is noteworthy that TLR-homologous Toll pathway in Anopheles haemocytes was shown to play a dual role in restricting ONNV infection and being actively downregulated during infection [Citation131].
Within the cytosol, PAMP recognition is mediated by a variety of intracellular molecular sensors capable of inducing type-I interferon and pro-inflammatory cytokine expression. Retinoic acid-inducible gene-I (RIG-I/DDX58) like receptors and melanoma differentiation-associated protein 5 (MDA-5/IFIH1) are members of a larger family of DExH/D-box RNA helicases that act as molecular sensors for virally encoded dsRNA intermediates and 5′ triphosphates [Citation132]. In vitro analysis on human fibroblast cells found RIG-I is significantly upregulated post-ONNV infection, suggesting a role for the cytosolic receptors in the innate immune response against ONNV [Citation133]. The cyclic GMP – AMP synthase stimulator of interferon genes (cGAS-STING) pathway constitutes another pivotal component of foreign DNA and cellular damage detection in the cytosol, orchestrating a robust antiviral response, but has also been implicated in various autoimmune and inflammatory disorders [Citation134]. In vivo modelling proposes that cGAS expression is essential for mediating the innate antiviral response against various alphaviruses, including: SINV, ONNV, Venezuelan equine encephalitis virus (VEEV), and CHIKV; through a unique activation of an IRF3-driven antiviral program, independent from canonical IFN/STAT1 signalling [Citation135]. These innate immune detection pathways are in turn intricately regulated by a variety of regulatory proteins, with one notable example being the ubiquitin X domain-containing gene 3B (UBXN3B). UBXN3B appears to play a crucial role as a positive regulator of the cGAS-STING pathway, mediating effective antiviral immune responses against both DNA and RNA viruses [Citation136]. Indeed, UBXN3B deficiency renders mice models highly susceptible to the severe immunopathologic arthritis induced by both arthritogenic alphaviruses, CHIKV, and ONNV [Citation137]. NOD-, LRR-, and pyrin domain-containing protein 3 (NLRP3) is another important cytosolic sensor that, when activated, leads to the assembly of the NLRP3 inflammasome, resulting in cellular pyroptosis [Citation138]. Inhibition of NRLP3 and downstream effector caspase-1 was shown to alleviate inflammatory symptoms for both CHIKV and RRV in vivo [Citation139]. Post-ONNV infection, NLRP3, and caspase-1 expression have been observed to increase in vitro [Citation133].
Innate immune response
The Type-I IFN response is constituted by the release of IFN-α and IFN-β cytokines following the initial detection of PAMPs and subsequent signalling. Integral to innate immunity against viral pathogens, these cytokines play a key role in controlling viral infections by inducing antiviral states in both virus-infected and uninfected cells [Citation140]. Type-I IFNs are also capable of causing downstream immunopathogenic and immunosuppressive consequences during both acute and chronic viral infections [Citation140,Citation141]. These dual characteristics underscore their importance in the immunopathology associated with arthritogenic alphaviruses, such as ONNV. Indeed, response to ONNV infection appears to be highly reliant on type-I IFN protection within the early acute phase of infection [Citation114]. Mice lacking IFNα/β signalling was most susceptible to lethal ONNV infection with infectious virions found in the brain and skeletal muscle [Citation114]. Importantly, MAVS-dependant type I IFN signalling in monocytes and in particular inflammatory monocytes have been demonstrated to be important mediators for controlling CHIKV and RRV infection [Citation142]. Interestingly, those infiltrating monocytes/macrophages have contrasting roles on viral clearance and tissue damage and repair (reviewed in [Citation143]). In contrast to type I IFN, and IFNγ signalling (type-II IFN) deficiency appeared to have no effect on mice survival during ONNV infection [Citation114]. This cytokine, however, stands out as highly elevated during the acute phase of alphavirus infection, particularly within the context of CHIKV [Citation144,Citation145].
PRR and IFN signalling activates the JAK/STAT pathway to induce the expression of antiviral effector molecules encoded by IFN-stimulated genes (ISGs), many of which have been identified to be associated with alphavirus infection and pathogenicity [Citation146]. An example being interferon-induced transmembrane 3 (IFITM3), a small transmembrane ISG shown to restrict pH-dependent viral fusion of multiple arthritogenic viruses (CHIKV, ONNV, SFV, SINV) and the encephalitic alphavirus VEEV [Citation147]. This was further supported when the ectopic expression of IFITM3 in STAT1-deficient human fibroblasts appeared to moderately reduce ONNV and CHIKV infection success [Citation135,Citation148]. Similarly, an increase in viral replication was observed in mouse fibroblasts lacking IFITM3 [Citation147]. Mice lacking in IFITM3 also exhibited more pronounced joint swelling, elevated proinflammatory cytokine levels, and increased viral burden, revealing a direct link between this ISG to the infection and pathogenicity of multiple arthritogenic alphaviruses (CHIKV, ONNV, SFV, and SINV) [Citation147]. Another important ISG is Viperin (RSAD2), which was shown to be important during immune responses to CHIKV in mouse models [Citation149]. Interestingly, this particular ISG was shown to possess a direct antiviral effect as well as influences innate and adaptive immune response to CHIKV infections [Citation69,Citation150] as well as other pathogens such as mycobacterium tuberculosis and West Nile virus (WNV) [Citation151,Citation152].
Adaptive immune response
Although there is a consensus that the innate immune response is responsible for the early control of alphavirus infection, mouse studies have illuminated the significant involvement of the adaptive immune response during the late acute and chronic phases of infection, for ONNV and CHIKV clearance and immunopathology [Citation114]. Viral clearance for alphaviruses in late acute phase is mostly mediated via antibody mechanisms. B-cells are essential for viral clearance as seen in CHIKV infections [Citation121,Citation153–155], producing neutralizing antibodies, mostly targeting the envelope proteins (reviewed in [Citation52,Citation63]). Interestingly, some of those highly neutralising antibodies against alphaviruses when assessed as potential therapies have been shown to necessitate Fc receptor functions for protective activity, as seen with MAYV (Earnest [Citation50]).
In ONNV infected mice, during the footpad swelling peak at day 6 post-subcutaneous infection, joints are infiltrated with inflammatory monocytes, CD4+ and CD8+ T-cell populations [Citation29,Citation123]. Depletion or inhibition of CD4+ T-cell infiltration was able to significantly alleviate the joint inflammation peak occurring at day 6, reducing total immune cell infiltration, muscle degeneration, and necrosis with no effect on viral clearance, confirming the key role of CD4+ T-cells in driving immunopathogenesis. For CHIKV, the pathogenic role of adaptive immunity is well understood with Th1 polarized CD4+ T-cells as a key driver of both acute and chronic arthritic joint pathology [Citation182,Citation69,Citation129]. On the other hand, CD8+ T-cells, while typically considered good antiviral effectors, have been shown to be unable to control viral load nor contribute to immunopathogenesis in mouse models [Citation153,Citation183]. This was further corroborated by a study showing that despite induction of virus-specific competent effector CD8+ T-cells, CHIKV was observed to be able to subvert these responses and allow for viral persistence in joint associated tissues [Citation156,Citation184].
Therapeutics
Vaccines
At the time of this review, there are currently no commercially available vaccines for ONNV. However, the FDA has recently approved a live attenuated CHIKV vaccine by Valneva [Citation157,Citation158]. It was attenuated by the deletion of part of the nsP3 protein [Citation159] and showed the generation of seroprotective antibody levels in 98.9% of the immunized participants [Citation160,Citation161]. Interestingly, as mentioned previously, nsP3 contains the hypervariable domain which interacts with a high number of host factors essential for full alphaviral virulence. Thus, abolishing some of these key interactions can lead to other live attenuated vaccine candidates, such as abolishing interaction motifs with FHL1 [Citation162]. Other strategies have generated promising live attenuated vaccine candidates, such as restricting replication in skeletal muscle cells [Citation163], the deletion of part of the capsid protein [Citation164] or point mutations in the non-structural proteins, triggering high early IFN responses and lower infectivity [Citation165]. In addition to live attenuated vaccines, several other platforms and technologies are currently in development (reviewed in [Citation166]). Interestingly, in several of these studies, vaccinated animals were challenged with related alphaviruses including ONNV and showed good cross-protection, suggesting that a CHIKV vaccine could be used against ONNV in the event of an outbreak.
Antibody based therapies
Treatment options for arthritogenic alphavirus infections likewise remain limited. The medical consensus currently is to manage symptoms using general anti-inflammatory medications like meloxicam and pain relief drugs such as paracetamol [Citation167]. For prophylaxis or for therapy, monoclonal antibodies (mAb) have been extensively explored for alphaviruses (reviewed in [Citation63]) and are mostly focused on the structural proteins E2 and E1. Interestingly, some mAbs have been shown to possess high cross-protection as exemplified by CHK-265, a mouse anti-CHIKV antibody able to neutralize CHIKV, MAYV. RRV, SFV and ONNV in mouse models [Citation168,Citation169]. mAbs have been also investigated in non-human primates and showed protective effects [Citation170,Citation171]. In addition to passive transfer of antibodies, the possibility of inoculating mRNA coding for the protective antibodies was explored and showed positive results with no serious adverse effects [Citation172], suggesting that mRNA-Lipid NanoParticle (mRNA-LNP) injections could be an avenue for prophylaxis or treatment during an outbreak or travel to an endemic area. One complementary approach to virus-targeting mAbs, is the clever use of anti-alphavirus receptor Mxra8 (conserved amongst the Semliki forest serogroup) blocking antibody or Mxra8 decoys, which showed efficacy in mice models for both CHIKV and ONNV [Citation60] Zhang [Citation61].
Antivirals
To our knowledge, no specific antivirals have been developed for ONNV. Nevertheless, almost all steps of other alphaviruses life cycle have been targeted for potential antiviral developments, and this has been extensively reviewed recently [Citation173,Citation174]. It is important to highlight that these antivirals can target either the viral or the host side of the virus-host interplay and this will lead to important strengths and weaknesses in the identified compounds. Viral-specific compounds will target viral proteins and can interfere, for example, with entry, enzymatic activity, protein–protein, or protein–RNA interactions. The advantage of these compounds is that they are usually virus or viral family specific and therefore have low toxicity to the host cells. However, due to the nature of positive sense RNA viruses [Citation175], the apparition of escape mutants is likely to appear rapidly.
For compounds targeting proviral host factors, there will be a higher probability of a broader range antiviral effect with lower escape mutation potential, but the trade-off is often a higher toxicity. For example, targeting valosin containing protein, also called p97, was shown to be highly antiviral for different viruses such as hepatitis C virus [Citation176], Japanese encephalitis virus [Citation177] and arthritogenic alphaviruses such as ONNV, CHIKV, and SFV [Citation68,Citation178]. However, despite IC50 well below CC50 for some compounds during the assay timeframe, unsurprising, longer treatment leads to toxicity since it is an essential gene and that these compounds were initially developed for chemotherapy (reviewed in [Citation179]). For efficient antiviral strategies targeting proviral host factors, there will be the need to develop non-toxic compounds that target the interplay between the virus and the host factor while preserving the host factor functionality or target non-essential genes that viruses are highly dependent on. For the latter, compounds targeting FHL1, a major host factor for some alphaviruses such as CHIKV and ONNV [Citation180], could be an interesting avenue for drug development. Similarly, a group has started to explore potential binders of G3BP1 inhibiting CHIKV infection with no effect on G3BP1 cellular functions (preprint [Citation181]).
Concluding remarks
The explosive re-emergence of arthritogenic alphaviruses such as CHIKV and MAYV has highlighted the health concerns and associated morbidity that come with these viruses. Research efforts on alphaviruses have produced a very detailed understanding on current epidemic viruses which are of importance in developed countries such as CHIKV, VEEV, RRV and to a lesser extent MAYV. While ONNV has so far been limited to the African continent, there is no known scientific argument that it will not spread to other continents, especially in the current context of rapid climate change leading to a shift and expansion of mosquito vector ecological niches. Fortunately, currently available pre-clinical studies in animal models strongly suggest that vaccines efficient against CHIKV should cross-protect against ONNV. Their availability would allow prompt immunisation of populations if local transmission events were detected outside of Africa to quickly control epidemics similar to ring vaccination strategies developed for Ebola. As detailed in this review, most of our knowledge of ONNV virus is implied from related alphaviruses. Therefore, a lot of important research questions are still open such as, why are ONNV and CHIKV so closely related genetically but differ in competent vector transmission. What are the extent of ONNV clinical manifestations and the incidence of neurological complications? Why do they differ in some key clinical manifestations such as absence of oedema or presence of lymphadenopathies, and what are the consequences on their mechanisms of immunopathogenicity during acute and chronic phases?
Author contributions
Samuel Tong Jia Ming, Guillaume Carissimo: conception and design. Samuel Tong Jia Ming, Katrina Tan Yi Jun, Guillaume Carissimo: literature review, data synthesis and drafting of the manuscript. Guillaume Carissimo: critical revision for intellectual content.
Acknowledgements
We want to thank the Electron Microscopy Unit, National University of Singapore for image acquisition and analysis and in particular Benoit Malleret and Erica Qian Hui Lee.
Disclosure statement
No potential conflict of interest was reported by the author(s).
Data availability statement
Data sharing is not applicable to this article as no new data were created or analysed in this study.
Correction Statement
This article has been republished with minor changes. These changes do not impact the academic content of the article.
Additional information
Funding
References
- Kading RC, Brault AC, Beckham JD. Global perspectives on arbovirus outbreaks: a 2020 snapshot. TropicalMed. 2020;5(3):142. doi: 10.3390/tropicalmed5030142
- Girard M, Nelson CB, Picot V. Arboviruses: a global public health threat. Vaccine. 2020;38(24):3989–17.
- Koerich LB, Sant’anna MRV, Huits R. Recent technological advances and strategies for arbovirus vector control. Trop Med Infect Dis. 2022;7(9):204.
- Grandadam M, Caro V, Plumet S. Chikungunya virus, southeastern France. Emerg Infect Dis. 2011;17(5):910–913.
- Rezza G, Nicoletti L, Angelini R. Infection with chikungunya virus in Italy: an outbreak in a temperate region. Lancet. 2007;370(9602):1840–1846.
- Balakrishnan VS. WHO launches global initiative for arboviral diseases. Lancet Microbe. 2022;3(6):e407.
- WHO. 2022. Global distribution of Chikungunya virus. from https://www.who.int/health-topics/chikungunya
- Liu-Helmersson J, Brannstrom A, Sewe MO. Estimating past, present, and future trends in the global distribution and abundance of the arbovirus vector Aedes aegypti under climate change scenarios. Front Public Health. 2019;7:148.
- Gorris ME, Bartlow AW, Temple SD. Updated distribution maps of predominant culex mosquitoes across the Americas. Parasites Vectors. 2021;14(1):547.
- Carlson CJ, Bannon E, Mendenhall E. Rapid range shifts in African anopheles mosquitoes over the last century. Biol Lett. 2023;19(2):20220365.
- Powers AM, Brault AC, Shirako Y. Evolutionary relationships and systematics of the alphaviruses. J Virol. 2001;75(21):10118–10131.
- Levi LI, Vignuzzi M. Arthritogenic alphaviruses: a worldwide emerging threat? Microorganisms. 2019;7(5):133.
- Strauss JH, Strauss EG. The alphaviruses: gene expression, replication, and evolution. Microbiol Rev. 1994;58(3):491–562.
- Haddow AJ, Davies C, Walker A. O’nyong-nyong fever: an epidemic virus disease in East Africa. I. Introduction. Trans R Soc Trop Med. 1960;54(6):517–522. doi:10.1016/0035-9203(60)90025-0
- Cottis S, Blisnick AA, Failloux AB. Determinants of Chikungunya and O’nyong-Nyong virus specificity for infection of Aedes and Anopheles mosquito Vectors. Viruses. 2023;15(3):589.
- Bowen ET, Simpson DI, Platt GS. Large scale irrigation and arbovirus epidemiology, Kano Plain, Kenya. II. Preliminary serological survey. Trans R Soc Trop Med Hyg. 1973;67(5):702–709.
- Woodruff AW, Bowen ET, Platt GS. Viral infections in travellers from tropical Africa. Br Med J. 1978;1(6118):956–958.
- Lhuillier M, Cunin P, Mazzariol M. Epidémie rurale à virus «Igbo Ora»(avec transmission inter-humaine) en Côte-d’Ivoire en 1984-1985. Bulletin de la Société de pathologie exotique. 1988;81(3):386–395.
- Sanders EJ, Rwaguma EB, Kawamata J. O’nyong-nyong fever in south-central Uganda, 1996–1997: description of the epidemic and results of a household-based seroprevalence survey. J Infect Dis. 1999;180(5):1436–1443.
- Posey DL, O’Rourke T, Roehrig JT. O’Nyong-nyong fever in West Africa. Am J Trop Med Hyg. 2005;73(1):32.
- Bessaud M, Peyrefitte CN, Pastorino BA. O’nyong-nyong virus, chad. Emerg Infect Dis. 2006;12(8):1248–1250.
- LaBeaud AD, Banda T, Brichard J. High rates of O’nyong nyong and Chikungunya virus transmission in coastal Kenya. PLOS Negl Trop Dis. 2015;9(2):e0003436.
- Hoze N, Diarra I, Sangare AK. Model-based assessment of Chikungunya and O’nyong-nyong virus circulation in Mali in a serological cross-reactivity context. Nat Commun. 2021;12(1):6735.
- Masika MM, Korhonen EM, Smura T. Serological evidence of exposure to o'nyong-nyong and chikungunya viruses in febrile patients of rural Taita-Taveta county and Urban Kibera informal settlement in Nairobi, Kenya. Viruses. 2022;14(6):1286.
- Pezzi L, LaBeaud A, Reusken C. GloPID-R report on chikungunya, O’nyong-nyong and Mayaro virus, part 2: epidemiological distribution of o’nyong-nyong virus. Antiviral Res. 2019;172:104611.
- Clements TL, Rossi CA, Irish AK, et al. Chikungunya and O’nyong-nyong viruses in Uganda: implications for diagnostics. 2019 Jan 3;6(3):ofz001. doi:10.1093/ofid/ofz001. PMID: 31660384; PMCID: PMC6411207.
- Williams M, Woodall J, Corbet PS. O’nyong-nyong fever: an epidemic virus disease in East Africa. VIII. virus isolations from anopheles mosquitoes. Trans R Soc Trop Med. 1965;59(3):300–306.
- Guerra CA, Gikandi PW, Tatem AJ, et al. The limits and intensity of Plasmodium falciparum transmission: implications for malaria control and elimination worldwide. PLOS Med. 2008;5(2):e38.
- Torres-Ruesta A, Teo TH, Chan YH. Malaria abrogates O’nyong-nyong virus pathologies by restricting virus infection in nonimmune cells. Life Sci Alliance. 2022;5(4):e202101272.
- Mala W, Wilairatana P, Kotepui KU. Prevalence of malaria and chikungunya co-Infection in febrile patients: a systematic review and meta-analysis. Trop Med Infect Dis. 2021;6(3):119.
- Teo TH, Lum FM, Ghaffar K. Plasmodium co-infection protects against chikungunya virus-induced pathologies. Nat Commun. 2018;9(1):3905.
- De Zulueta J, Woodall JP, Cullen JR. An observation on the possible effect of O’Nyong-nyong fever on malaria. Bull World Health Organ. 1962;26(1):135–139.
- Tappe D, Kapaun A, Emmerich P. O’nyong-nyong virus infection imported to Europe from Kenya by a traveler. Emerg Infect Dis. 2014;20(10):1766–1767.
- Tsetsarkin KA, Vanlandingham DL, McGee CE, et al. A single mutation in chikungunya virus affects vector specificity and epidemic potential. PLOS Pathog. 2007;3(12):e201.
- Afrane YA, Githeko AK, Yan G. The ecology of anopheles mosquitoes under climate change: case studies from the effects of deforestation in East African highlands. Ann NY Acad Sci. 2012;1249(1):204–210.
- Mutsaers M, Engdahl CS, Wilkman L. Vector competence of Anopheles stephensi for O’nyong-nyong virus: a risk for global virus spread. Parasites Vectors. 2023;16(1):133.
- Lanciotti RS, Ludwig ML, Rwaguma EB. Emergence of epidemic O’nyong-nyong fever in Uganda after a 35-year absence: genetic characterization of the virus. Virology. 1998;252(1):258–268.
- Diagne CT, Bengue M, Choumet V. Mayaro virus pathogenesis and transmission mechanisms. Pathogens. 2020;9(9):738.
- Diallo D, Fall G, Diagne CT. Concurrent amplification of Zika, Chikungunya, and Yellow fever virus in a sylvatic focus of arboviruses in Southeastern Senegal, 2015. BMC Microbiol. 2020;20(1):181.
- Matusali G, Colavita F, Bordi L. Tropism of the chikungunya virus. Viruses. 2019;11(2):175.
- Brault AC, Foy BD, Myles KM. Infection patterns of O’nyong nyong virus in the malaria-transmitting mosquito, anopheles gambiae. Insect Mol Biol. 2004;13(6):625–635.
- Saxton-Shaw KD, Ledermann JP, Borland EM. O’nyong nyong virus molecular determinants of unique vector specificity reside in non-structural protein 3. PLOS Negl Trop Dis. 2013;7(1):e1931.
- Brustolin M, Pujhari S, Henderson CA. Anopheles mosquitoes may drive invasion and transmission of Mayaro virus across geographically diverse regions. PLOS Negl Trop Dis. 2018;12(11):e0006895.
- Vanlandingham DL, Hong C, Klingler K. Differential infectivities of o’nyong-nyong and chikungunya virus isolates in anopheles gambiae and Aedes aegypti mosquitoes. Am J Trop Med Hyg. 2005;72(5):616–621.
- Vanlandingham DL, Tsetsarkin K, Klingler KA. Determinants of vector specificity of O’nyong nyong and Chikungunya viruses in anopheles and Aedes mosquitoes. Am J Trop Med Hyg. 2006;74(4):663–669.
- Götte B, Liu L, McInerney GM. The enigmatic alphavirus non-structural protein 3 (nsP3) revealing its secrets at last. Viruses. 2018;10(3):105.
- Levinson RS, Strauss JH, Strauss EG. Complete sequence of the genomic RNA of O’nyong-nyong virus and its use in the construction of alphavirus phylogenetic trees. Virology. 1990;175(1):110–123.
- Powers AM, Brault AC, Tesh RB. Re-emergence of chikungunya and O’nyong-nyong viruses: evidence for distinct geographical lineages and distant evolutionary relationships. J Gen Virol. 2000;81(Pt 2):471–479.
- Ashbrook AW, Burrack KS, Silva LA. Residue 82 of the Chikungunya virus E2 attachment protein modulates viral dissemination and arthritis in mice. J Virol. 2014;88(21):12180–12192.
- Earnest, J. T., Basore, K., Roy, V., Bailey, A. L., Wang, D., Alter, G., … Diamond, M. S. Neutralizing antibodies against Mayaro virus require Fc effector functions for protective activity. J Exp Med 2019;216:2282-2301, doi:10.1084/jem.20190736
- Weger-Lucarelli J, Aliota MT, Wlodarchak N, et al. Dissecting the role of E2 protein domains in alphavirus pathogenicity. J Virol. 2016;90(5):2418–2433.
- Holmes AC, Basore K, Fremont DH. A molecular understanding of alphavirus entry. PLOS Pathog. 2020;16(10):e1008876.
- Gardner CL, Ebel GD, Ryman KD. Heparan sulfate binding by natural eastern equine encephalitis viruses promotes neurovirulence. Proc Natl Acad Sci, USA. 2011;108(38):16026–16031. doi: 10.1073/pnas.1110617108
- Silva LA, Khomandiak S, Ashbrook AW. A single-amino-acid polymorphism in Chikungunya virus E2 glycoprotein influences glycosaminoglycan utilization. J Virol. 2014;88(5):2385–2397.
- Froelich S, Tai A, Kennedy K. Virus-receptor mediated transduction of dendritic cells by lentiviruses enveloped with glycoproteins derived from Semliki Forest virus. PLOS One. 2011;6(6):e21491.
- Klimstra WB, Nangle EM, Smith MS. DC-SIGN and L-SIGN can act as attachment receptors for alphaviruses and distinguish between mosquito cell-and mammalian cell-derived viruses. J Virol. 2003;77(22):12022–12032.
- Lozach PY, Burleigh L, Staropoli I. The C type lectins DC-SIGN and L-SIGN: receptors for viral glycoproteins. Methods Mol Biol. 2007;379:51–68.
- Jemielity S, Wang JJ, Chan YK. TIM-family proteins promote infection of multiple enveloped viruses through virion-associated phosphatidylserine. PLOS Pathogens. 2013;9(3):e1003232.
- Moller-Tank S, Kondratowicz AS, Davey RA. Role of the phosphatidylserine receptor TIM-1 in enveloped-virus entry. J Virol. 2013;87(15):8327–8341.
- Zhang R, Kim AS, Fox JM. Mxra8 is a receptor for multiple arthritogenic alphaviruses. Nature. 2018;557(7706):570–574.
- Earnest JT, Basore K, Roy V. Neutralizing antibodies against Mayaro virus require Fc effector functions for protective activity. J Exp Med. 2019;216(10):2282–2301.
- Clark LE, Clark SA, Lin C. VLDLR and ApoER2 are receptors for multiple alphaviruses. Nature. 2022;602(7897):475–480.
- Kim AS, Diamond MS. A molecular understanding of alphavirus entry and antibody protection. Nat Rev Microbiol. 2023;21(6):396–407.
- Ma H, Kim AS, Kafai NM. LDLRAD3 is a receptor for Venezuelan equine encephalitis virus. Nature. 2020;588(7837):308–314.
- Zhai X, Li X, Veit M, et al. LDLR is used as a cell entry receptor by multiple alphaviruses. Nat Commun. 2024;15(1):622.
- De Caluwé L, Coppens S, Vereecken K, et al. The CD147 protein complex is involved in entry of chikungunya virus and related alphaviruses in human cells. Front Microbiol. 2021;12:615165.
- Wintachai P, Thuaud F, Basmadjian C. Assessment of flavaglines as potential Chikungunya virus entry inhibitors. Microbiol Immunol. 2015;59(3):129–141.
- Carissimo G, Chan YH, Utt A. VCP/p97 Is a proviral host factor for replication of Chikungunya virus and other alphaviruses. Front Microbiol. 2019;10:2236.
- Carissimo G, Teo TH, Chan YH. Viperin controls chikungunya virus-specific pathogenic T cell IFNgamma Th1 stimulation in mice. Life Sci Alliance. 2019;2(1):e201900298. doi:10.26508/lsa.201900298
- Bernard E, Solignat M, Gay B. Endocytosis of Chikungunya virus into mammalian cells: role of clathrin and early endosomal compartments. PLoS One. 2010;5(7):e11479.
- Scott CC, Vacca F, Gruenberg J. Endosome maturation, transport and functions. Semin Cell Dev Biol. 2014 Jul;31:2–10. doi:10.1016/j.semcdb.2014.03.034. PMID: 24709024.
- Varghese FS, Rausalu K, Hakanen M. Obatoclax inhibits alphavirus membrane fusion by neutralizing the acidic environment of endocytic compartments. Antimicrob Agents Chemother. 2017;61(3):02227–02216.10.1128/aac.
- Wahlberg JM, Garoff H. Membrane fusion process of semliki forest virus. I: Low pH-induced rearrangement in spike protein quaternary structure precedes virus penetration into cells. J Cell Bio. 1992;116(2):339–348.
- Helenius A, Kartenbeck J, Simons K. On the entry of semliki forest virus into BHK-21 cells. J Cell Bio. 1980;84(2):404–420.
- Paredes AM, Ferreira D, Horton M. Conformational changes in Sindbis virions resulting from exposure to low pH and interactions with cells suggest that cell penetration may occur at the cell surface in the absence of membrane fusion. Virology. 2004;324(2):373–386.
- Li L, Jose J, Xiang Y. Structural changes of envelope proteins during alphavirus fusion. Nature. 2010;468(7324):705–708.
- Zaitseva E, Mittal A, Griffin DE. Class II fusion protein of alphaviruses drives membrane fusion through the same pathway as class I proteins. J Cell Bio. 2005;169(1):167–177.
- de Groot RJ, Hardy WR, Shirako Y. Cleavage-site preferences of Sindbis virus polyproteins containing the non-structural proteinase. Evidence for temporal regulation of polyprotein processing in vivo. Embo J. 1990;9(8):2631–2638.
- Kaariainen L, Ahola T. Functions of alphavirus nonstructural proteins in RNA replication. Prog Nucleic Acid Res Mol Biol. 2002;71:187–222.
- Myles KM, Kelly CL, Ledermann JP. Effects of an opal termination codon preceding the nsP4 gene sequence in the O’Nyong-nyong virus genome on Anopheles gambiae infectivity. J Virol. 2006;80(10):4992–4997.
- Tomar S, Hardy RW, Smith JL, et al. Catalytic core of alphavirus nonstructural protein nsP4 possesses terminal adenylyltransferase activity. J Virol. 2006;80(20):9962–9969.
- Paul D, Bartenschlager R. Architecture and biogenesis of plus-strand RNA virus replication factories. World J Virol. 2013;2(2):32–48.
- Firth AE, Chung BY, Fleeton MN. Discovery of frameshifting in alphavirus 6K resolves a 20-year enigma. Virol J. 2008;5:108.
- Peränen J, Laakkonen P, Hyvönen M. The alphavirus replicase protein nsP1 is membrane-associated and has affinity to endocytic organelles. Virology. 1995;208(2):610–620.
- Russo AT, Malmstrom RD, White MA, et al. Structural basis for substrate specificity of alphavirus nsP2 proteases. J Mol Graph Model. 2010;29(1):46–53.
- Law Y-S, Utt A, Tan YB. Structural insights into RNA recognition by the chikungunya virus nsP2 helicase. Proc Nat Acad Sci. 2019;116(19):9558–9567.
- Saisawang C, Sillapee P, Sinsirimongkol K. Full length and protease domain activity of chikungunya virus nsP2 differ from other alphavirus nsP2 proteases in recognition of small peptide substrates. Biosci Rep. 2015 Apr 22;35(3):e00196. doi:10.1042/BSR20150086. PMID: 26182358; PMCID: PMC4445351.
- Byers NM, Burns PL, Stuchlik O. Identification of mosquito proteins that differentially interact with alphavirus nonstructural protein 3, a determinant of vector specificity. PloS Negl Trop Dis. 2023;17(1):e0011028.
- Mazzon M, Castro C, Thaa B. Alphavirus-induced hyperactivation of PI3K/AKT directs pro-viral metabolic changes. PLOS Pathog. 2018;14(1):e1006835.
- Neuvonen M, Kazlauskas A, Martikainen M, et al. SH3 domain-mediated recruitment of host cell amphiphysins by alphavirus nsP3 promotes viral RNA replication. PLOS Pathog. 2011;7(11):e1002383.
- Rubach JK, Wasik BR, Rupp JC. Characterization of purified sindbis virus nsP4 RNA-dependent RNA polymerase activity in vitro. Virology. 2009;384(1):201–208.
- Staples JE, Powers AM. Togaviridae: Alphaviruses. In: Long, SS, editor. Principles and Practice of Pediatric Infectious Diseases. 6th ed. Elsevier; 2023. p. 1145–1147.e3. doi:10.1016/B978-0-323-75608-2.00217-2
- Kiwanuka N, Sanders EJ, Rwaguma EB O’nyong-nyong fever in south-central Uganda, 1996-1997: clinical features and validation of a clinical case definition for surveillance purposes. Clin Infect Dis. 1999;29(5):1243–1250.
- Soares-Schanoski A, Baptista Cruz N, de Castro-Jorge LA. Systems analysis of subjects acutely infected with the chikungunya virus. PLOS Pathog. 2019;15(6):e1007880.
- Pinheiro FP, Freitas RB, Travassos da Rosa JF. An outbreak of Mayaro virus disease in Belterra, Brazil. I. Clinical and virological findings. Am J Trop Med Hyg. 1981;30(3):674–681.
- Assuncao-Miranda I, Cruz-Oliveira C, Da Poian AT. Molecular mechanisms involved in the pathogenesis of alphavirus-induced arthritis. Biomed Res Int. 2013;2013:973516.
- Marques CDL, Ranzolin A, Cavalcanti NG, et al. Arboviruses related with chronic musculoskeletal symptoms. Best Pract Res Clin Rheumatol. 2020;34(4):101502.
- Wesula Olivia L, Obanda V, Bucht G, et al. Global emergence of alphaviruses that cause arthritis in humans. Infect Ecol Epidemiol. 2015;5(1):29853.
- Schilte C, Staikowsky F, Couderc T. Chikungunya virus-associated long-term arthralgia: a 36-month prospective longitudinal study. PLOS Negl Trop Dis. 2013;7(3):e2137.
- Arpino C, Curatolo P, Rezza G. Chikungunya and the nervous system: what we do and do not know. Rev Med Virol. 2009;19(3):121–129.
- Carey DE, Myers RM, DeRanitz C. The 1964 chikungunya epidemic at Vellore, South India, including observations on concurrent dengue. Trans R Soc Trop Med. 1969;63(4):434–445.
- Chastel C. Human infections in Cambodia by the Chikungunya virus or an apparently closely related agent. Ii. Experimental pathological anatomy. Bull Soc Pathol Exot Filiales. 1963;56(5):915–924.
- Das T, Jaffar-Bandjee MC, Hoarau JJ. Chikungunya fever: CNS infection and pathologies of a re-emerging arbovirus. Prog Neurobiol. 2010;91(2):121–129.
- Hammon WM, Rudnick A, Sather GE. Viruses associated with epidemic hemorrhagic fevers of the Philippines and Thailand. Science. 1960;131(3407):1102–1103.
- Nimmannitya S, Halstead SB, Cohen SN. Dengue and chikungunya virus infection in man in Thailand, 1962-64. I. Observations on hospitalized patients with hemorrhagic fever. Am J Trop Med Hyg. 1969;18(6, Pt. 1):954–971.
- Thiruvengadam KV, Kalyanasundaram V, Rajgopal J. Clinical and pathological studies on Chikungunya fever in Madras city. Indian J Med Res. 1965;53(8):729–744.
- Oehler E, Fournier E, Leparc-Goffart I. Increase in cases of Guillain-Barre syndrome during a Chikungunya outbreak, French Polynesia, 2014 to 2015. Euro Surveill. 2015;20(48):30079.
- Khan E, Barr KL, Farooqi JQ. Human West Nile virus disease outbreak in Pakistan, 2015–2016. Front Public Health. 2018;6:20.
- Mehta R, Gerardin P, de Brito CAA, et al. The neurological complications of Chikungunya virus: A systematic review. Rev Med Virol. 2018;28(3):e1978.
- WorldHealth Organization. Geographical expansion of cases of dengue and Chikungunya beyond the historical areas of transmission in the region of the Americas. Disease Outbreak News. 2023. https://www.who.int/emergencies/disease-outbreak-news/item/2023-DON448
- Couderc T, Chrétien F, Schilte C, et al. Cell and tissue tropisms of Chikungunya virus and its dissemination to the central nervous system. BMC Proc. 2008;2(Suppl 1):S7. doi:10.1186/1753-6561-2-s1-s7
- Sourisseau M, Schilte C, Casartelli N. Characterization of reemerging Chikungunya virus. PLOS Pathog. 2007;3(6):e89.
- Bedoui Y, De Larichaudy D, Daniel M. Deciphering the role of Schwann cells in inflammatory peripheral neuropathies post alphavirus infection. Cells. 2022;12(1):100.
- Seymour RL, Rossi SL, Bergren NA, et al. The role of innate versus adaptive immune responses in a mouse model of O’nyong-nyong virus infection. Am J Trop Med Hyg. 2013;88(6):1170–1179.
- Gardner J, Anraku I, Le TT, et al. Chikungunya virus arthritis in adult wild-type mice. J Virol. 2010;84(16):8021–8032.
- Tharmarajah K, Everest-Dass A, Vider J. N-Linked glycans shape skin immune responses during arthritis and myositis after intradermal infection with ross river virus. J Virol. 2022;96(17):e0099922.
- Chen W, Foo S-S, Sims NA. Arthritogenic alphaviruses: new insights into arthritis and bone pathology. Trends Microbiol. 2015;23(1):35–43.
- Chow A, Her Z, Ong EK, et al. Persistent arthralgia induced by Chikungunya virus infection is associated with interleukin-6 and granulocyte macrophage colony-stimulating factor. J Infect Dis. 2011;203(2):149–157.
- Schilte C, Couderc T, Chretien F, et al. Type I IFN controls Chikungunya virus via its action on nonhematopoietic cells. J Exp Med. 2010;207(2):429–442.
- Hoarau JJ, Jaffar Bandjee MC, Krejbich Trotot P, et al. Persistent chronic inflammation and infection by Chikungunya arthritogenic alphavirus in spite of a robust host immune response. J Immunol. 2010;184(10):5914–5927.
- Hawman DW, Stoermer KA, Montgomery SA, et al. Chronic joint disease caused by persistent Chikungunya virus infection is controlled by the adaptive immune response. J Virol. 2013;87(24):13878–13888.
- Labadie K, Larcher T, Joubert C, et al. Chikungunya disease in nonhuman primates involves long-term viral persistence in macrophages. J Clin Invest. 2010;120(3):894–906.
- Chan YH, Teo TH, Torres-Ruesta A. Longitudinal [18F]FB-IL-2 PET imaging to assess the immunopathogenicity of O’nyong-nyong virus Infection. Front Immunol. 2020;11:894.
- Traverse EM, Millsapps EM, Underwood EC. Chikungunya immunopathology as it presents in different organ systems. Viruses. 2022;14(8):1786.
- Brubaker SW, Bonham KS, Zanoni I, et al. Innate immune pattern recognition: a cell biological perspective. Annu Rev Immunol. 2015;33:257–290.
- Diebold SS, Kaisho T, Hemmi H, et al. Innate antiviral responses by means of TLR7-mediated recognition of single-stranded RNA. Science. 2004;303(5663):1529–1531.
- Heil F, Hemmi H, Hochrein H, et al. Species-specific recognition of single-stranded RNA via toll-like receptor 7 and 8. Science. 2004;303(5663):1526–1529.
- Neighbours LM, Long K, Whitmore AC. Myd88-dependent toll-like receptor 7 signaling mediates protection from severe ross river virus-induced disease in mice. J Virol. 2012;86(19):10675–10685.
- Her Z, Teng TS, Tan JJ, et al. Loss of TLR3 aggravates CHIKV replication and pathology due to an altered virus-specific neutralizing antibody response. EMBO Mol Med. 2015;7(1):24–41.
- Dutta SK, Tripathi A. Association of toll-like receptor polymorphisms with susceptibility to Chikungunya virus infection. Virology. 2017;511:207–213.
- Carissimo G, Pondeville E, McFarlane M, et al. “Antiviral immunity of anopheles gambiae is highly compartmentalized, with distinct roles for RNA interference and gut microbiota. Proc Nat Acad Sci. 2015;112(2):E176–E185. doi: 10.1073/pnas.1412984112
- Brisse M, Ly H. Comparative structure and function analysis of the RIG-I-Like receptors: RIG-I and MDA5. Front Immunol. 2019;10:1586.
- Sandenon Seteyen AL, Guiraud P, Gasque P, et al. In vitro analyses of the multifocal effects of natural alkaloids berberine, matrine, and tabersonine against the O’nyong-nyong arthritogenic alphavirus infection and inflammation. Pharmaceuticals (Basel). 2023;16(8):1125.
- Liu Y, Pu F. Updated roles of cGAS-STING signaling in autoimmune diseases. Front Immunol. 2023;14:1254915.
- Schoggins JW, MacDuff DA, Imanaka N, et al. Pan-viral specificity of IFN-induced genes reveals new roles for cGAS in innate immunity. Nature. 2014;505(7485):691–695.
- Yang L, Wang L, Ketkar H, et al. UBXN3B positively regulates STING-mediated antiviral immune responses. Nat Commun. 2018;9(1):2329.
- Geng T, Yang D, Lin T, et al. UBXN3B controls immunopathogenesis of arthritogenic alphaviruses by maintaining hematopoietic homeostasis. MBio. 2022;13(6):e0268722.
- Swanson KV, Deng M, Ting JP. The NLRP3 inflammasome: molecular activation and regulation to therapeutics. Nat Rev Immunol. 2019;19(8):477–489.
- Chen W, Foo SS, Zaid A, et al. Specific inhibition of NLRP3 in chikungunya disease reveals a role for inflammasomes in alphavirus-induced inflammation. Nat Microbiol. 2017;2(10):1435–1445.
- McNab F, Mayer-Barber K, Sher A, et al. Type I interferons in infectious disease. Nat Rev Immunol. 2015;15(2):87–103.
- Biron CA. Interferons α and β as immune regulators—a new look. Immunity. 2001;14(6):661–664.
- Haist KC, Burrack KS, Davenport BJ, et al. Inflammatory monocytes mediate control of acute alphavirus infection in mice. PLOS Pathog. 2017;13(12):e1006748.
- Atella MO, Carvalho AS, Da Poian AT. Role of macrophages in the onset, maintenance, or control of arthritis caused by alphaviruses. Exp Biol Med (Maywood). 2023 Nov;248(22):2039–2044. doi:10.1177/15353702231214261. PMID: 38058027; PMCID: PMC10800133.
- Kelvin AA, Banner D, Silvi G, et al. Inflammatory cytokine expression is associated with Chikungunya virus resolution and symptom severity. PLOS negl trop dis. 2011;5(8):e1279.
- Wauquier N, Becquart P, Nkoghe D, et al. The acute phase of Chikungunya virus infection in humans is associated with strong innate immunity and T CD8 cell activation. J Infect Dis. 2011;204(1):115–123.
- Schoggins JW. Interferon-stimulated genes: what do they all do? Annu Rev Virol. 2019;6:567–584.
- Poddar S, Hyde JL, Gorman MJ, et al. The interferon-stimulated gene IFITM3 restricts infection and pathogenesis of arthritogenic and encephalitic alphaviruses. J Virol. 2016;90(19):8780–8794.
- Schoggins JW, Wilson SJ, Panis M, et al. A diverse range of gene products are effectors of the type I interferon antiviral response. Nature. 2011;472(7344):481–485.
- Teng TS, Foo SS, Simamarta D, et al. Viperin restricts chikungunya virus replication and pathology. J Clin Invest. 2012;122(12):4447–4460.
- Hee JS, Cresswell P. Viperin interaction with mitochondrial antiviral signaling protein (MAVS) limits viperin-mediated inhibition of the interferon response in macrophages. PLOS One. 2017;12(2):e0172236.
- Szretter KJ, Brien JD, Thackray LB, et al. The interferon-inducible gene viperin restricts West Nile virus pathogenesis. J Virol. 2011;85(22):11557–11566.
- Zhou X, Zhang Z, Xu H, et al. Viperin impairs the innate immune response through the IRAK1-TRAF6-TAK1 axis to promote Mtb infection. Sci Signaling. 2022;15(754):eabe1621.
- Lum FM, Teo TH, Lee WW, et al. An essential role of antibodies in the control of chikungunya virus infection. J Immunol. 2013;190(12):6295–6302.
- Poo YS, Rudd PA, Gardner J, et al. Multiple immune factors are involved in controlling acute and chronic chikungunya virus infection. PLOS Negl Trop Dis. 2014;8(12):e3354.
- Seymour RL, Adams AP, Leal G, et al. A rodent model of Chikungunya virus infection in RAG1 -/- mice, with features of persistence, for vaccine safety evaluation. PlOS Negl Trop Dis. 2015;9(6):e0003800.
- Davenport BJ, Bullock C, McCarthy MK, et al. Chikungunya Virus evades antiviral CD8(+) T cell responses to establish persistent infection in Joint-associated tissues. J Virol. 2020;94(9):e02036–02019.
- Harris E. FDA approves first chikungunya vaccine. JAMA. 2023. Dec 19;330(23):2241. doi:10.1001/jama.2023.23505. PMID: 38019496.
- Roques P, Fritzer A, Dereuddre-Bosquet N, et al. Effectiveness of CHIKV vaccine VLA1553 demonstrated by passive transfer of human sera. JCI Insight. 2022 Jul 22;7(14):e160173. doi:10.1172/jci.insight.160173. PMID: 35700051; PMCID: PMC9431671.
- Hallengard D, Kakoulidou M, Lulla A, et al. Novel attenuated Chikungunya vaccine candidates elicit protective immunity in C57BL/6 mice. J Virol. 2014;88(5):2858–2866.
- Coates EE, Edupuganti S, Chen GL, et al. Safety and immunogenicity of a trivalent virus-like particle vaccine against western, eastern, and Venezuelan equine encephalitis viruses: a phase 1, open-label, dose-escalation, randomised clinical trial. Lancet Infect Dis. 2022;22(8):1210–1220.
- Schneider M, Narciso-Abraham M, Hadl S, et al. Safety and immunogenicity of a single-shot live-attenuated chikungunya vaccine: a double-blind, multicentre, randomised, placebo-controlled, phase 3 trial. Lancet. 2023;401(10394):2138–2147.
- Ng WH, Liu X, Ling ZL, et al. FHL1 promotes chikungunya and O’nyong-nyong virus infection and pathogenesis with implications for alphavirus vaccine design. Nat Commun. 2023;14(1):6605.
- Lentscher AJ, McCarthy MK, May NA, et al. Chikungunya virus replication in skeletal muscle cells is required for disease development. J Clin Investig. 2020;130(3):1466–1478.
- Taylor A, Liu X, Zaid A, et al. Mutation of the N-terminal region of chikungunya virus capsid protein: implications for vaccine design. MBio. 2017;8(1):01970–0191610.1128/mbio.
- Chan YH, Teo TH, Utt A, et al. Mutating Chikungunya virus non-structural protein produces potent live-attenuated vaccine candidate. EMBO Mol Med. 2019;11(6):e10092.
- DeFilippis VR. Chikungunya virus vaccines: platforms, progress, and challenges. In: Heise M, editors. Chikungunya Virus. Cham: Springer International Publishing, p 81–106:2022.
- Marti-Carvajal A, Ramon-Pardo P, Javelle E, et al. Interventions for treating patients with Chikungunya virus infection-related rheumatic and musculoskeletal disorders: A systematic review. PLoS One. 2017;12(6):e0179028.
- Fox JM, Huang L, Tahan S, et al. A cross-reactive antibody protects against ross river virus musculoskeletal disease despite rapid neutralization escape in mice. PLOS Pathog. 2020;16(8):e1008743.
- Fox JM, Long F, Edeling MA, et al. Broadly neutralizing alphavirus antibodies bind an epitope on E2 and inhibit entry and egress. Cell. 2015;163(5):1095–1107.
- Broeckel R, Fox JM, Haese N, et al. Therapeutic administration of a recombinant human monoclonal antibody reduces the severity of Chikungunya virus disease in rhesus macaques. PLOS Negl Trop Dis. 2017;11(6):e0005637.
- Burke CW, Froude JW, Rossi F, et al. Therapeutic monoclonal antibody treatment protects nonhuman primates from severe Venezuelan equine encephalitis virus disease after aerosol exposure. PLOS Pathog. 2019;15(12):e1008157.
- Kose N, Fox JM, Sapparapu G, et al. A lipid-encapsulated mRNA encoding a potently neutralizing human monoclonal antibody protects against chikungunya infection. Sci Immunol. 2019;4(35):eaaw6647.
- Abdelnabi R, Delang L. Antiviral strategies against arthritogenic alphaviruses. Microorganisms. 2020;8(9):1365.
- Skidmore AM, Bradfute SB. The life cycle of the alphaviruses: from an antiviral perspective. Antiviral Res. 2023;209:105476.
- Patterson EI, Khanipov K, Swetnam DM, et al. Measuring alphavirus fidelity using non-infectious virus particles. Viruses. 2020 May 15;12(5):546. doi:10.3390/v12050546. PMID: 32429270; PMCID: PMC7291308.
- Yi Z, Fang C, Zou J, et al. Affinity purification of the hepatitis C virus replicase identifies valosin-containing protein, a member of the ATPases associated with diverse cellular activities family, as an active virus replication modulator. J Virol. 2016;90(21):9953–9966.
- Sehrawat S, Khasa R, Deb A, et al. Valosin-containing protein/p97 plays critical roles in the Japanese encephalitis virus life cycle. J Virol. 2021;95(11):02336–0232010.1128/jvi.
- Yin P, Jian X, Liu Y, et al. Elucidating cellular interactome of Chikungunya virus identifies host dependency factors. Virol Sin. 2023;38(4):497–507.
- Kilgas S, Ramadan K. Inhibitors of the ATPase p97/VCP: From basic research to clinical applications. Cell Chem Biol. 2023;30(1):3–21.
- Meertens L, Hafirassou ML, Couderc T. FHL1 is a major host factor for Chikungunya virus infection. Nature. 2019;574(7777):259–263.
- Mahajan S, Kumar R, Singh A. Targeting host protein G3BP1 for the discovery of novel antiviral inhibitors against the Chikungunya virus. bioRxiv. 2023. 2022.2011.2011.516135.
- Teo T-H, Her Z, Tan JJ. Caribbean and La reunion Chikungunya virus isolates differ in their capacity to induce proinflammatory Th1 and NK cell responses and acute joint pathology. J Virol. 2015;89(15):7955–7969.
- Teo TH, Lum FM, Claser C. A pathogenic role for CD4+ T cells during Chikungunya virus infection in mice. J Immunol. 2013;190(1):259–269.
- Zhang R, Earnest JT, Kim AS. Expression of the Mxra8 receptor promotes alphavirus infection and pathogenesis in mice and drosophila. Cell Rep. 2019;28(10): 2647–2658. e2645.