ABSTRACT
To investigate the functions of hypoxia inducible factor-1α (HIF-1α) homologs, we cloned and characterized the full-length cDNA of a novel HIF-1α homolog (SinHIF-1α) from the sea urchin Strongylocentrotus intermedius. We then explored the functions of SinHIF-1α and its regulatory factor (miR-2013-3p) in the pathogen-induced metabolic response of S. intermedius. The results showed the following: 1) The full-length cDNA of SinHIF-1α was 4265 bp, with a high level of sequence conservation across the phylum Echinodermata. 2) MiR-2013-3p could bind to the 3’-UTR of SinHIF-1α and negatively regulate the expression of SinHIF-1α in S. intermedius. 3) Both SinHIF-1α silencing and miR-2013-3p overexpression suppressed the relative content of adenosine triphosphate (ATP) in coelomocytes as well as the relative expression (mRNA and protein) and total enzyme activity of glutamate dehydrogenase (GDH) in S. intermedius. 4) The miR-2013-3p/SinHIF-1α axis may regulate ATP production at 6–24 h and 48–72 h post-pathogen infection through accompanied by alterations in the relative expression and enzyme activity of GDH in S. intermedius. In summary, all observations from this study indicated that the pathogen utilizes the miR-2013-3p/SinHIF-1α axis to reduce ATP production and thereby attenuate the antimicrobial capability of the host via targeting GDH.
Introduction
Hypoxia inducible factor-1α (HIF-1α) is an oxygen-sensitive transcription factor belonging to the HIF family. HIF-1α is proteasomally degraded via interaction with the von Hippel – Lindau (VHL) protein under standard oxygen levels (normoxia) [Citation1]. Under hypoxic conditions, HIF-1α is stabilized and accumulated; this enhances the dimerization of HIF-1α and HIF-β and leads to the transcription activation of genes associated with anaerobic metabolism, erythrocyte production, mitochondrial metabolism, and angiogenesis [Citation2]. In aquatic animals, HIF-1α homologs have been identified and characterized in fishes [Citation3–8], arthropods [Citation9,Citation10], and molluscs [Citation11–14]. Hypoxia inducing the upregulation of HIF-1α has also been observed in Perca fluviatilis [Citation15], Clarias batrachus [Citation16], Pelteobagrus vachelli [Citation17], and Micropterus salmoides [Citation18]. However, it remains unclear whether physiological changes involving HIF-1α are induced by other types of exogenous stimuli (such as pathogen infection).
Oxygen is essential for cellular energy production in aerobic organisms [Citation19]. Given the close association between oxygen and the stability and activity of HIF-1α, alterations in the energy budget have always been a focus in functional studies of HIF-1α. It has been documented that HIF-1α decreases the level of cellular adenosine triphosphate (ATP) under hypoxic conditions by altering glucose metabolism, from glucose oxidation to glycolysis, via targeting important glycolytic genes such as glucose transporter (GLUT) [Citation20–23], hexokinases (HK) [Citation24], 6-phosphofructo-2-kinase/fructose-2,6-biphosphatase (PFKFB) [Citation18], and phosphofructokinase (PFKP) [Citation17]. However, besides the glycolysis pathway, whether HIF-1α regulates other energy metabolic pathways has yet to be fully investigated. In addition, as a hub regulator for many cellular physiological processes, factors affecting the stability and activity of HIF-1α have been considered in HIF-1α functional research. For example, prolyl hydroxylase (PHD) and factor-inhibiting hypoxia-inducible factor (FIH) are effectors regulating the proteasomal degradation of HIF-1α [Citation1,Citation25,Citation26]. Furthermore, growth hormone (GH) can affect the stability of HIF-1α through activation of insulin-like growth factor (IGF) signalling pathways [Citation27]. However, little is unknown concerning the effects of epigenetic regulators such as microRNAs (miRNAs) or long non-coding RNAs (lncRNAs) on the stability and activity of HIF-1α.
Sea urchins, as representative marine invertebrates and fishery resources [Citation28], have been employed for more than a century as model animals to elucidate the origin and mechanisms of innate immunity [Citation29–33]. Recently, our bioinformatics prediction data suggested that there may be a potential regulatory targeting relationship between HIF-1α and miR-2013-3p in the sea urchin Strongylocentrotus intermedius. Furthermore, inspired by the observation that silencing of HIF-1α decreased the mortality of shrimp infected with white spot syndrome virus (WSSV) [Citation34] and that miR-2013 has been demonstrated to be an epigenetic regulator involved in the innate immune response of amphioxus (Branchiostoma belcheri tsingtaunese) [Citation35], we therefore hypothesized that 1) HIF-1α may be involved in the cellular response to pathogen infection in other aquatic invertebrates (sea urchins in this study); 2) the regulatory function of HIF-1α induced by pathogen infection could be affected by miR-2013-3p, and 3) energy metabolism may be regulated by the interaction between HIF-1α and miR-2013-3p during pathogen infection because the expression of HIF-1α could affect the level of ATP [Citation17,Citation18,Citation20–24].
To investigate the above hypotheses, the temperate sea urchin S. intermedius, a representative aquatic invertebrate of economic importance, was used as an experimental model in the current study. Sequencing of the HIF-1α of S. intermedius (named SinHIF-1α) was performed, and the gene was characterized. The targeting relationship between SinHIF-1α and miR-2013-3p was subsequently investigated and validated. Alterations in the relative expression of both SinHIF-1α and miR-2013-3p in S. intermedius and their functions in ATP production in coelomocytes after infection with the causative pathogen of sea urchin black peristomial membrane disease were investigated.
Materials and methods
Experimental animals and cell lines
Healthy specimens of S. intermedius (average wet body weight 20.0 ± 2.0 g; average test diameter 34.1 ± 3.3 mm) were collected from the Dalian Lvshun Longwangtang Farm (Dalian, Liaoning Province, China). Prior to experimentation, all specimens were maintained in ~1000 L laboratory circulating seawater tanks without feeding for 3 days. Temporary incubation conditions included freshly filtered seawater (FSW) maintained at 15.0°C, with a salinity of 31.21 ± 0.20, a pH of 8.10 ± 0.03, a dissolved oxygen concentration of 7.88 mg/L, and a water change cycle of 2 days.
Human embryonic kidney cells transformed with SV40 large T-antigen (HEK-293T) were used as the vectors for the expression of luciferase enzyme, as they have the advantages of a rapid growth rate, robustness, and stronger bioluminescent signals [Citation36,Citation37]. In recent years, HEK-293T cells have been widely used in luciferase reporter assays in aquatic animals to verify the binding sites between miRNAs and their target genes [Citation38,Citation39]. In this study, HEK-293T cells were purchased from Sangon Biotechnology (Shanghai, China), maintained in DMEM (Sangon Biotechnology, Shanghai, China) supplemented with 10% foetal bovine serum (FBS), and cultured at 37°C in a 5% CO2 incubator.
Sample collection
Nine healthy adult S. intermedius were starved for 3 days. Tissues (tube foot, perioral membrane, interdental muscle, coelomocytes, gonad, and intestine) were then carefully removed. For the collection of coelomocytes, coelomic fluid was centrifuged at 3000 rpm for 10 min at 4°C immediately after collection. All samples were immediately frozen in liquid nitrogen and stored at − 80°C for cDNA isolation and expression analysis.
Total RNA extraction and cDNA synthesis
Total RNA extraction, detection of RNA integrity and concentration, synthesis of the first cDNA strand, and full-length amplification were performed according to Ren et al. [Citation40]. The obtained cDNA was diluted and stored at − 20°C. A SMARTer® RACE 5’/3’ Kit (Sangon Biotechnology, China) was used to synthesize a 5’/3’ terminal RACE cDNA template via qualified total RNA synthesis. The reaction system and PCR procedure were performed according to the manufacturer’s instructions. The obtained 5’/3’ terminal RACE cDNA template was diluted and stored at − 20°C. PCR products were recovered and purified using a SanPrep Column DNA Gel Extraction Kit (Sangon Biotechnology, China). The linking, transformation, and colony PCR experiments were performed according to Ren et al. [Citation40]. After colony PCR identification, three positive clones were selected and sent to Sangon Biotechnology (Shanghai, China) for sequencing.
Characterization of SinHIF-1α
Characterization analyses of SinHIF-1α were performed following the methods of Liu et al. [Citation41], including complete full-length cDNA sequence assembling and annotation, sequence structure analyses, physicochemical property predictions, multiple sequence alignment, and phylogenetic analysis. The information used for multiple sequence alignment, characteristic domain comparison, and phylogenetic analysis is shown in Table S1.
Quantitative real-time reverse transcription-polymerase chain reaction (qRT-PCR) and western blotting
The relative expression of SinHIF-1α, miR-2013-3p, and SinGDH was analysed using a LightCycler® 96 real-time quantitative PCR instrument (Roche Life Science, Germany). The primers used for qRT-PCR are listed in . β-actin and U6 (RNAU6B) were used as internal references because they are commonly employed in relative transcriptional expression analyses of aquatic animals [Citation41–45]. The relative expression levels of SinHIF-1α, miR-2013-3p, and SinGDH were analysed by the 2−ΔΔCt method [Citation46].
Table 1. PCR primers used in this study.
The recombinant expression of SinHIF-1α and SinGDH proteins and the preparation of a polyclonal antibody were completed at Dia-An Biotechnology (Wuhan, China). To test the specificity of the SinHIF-1α and SinGDH antibodies, the antiserum was preabsorbed with the purified recombinant SinHIF-1α and SinGDH proteins at 4°C for 16 h. The pre-immune serum from the same rabbit was subjected to western blotting analysis as a negative control. The total protein concentration determination of each tissue sample and the steps involved in western blotting were performed following the methods of Zhao et al. [Citation47] and Li et al. [Citation48].
Target prediction of miRNA and 3’-UTR luciferase reporter assay
The target fragment of miR-2013-3p in SinHIF-1α was identified through RNA22 v2 microRNA target detection (https://cm.jefferson.edu/rna22/Interactive/) and artificial sequence alignment (Sangon Biotechnology; Shanghai, China). Wild-type SinHIF-1α containing a putative target of miR-2013-3p at the 3’-noncoding region (UTR) and a 3’-UTR mutant SinHIF-1α were synthesized by Sangon Biotechnology (Shanghai, China) (). The target fragment was inserted between the XhoI and NotI sites of the luciferase plasmid to construct the plasmid, and the clones were further confirmed by sequencing. In the transfection experiment, HEK-293T cells were inoculated in 96-well white TC plates with a total volume of 100 μL, and two solutions were prepared: the first consisted of 10 µL of DMEM, 0.16 µg of pmirGLO luciferase plasmid or 200 ng of wild-type or mutant-type SinHIF-1α 3’-UTR, and 5 pmol of the miRNA/negative control (NC). The second solution consisted of 10 μL of DMEM and 0.3 μL of Lipofectamine 2000 (Lip2000; Thermo Fisher Scientific, USA), and it was incubated at room temperature for 5 min. Then, 25 μL of the first and second solutions was combined and incubated at room temperature for 20 min. Then, 100 μL of the medium was added to each well of a 96-well white TC plate inoculated with HEK-293T cells. After transfection (48 h), the cells were collected, and the activity was determined using a dual luciferase reporter assay system (E1980, Promega Biotechnology, Beijing). The luciferase activity was determined by calculating the signal ratio between Renilla Luciferase and Firefly Luciferase using SpectraMax i3× enzyme marker (Mei Ya Molecular Instruments, China). All experiments were performed in three replicates.
Functional analysis of miRNA in vivo
The miRNA agomir, antagomir, and NC were designed and synthesized at Gene-Pharma (Shanghai, China; sequence information is shown in ) and were dissolved in RNase-free water to obtain a working solution of 20 nM. Then, 10 μL of miR-2013-3p agomir (or antagomir) or NC (or NC-inhibitor) was mixed with 10 μL of Lip 2000 transfection reagent and 80 μL of PBS to serve as the transfection solution. Healthy sea urchins were injected with 100 μL of transfection solution or the NC solution mixture. At 24 h post-transfection, the coelomocytes from each group were collected, immediately frozen in liquid nitrogen, and stored at − 80°C for further analyses by qRT-PCR, western blotting, enzyme activity assays, and determination of ATP content. The assays described above were repeated three times and were run in triplicate.
Table 2. Synthetic sequences used in this study.
Silencing of SinHIF-1α using siRNA
Specific small interfering RNAs (siRNAs) targeting SinHIF-1α (siHIF-1α) and NC-RNAi were designed and synthesized by Sangon Biotech (Shanghai, China; ). For the in vivo SinHIF-1α knockdown, 10 μL of siHIF-1α (20 nM) or NC-RNAi was mixed with 10 μL of Lip 2000 transfection reagent and 80 μL of PBS to serve as the transfection solution. Healthy sea urchins were injected with 100 μL of the siHIF-1α mixture or the NC-RNAi mixture (as a control). The injection procedures are summarized in Fig S1. No significant changes in status were observed in the examined sea urchins before or after injection. At 24 h post-transfection, the coelomocytes from the siHIF-1α group and the control group were collected, immediately frozen in liquid nitrogen, and stored at − 80°C for further analyses by qRT-PCR, western blotting, enzyme activity assay, and determination of ATP content. The assays described above were repeated three times and were run in triplicate.
Laboratory infection and sample collection
To investigate the expression of SinHIF-1α in response to bacterial infection, a causative pathogen strain of sea urchin black peristomial membrane disease (strain No.: SIBMPM03) was chosen to infect S. intermedius [Citation49]. A total of 40 healthy adult S. intermedius were selected and randomly divided into two groups (20 individuals each). All specimens in the challenged group were immersed in seawater containing the pathogen (1 × 107 CFU·mL−1), and the specimens of the other group were without any treatment and served as controls. Three individuals from each group were randomly selected at 0, 6, 12, 24, 48, and 72 h post-infection (hpi) (n = 3). The coelomocytes of each individual from each group were collected, immediately frozen in liquid nitrogen, and stored at − 80°C for further analyses by qRT-PCR, western blotting, enzyme activity assay, and determination of ATP content.
Enzyme activity and total ATP content determination
Coelomocytes (0.5 g) were ground in liquid nitrogen, and sterile water was added in proportion to the weight of the coelomocytes, with the ratio of coelomocytes (g) to sterile water volume (ml) being 1:9. The protein concentration of the homogenate, SinGDH enzyme activity, and the ATP content were determined using commercial kits (No. A045–2, No. A125-1-1, and No. A095-1-1, respectively; Nanjing Jiancheng Bioengineering Institute, China) following the manufacturer’s instructions. The absorbance of the chromogenic solution was determined using an Epoch enzyme labelling apparatus (Biotek, USA). The protein concentration of the homogenate, SinGDH enzyme activity, and the ATP content were calculated by using the following formulae:
Cp (g/L) = [(Tt - T0)/(Ts – T0)] × 0.524 × DR,
SinGDH enzyme activity (U/mgprot) = [643 × (AT1– AT0)]/Cp,
ATP content (μmol/gprot) = [(Tt - Tc)/(Ts – T0)] × 1000 ×DR/Cp,
where Cp is the protein concentration; Tt is the transmittance of the test group; Tc is the transmittance of the control group; Ts is the transmittance of the standard group; T0 is the transmittance of the blank group; DR is the dilution ratio of coelomocytes; AT0 is the initial light absorption value of the reaction; and AT1 is the light absorption value after the reaction.
Data analysis
All data were expressed as mean ± S.D. Prism 9 (GraphPad, USA) was used for data processing, data collation, and chart drawing. Statistical analysis was performed by independent-sample t-tests and one-way analysis of variance (ANOVA) in Prism 9. Significant differences and extremely significant differences were set at P < 0.05 and P < 0.01, respectively.
Results
SinHIF-1α cDNA sequence characterization
The results of RACE amplification showed that the full-length cDNA of SinHIF-1α (GenBank accession No. OQ397583) was 4265 bp, comprising a 245 bp 5’-UTR, a 2799 bp ORF, and a 1218 bp 3’-UTR (). The ORF of SinHIF-1α encoded a protein of 933 aa with a predicted molecular weight of 103.53 kDa. Further analyses indicated that the SinHIF-1α protein was a non-transmembrane hydrophilic protein (). The structure prediction results revealed that the secondary structure of the SinHIF-1α protein contained 29 α-helices, 16 β-strands, and 14 random coils (). Characteristic domain analysis revealed that the SinHIF-1α protein contained one 54 aa basic helix-loop-helix (bHLH) domain (residues 14–67) and two Per-ARNT-Sim (PAS) domains (PAS-A: 69 aa, residues 83–151; PAS-B: 52 aa, residues 252–303). The HIF-1α protein from Mus musculus (Swiss-model No.: 4zpr.1.B) was used as the template for 3D structure prediction of the SinHIF-1α protein and the HIF-1α proteins from six other species. The similarities of the SinHIF-1α protein and the HIF-1α proteins from six other species were 83.43% (Xenopus tropicalis), 77.78% (Danio rerio), 47.17% (Scylla paramamosain), 53.29% (Mizuhopecten yessoensis), 53.08% (Apostichopus japonicus), 53.96% (Strongylocentrotus purpuratus), and 54.25% (S. intermedius) compared with the template (). This indicated that the 3D structures of the HIF-1α proteins were relatively conserved from invertebrates to higher vertebrates and that they may have similar functions.
Figure 1. Nucleotide and predicted amino acid sequences of SinHIF-1α in Strongylocentrotus intermedius. The numbers on the left alternate from top to bottom as the nucleotide sequence and the deduced amino acid sequence numbers. The black font indicates the nucleotide sequence, and the red font indicates the amino acid sequence. The basic helix-loop-helix (bHLH) domain (residues 14–67) is shaded in green, the Per-ARNT-Sim (PAS) a (PAS-A) domain (residues 83–151) is shaded in blue, and the PAS-B domain (residues 252–303) is shaded in yellow. Blue italic font represents the initiation codon (ATG), the asterisk (*) represents the termination codon (TGA), and the polyadenylation signal (AATAA) is framed by a box.
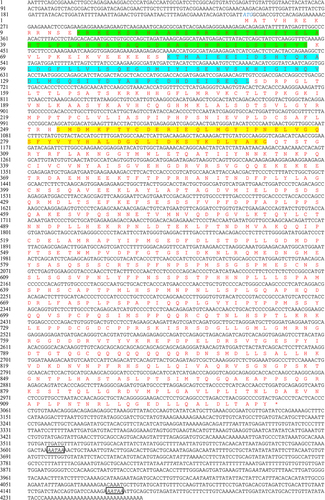
Figure 2. Sequence characteristic analysis of SinHIF-1α in Strongylocentrotus intermedius. (a) Transmembrane structure analysis of SinHIF-1α protein. (b) Hydrophobic or hydrophilic analysis of SinHIF-1α protein. (c) Predicted protein secondary structure (Pred) of the SinHIF-1α protein. Yellow, pink, and grey indicate strands, helices, and coils, respectively. (d) Predicted 3D structure of the SinHIF-1α protein and HIF-1α proteins from six other species. The predicted 3D structure of Mus musculus HIF-1α protein (MmHIF-1α; Swiss-model No.: 4zpr.1.B) was used as a template. XtHIF-1α, DrHIF-1α, SpaHIF-1α, MyHIF-1α, AjHIF-1α, and SpuHIF-1α represent HIF-1α proteins from Xenopus tropicalis, Danio rerio, Scylla paramamosain, Mizuhopecten yessoensis, Apostichopus japonicus, and Strongylocentrotus purpuratus, respectively.
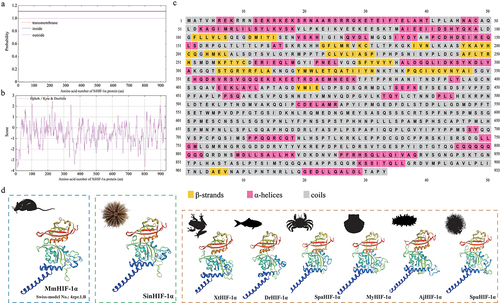
Multiple sequence alignment indicated that the average similarity between the SinHIF-1α sequence and HIF-1α sequences from 28 other species was 40.79% (). The highest similarity (98.18%) was observed between SinHIF-1α and the HIF-1α homolog from S. purpuratus. Characteristic domain comparisons revealed that the SinHIF-1α and HIF-1α proteins of 28 other eukaryotic organisms shared relatively high homologies in the bHLH domain (81.29%) and the PAS domains (PAS-A, 64.32%; PAS-B, 66.85%). In addition, the number and type of characteristic domains of SinHIF-1α were the same as those of HIF-1α proteins from S. purpuratus and Caenorhabditis elegans, but less than those of shrimp HIF-1α protein and fish HIF-1α proteins (). Phylogenetic analysis showed that the SinHIF-1α protein and the HIF-1α protein from S. purpuratus were clustered into one branch and belonged to the echinoderm clade, a result that was consistent with traditional taxonomy ().
Figure 3. Phylogenetic analysis of SinHIF-1α in Strongylocentrotus intermedius. (a) Multiple sequence alignment of HIF-1α proteins from S. intermedius and 28 other species. The bHLH domain is framed by a green box, while the PAS-A and PAS-B domains are framed by a red box and a blue box, respectively. (b) Schematic diagram of the conserved structural domain of HIF-1α homologs from S. intermedius and eight other species. (c) Neighbor-joining phylogenetic tree of HIF-1α family homologs from S. intermedius and 39 other species. SinHIF-1α is marked with a “◄” symbol. The numbers at the tree nodes indicate bootstrap support values based on 1,000 replicates..
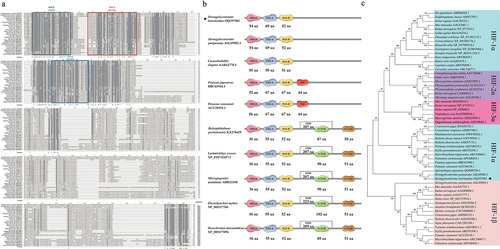
Spatial expression patterns of SinHIF-1α (mRNA and protein) and miR-2013-3p
The results of qRT-PCR showed that both SinHIF-1α mRNA and miR-2013-3p were expressed in all examined tissues. The relative expression of SinHIF-1α mRNA in interdental muscle was the highest in all examined tissues. By contrast, miR-2013-3p was highly expressed in the peristomial membrane, followed by that in the interdental muscle (). Opposite expression trends of SinHIF-1α mRNA and miR-2013-3p were observed in all examined tissues; in particular, the relative expression of miR-2013-3p is higher than that of SinHIF-1α in the coelomocytes and the peristomial membrane. Western blotting showed that the SinHIF-1α protein was expressed in all examined tissues, with the relative expression level from low to high being peristomial membrane < coelomocytes < gonad < interdental muscle. This is similar to the expression pattern of SinHIF-1α mRNA ().
Figure 4. Spatial expression pattern, binding site identification, and verification of miR-2013-3p and SinHIF-1α and their effects on ATP content in coelomocytes of Strongylocentrotus intermedius. (a) Spatial expression pattern of miR-2013-3p and SinHIF-1α mRNA. (b) Spatial expression pattern of SinHIF-1α protein. PM: perioral membrane; IM: interdental muscle; CL: coelomocytes; GN: gonad. Different lower-case letters (e.g. a or a’) represent differing significance levels between different tissues (P< 0.05). ** represents an extremely significant difference between miR-2013-3p and SinHIF-1α mRNA in the same tissue (P < 0.01). (c) Schematic representation of the putative miR-2013-3p targeting site in SinHIF-1α mRNA and the analysis of relative luciferase activities. (d) &; (e) Validation of the interaction relationship of miR-2013-3p and SinHIF-1α in vivo. (f) Effects of miR-2013-3p and SinHIF-1α on ATP content in coelomocytes of S. intermedius. NC-RNAi: negative control of SinHIF-1α silencing (RNAi) group: NC: negative control of miR-2013-3p overexpression (agomir) group; NC-inhibitor: negative control of miR-2013-3p inhibition (antagomir) group. ** represents P < 0.01; n = 3.
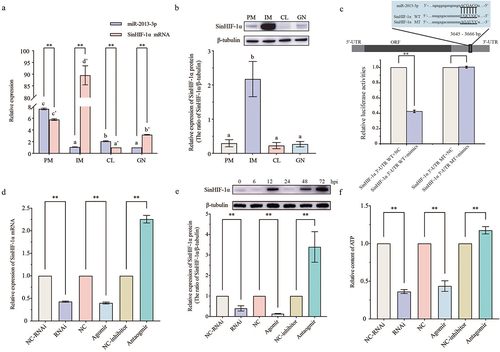
Validation and functional analysis of the interaction between SinHIF-1α and miR-2013-3p
Dual-luciferase reporter assays were employed to validate the binding site of miR-2013-3p at the 3‘-UTR of SinHIF-1α predicted previously. To begin, luciferase reporter vectors containing WT and MT fragments of the SinHIF-1α 3‘-UTR were constructed (). The results showed that the WT 3’-UTR + miR-2013-3p mimic group had a remarkably lower luciferase activity than the NC group (), indicating the existence of a binding site between SinHIF-1α and miR-2013-3p. Further in vivo functional verification experiments revealed that the relative expression levels of SinHIF-1α (mRNA and protein) decreased significantly when miR-2013-3p was overexpressed (P< 0.01), whereas the relative expression of SinHIF-1α (mRNA and protein) was increased significantly when the expression of miR-2013-3p was inhibited (P < 0.01), similar to the results of the RNA interference experiment for SinHIF-1α (). These observations indicated that miR-2013-3p negatively regulated SinHIF-1α protein expression from a posttranscriptional aspect, and that the siRNA of SinHIF-1α inhibited SinHIF-1α expression at the transcriptional level. Meanwhile, the relative ATP content was also reduced significantly under SinHIF-1α silencing or miR-2013-3p overexpression conditions ().
Effects of SinHIF-1α and miR-2013-3p on mRNA expression, enzyme activity of SinGDH, and the ATP content
Significantly decreased relative expression (mRNA and protein) and enzyme activity of SinGDH were observed after SinHIF-1α silencing or miR-2013-3p overexpression (). Combined with the above observation that SinHIF-1α silencing or miR-2013-3p overexpression could reduce the relative ATP content, the functional analysis data for SinHIF-1α and miR-2013-3p indicated that the miR-2013-3p/SinHIF-1α axis may regulate ATP production in coelomocytes of S. intermedius via targeting SinGDH.
Figure 5. Effects of SinHIF-1α and miR-2013-3p on mRNA expression and enzyme activity of SinGDH in coelomocytes of Strongylocentrotus intermedius. (a) and (b) Effects of SinHIF-1α and miR-2013-3p on SinGDH mRNA and protein expression. (c) Effects of SinHIF-1α and miR-2013-3p on total SinGDH enzyme activity. NC-RNAi: negative control of SinHIF-1α silencing (RNAi) group: NC: negative control of miR-2013-3p overexpression (agomir) group; NC-inhibitor: negative control of miR-2013-3p inhibition (antagomir) group. * represents P < 0.05; ** represents P < 0.01; n = 3.
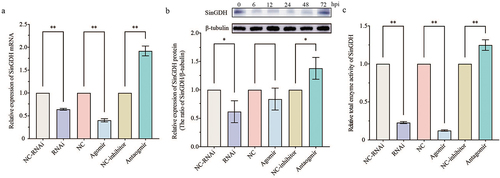
Changes in SinHIF-1α expression (mRNA and protein), miR-2013-3p, and ATP production after pathogen infection
The relative expression of SinHIF-1α mRNA in the coelomocytes of S. intermedius increased initially, then decreased, and then increased again, finally decreasing after pathogen infection. The three expression peaks appeared at 6 hpi, 12 hpi, and 48 hpi (). For the SinHIF-1α protein, the relative expression trend in the coelomocytes of S. intermedius after pathogen infection was similar to the trend in mRNA expression (). The relative expression level of miR-2013-3p in the coelomocytes of S. intermedius after pathogen infection was first downregulated at 6 hpi, then upregulated from 6 hpi to 24 hpi, then decreased at 48 hpi, and then increased from 48 hpi to 72 hpi ().
Figure 6. Pathogen infection altered the relative expression of miR-2013-3p, SinHIF-1α (mRNA and protein), and SinGDH (mRNA and protein) and induced changes in total SinGDH enzyme activity and ATP content in coelomocytes of Strongylocentrotus intermedius. (a) Relative alteration of the expression of miR-2013-3p and SinHIF-1α mRNA in coelomocytes of S. intermedius after pathogen infection. (b) Relative alteration of the expression of SinHIF-1α protein after pathogen infection. (c) Changes in ATP content in coelomocytes of S. intermedius after pathogen infection. (d) &; (e) Relative alteration of the expression of SinGDH mRNA and protein in coelomocytes of S. intermedius after pathogen infection. (f) Total changes in SinGDH enzyme activity in coelomocytes of S. intermedius after pathogen infection. Different lower-case letters (e.g. a) represent differing significance levels between different groups (P < 0.05). ** represents an extremely significant difference compared with 0 hpi (P < 0.01); * represents a significant difference compared with 0 hpi (P < 0.05); n = 3.
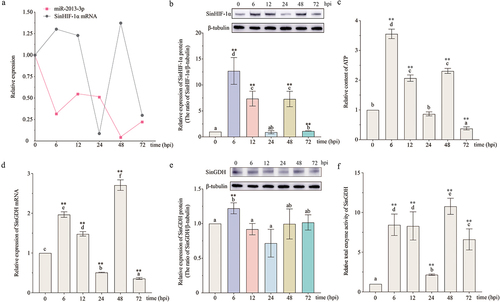
The ATP content analysis results revealed that the relative ATP content in the coelomocytes of S. intermedius increased initially, then decreased, then increased again, and finally decreased after pathogen infection. The three peaks appeared at 6 hpi, 12 hpi, and 48 hpi, consistent with the expression peaks of SinHIF-1α ().
Effects of SinHIF-1α and miR-2013-3p on ATP production via regulating GDH in coelomocytes after pathogen infection
Since the trend in the alteration of ATP content was consistent with the expression trend of SinHIF-1α, we further investigated whether SinHIF-1α and miR-2013-3p could regulate ATP production via targeting GDH in the coelomocytes of S. intermedius after pathogen infection. The relative expression levels of SinGDH (mRNA and protein), total SinGDH enzyme activity, and relative ATP content in the coelomocytes of S. intermedius were then determined under normal conditions, SinHIF-1α silencing, miR-2013-3p overexpression, and miR-2013-3p inhibition after pathogen infection.
The results showed that under pathogen infection conditions, the relative expression of SinGDH mRNA in the coelomocytes of S. intermedius increased initially, then decreased, then increased again, and finally decreased. Three expression peaks appeared at 6 hpi, 12 hpi, and 48 hpi (). For the SinGDH protein, the relative expression trend was similar to the trend of mRNA expression (). Relative total SinGDH enzyme activity in the coelomocytes of S. intermedius after pathogen infection first increased, then increased, and finally decreased; peaks in enzyme activity were observed at 6 hpi, 12 hpi, and 48 hpi ().
In the SinHIF-1α silencing (RNAi) group and the miR-2013-3p overexpression (agomir) group (, the relative ATP content was decreased in the coelomocytes of S. intermedius after pathogen infection (). Meanwhile, the relative expression of SinGDH (mRNA and protein) and the relative total SinGDH enzyme activity exhibited generally decreasing trends in the coelomocytes of S. intermedius after pathogen infection ().
Figure 7. Effects of changes in miR-2013-3p expression and SinHIF-1α silencing on SinHIF-1α and SinGDH (mRNA and protein) expression, ATP content, and total SinGDH enzyme activity in coelomocytes of Strongylocentrotus intermedius after pathogen infection. (a) &; (b) Effects of miR-2013-3p expression changes and SinHIF-1α silencing on SinHIF-1α (mRNA and protein) expression patterns in coelomocytes of S. intermedius after pathogen infection. (c) Effects of miR-2013-3p expression changes and SinHIF-1α silencing on the ATP content in coelomocytes of S. intermedius after pathogen infection. (d) &; (e) Effects of miR-2013-3p expression changes and SinHIF-1α silencing on SinGDH (mRNA and protein) expression patterns in coelomocytes of S. intermedius after pathogen infection. (f) Effects of miR-2013-3p expression changes and SinHIF-1α silencing on total SinGDH enzyme activity after in coelomocytes of S. intermedius after pathogen infection. NC-RNAi: negative control of SinHIF-1α silencing (RNAi) group; NC: negative control of miR-2013-3p overexpression (agomir) group; NC-inhibitor: negative control of miR-2013-3p inhibition (antagomir) group. Different lower-case letters (e.g. a, a’, or A) represent differing significance levels between different groups (P < 0.05). * represents P < 0.05; ** represents P < 0.01; n = 3.
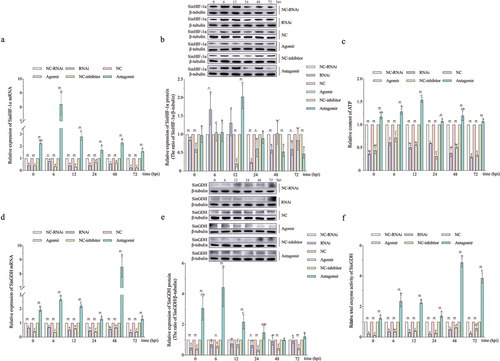
In the miR-2013-3p inhibition (antagomir) group (), the relative ATP content was extremely significantly increased in the coelomocytes of S. intermedius after pathogen infection (). Meanwhile, the relative expression of SinGDH (mRNA and protein) and the relative total SinGDH enzyme activity exhibited generally increasing trends in the coelomocytes of S. intermedius after pathogen infection ().
Discussion
In this study, a novel homologous HIF-1α gene (SinHIF-1α) was identified and characterized in the sea urchin S. intermedius. The targeting relationship between miR-2013-3p and SinHIF-1α and their functions in the regulation of ATP production in sea urchins were systematically clarified. Moreover, the potential mechanism by which the miR-2013-3p/SinHIF-1α axis regulates ATP production during pathogen infection in sea urchins was investigated in this study.
Characteristic domain analysis revealed that the SinHIF-1α and HIF-1α proteins of 28 other eukaryotic organisms shared relatively high homology in the bHLH domain (81.29%) and the PAS domains (PAS-A, 64.32%; PAS-B, 66.85%). Although SinHIF-1α and the HIF-1α proteins of S. purpuratus and C. elegans shared the same number and type of characteristic domains [Citation50,Citation51], there was variation in the characteristic domains between SinHIF-1α and the HIF-1α proteins of other organisms. Specifically, SinHIF-1α lacks the PAC domain in shrimp HIF-1α protein and three types of domains (ODD, N-TAD, and C-TAD) in fish HIF-1α proteins [Citation6,Citation52–54]. Taken together, the characteristic domain analysis results suggest that the bHLH, PAS-A, and PAS-B domains are highly conserved in both vertebrates and invertebrates. Moreover, species-specific characteristic domains (such as PAC, ODD, N-TAD, and C-TAD) in HIF-1α proteins may be important clues as to the species-specific functions of HIF-1α proteins, and these domains may be evidence of a molecular evolutionary footprint.
The phylogenetic analysis suggested that SinHIF-1α was clustered with HIF-1α proteins from S. purpuratus and A. japonicus. Moreover, the cluster containing SinHIF-1α was located in the middle of HIF-1α clusters between invertebrates and vertebrates, indicating the evolutionary transitional position of SinHIF-1α. All of the above findings were not only consistent with the traditional taxonomic status of these species but also provide novel clues for the evolutionary position of HIF-1α homologs from the perspective of biological macromolecules [Citation55].
As a critical transcription factor in regulating multiple cellular processes, HIF-1α expression can be detected in both normoxic and hypoxic conditions in different tissues of organisms [Citation16,Citation56–58]. Similarly, ubiquitous expression of SinHIF-1α (mRNA and protein) was observed in all examined tissues in this study. Moreover, tissue-specific variation in the expression of SinHIF-1α (mRNA and protein) was also observed in this study, similar to the results from fishes [Citation53], molluscs [Citation59], and crustaceans [Citation52]. The findings support the hypothesis that HIF-1α plays an important role in tissue homoeostasis, while the distinctive expression pattern implies its functional variation in different tissues [Citation16,Citation56]. Unlike many immune-related genes that have relatively high expression in immune-related tissues, relatively low expression of SinHIF-1α transcripts and protein was obtained under normoxic conditions in the coelomocytes (the main immune system) of S. intermedius. Similar observations were also found in the Atlantic croaker Micropogonias undulatus and the mudskipper Boleophthalmus pectinirostris [Citation53,Citation60]. Meanwhile, relatively high expression of HIF-1α homolog genes was observed in the immune tissues of S. paramamosain and the Mandarin fish Siniperca chuatsi; this was in contrast to the spatial expression pattern of SinHIF-1α observed in this study [Citation6,Citation61]. From the above, we speculate that the spatial expression pattern of HIF-1α homologs has species-specific variation and that whether a gene is involved in the immune defence response cannot be judged only by its expression level in the main immune tissues or systems.
The relatively lower expression of SinHIF-1α (mRNA and protein) in coelomocytes under normal conditions also suggested that there may be regulators in the coelomocytes of S. intermedius that inhibit the accumulation or promote the degradation of SinHIF-1α. Combined with our bioinformatics-derived prediction that miR-2013-3p has a potential targeting relationship with SinHIF-1α, we therefore hypothesized that miR-2013-3p may negatively regulate the expression of SinHIF-1α in the coelomocytes of S. intermedius. From the spatial expression pattern, opposite relative expression trends were observed between miR-2013-3p and SinHIF-1α (mRNA and protein) in all examined tissues. The relative expression trends of miR-2013-3p and SinHIF-1α reflected a potential negative regulatory relationship that is consistent with the conserved mechanism of miRNA regulation [Citation62]. The luciferase reporter assay data confirmed that miR-2013-3p attenuates the expression of SinHIF-1α by binding its 3’-UTR. As the relative expression of miRNAs needed to be higher than those of their target genes matching the conserved regulatory relationship between miRNA and its target genes [Citation41,Citation45,Citation49,Citation63–65], in this study, the relative expression of miR-2013-3p was higher than that of SinHIF-1α in the coelomocytes and peristomial membrane indicating that miR-2013-3p may specifically regulate the expression of SinHIF-1α in the coelomocytes and peristomial membrane in S. intermedius. This observation also indicates that the regulatory function of miRNAs could be tissue-specific. Since coelomocytes are the primary cells managing the innate immunity of sea urchins [Citation66], the result obtained in this study also suggests that the miR-2013-3p/SinHIF-1α axis may be involved in innate defence via affecting the physiological processes of coelomocytes in S. intermedius.
In addition to the regulation of low oxygen adaptability of organisms, the immunity regulation function of HIF-1α against pathogenic infections has gained increasing attention in recent years [Citation67,Citation68]. Similarly, whether SinHIF-1α is involved in pathogenic infection defence is one of the core concerns of this study. Fluctuating expression of SinHIF-1α (mRNA and protein) was observed in coelomocytes after pathogen infection, similar to results from existing research [Citation6,Citation53,Citation61], suggesting pathogen-induced alteration of the expression of SinHIF-1α in S. intermedius. Notably, linear downregulation of SinHIF-1α (mRNA and protein) occurred from 6 to 24 hpi and from 48 to 72 hpi, consistent with the upregulation periods of miR-2013-3p expression. Based on the above observation that miR-2013-3p negatively regulates the expression of SinHIF-1α in coelomocytes, we assume that the attenuated SinHIF-1α expression in coelomocytes during pathogenic infection was due to miR-2013-3p reducing the stability of SinHIF-1α mRNA by binding to its 3’-UTR. Further functional analysis also revealed that SinHIF-1α (mRNA and protein) expression was continuously enhanced by inhibiting the expression of miR-2013-3p in coelomocytes after pathogen infection (). This observation not only confirmed our hypothesis that SinHIF-1α was involved in pathogenic defence process via interacting with miR-2013-3p but also suggested that the miR-2013-3p/SinHIF-1α axis could be one of the targets of sea urchin pathogens.
Figure 8. Schematic diagrams of miR-2013-3p and SinHIF-1α involved in host-pathogen interaction of Strongylocentrotus intermedius. α-KG: alpha-ketoglutarate; TCA: tricarboxylic acid cycle; LPS: lipopolysaccharide.
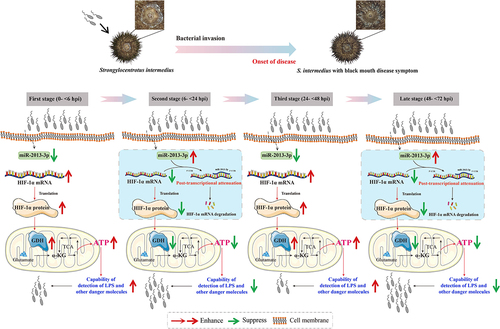
If the miR-2013-3p/SinHIF-1α axis is the target of sea urchin pathogens (black peristomial membrane disease in this study), then how does the pathogen utilize the miR-2013-3p/SinHIF-1α axis to regulate the defence mechanisms of the host (sea urchins in this study) and achieve infection? It has been documented that HIF homologous genes are expressed in normal tissues for inducing the expression of genes that are necessary to provide the cellular ATP (the major energy source) requirements [Citation56]. In addition, cellular ATP release is considered an essential signal for the detection of lipopolysaccharide (LPS) and other harmful molecules by monocytes and macrophages in Homo sapiens [Citation69]. A previous study reported that ATP synthesis could be suppressed in haemocytes and gill cells of the shrimp Litopenaeus vannamei after WSSV infection [Citation70]. Furthermore, Wang et al. proposed that activation of HIF-1α enhanced the antimicrobial functions of haemocytes through increasing energy production in S. paramamosain [Citation61]. Based on the above findings, we thus hypothesized that sea urchin pathogens may regulate the miR-2013-3p/SinHIF-1α axis to repress ATP production in the coelomocytes of S. intermedius and thereby achieve successful infection. As we expected, the trend in ATP content was consistent with the trend of SinHIF-1α expression but opposite to that of miR-2013-3p expression in the coelomocytes of S. intermedius after pathogen infection. The functional analysis also confirmed that the ATP content in coelomocytes was reduced after knocking down SinHIF-1α expression or following overexpression of miR-2013-3p. Taken together, the results suggest that the pathogen utilizes the miR-2013-3p/SinHIF-1α axis from 6 to 24 hpi and from 48 to 72 hpi to reduce ATP production and thereby attenuate the antimicrobial capability of the host (). Typically, the enhancement of expression and activity of key enzymes of glycolysis are key nodes linking HIF-1α homologous gene expression and ATP production under hypoxic conditions in organisms [Citation61,Citation71–73]. In this study, we found that changes in GDH expression and enzyme activity were consistent with the trend of SinHIF-1α expression but opposite to that of miR-2013-3p in the coelomocytes of S. intermedius after pathogen infection under normoxic conditions. GDH catalyzes the reversible reaction between α-ketoglutaric acid and glutamate; this reaction is considered one of the key nodes of the tricarboxylic acid cycle (TCA cycle) and amino acid metabolism [Citation74]. Since the TCA cycle is the most energy-producing metabolic pathway [Citation75], we therefore supposed that HIF-1α homologs could regulate cellular ATP production via targeting GDH under normoxic conditions. The functional analysis revealed that SinGDH expression and enzyme activity in coelomocytes were reduced by knocking down the expression of SinHIF-1α or overexpression of miR-2013-3p under normoxic conditions, further supporting the above hypothesis. To summarize, we suggest that the pathogen utilizes the miR-2013-3p/SinHIF-1α axis from 6 to 24 hpi and from 48 to 72 hpi to reduce ATP production via targeting SinGDH expression and enzyme activity to attenuate the antimicrobial capability of the host under normoxic conditions ().
Conclusions
In this study, a novel HIF-1α homolog in S. intermedius (SinHIF-1α) was identified and characterized. The negative regulatory targeting relationship between SinHIF-1α and miR-2013-3p was validated. The miR-2013-3p/SinHIF-1α axis is involved in the defence against peristomial membrane disease pathogen infection by regulating ATP production via targeting GDH. The miR-2013-3p/SinHIF-1α axis may be a key target of pathogen infection in sea urchins; this indicates the need for increased attention in the prevention and control of diseases of cultured sea urchins in the future. Specifically, we could realize disease control by designing or mining miR-2013-3p inhibitors or HIF-1α homolog activators (or stabilizers) in sea urchin aquaculture. We could also mine valuable SNPs from the miR-2013-3p/SinHIF-1α axis to develop new molecular assistive selective breeding markers for sea urchin cultivation. Generally speaking, the findings in this study have not only clarified the molecular mechanism of the regulatory functions of HIF-1α homologs under normoxic conditions but have also deepened our understanding of the pathogen defence response of economically important species of echinoderms. The observations from this study also enrich our knowledge of epigenetic regulator (miRNA) functions in innate immunity and provide new clues for developing disease control strategies for economic echinoderm aquaculture from the molecular aspect.
Authors’ contributions
YYZ and YQC conceived and designed the experiments. BYN, JXS, DYC, and SXZ performed the experiments. YYZ, BYN, JXS, and YQC analysed the data. YYZ and BYN drafted the manuscript. All authors read and approved the manuscript.
Consent for publication
All authors provided their consent for publication.
Ethics approval and consent to participate
The approval of this study was granted by the Ethics Committee of Dalian Ocean University (Date 2023.12.4/No DLOU2023007).
Highlights
A novel HIF-1α homolog gene (SinHIF-1α) in Strongylocentrotus intermedius was cloned and characterized.
The targeting interaction relationship between SinHIF-1α and miR-2013-3p was verified.
Relative ATP content was decreased in coelomocytes by SinHIF-1α silencing or by miR-2013-3p overexpression.
SinHIF-1α silencing or miR-2013-3p overexpression repressed the relative expression and enzyme
SinHIF-1α silencing or miR-2013-3p overexpression repressed the relative expression and enzyme
Supplemental Material
Download MS Word (485.8 KB)Disclosure statement
No potential conflict of interest was reported by the author(s).
Data availability statement
Data openly available in a public repository. The data that support the findings of this study are openly available in GenBank accession No. OQ397583 (https://www.ncbi.nlm.nih.gov/nuccore/OQ397583) and Figshare accession No. 10.6084/m9.figshare.25896994 (https://figshare.com/articles/dataset/The_raw_data/25896994).
Supplemental data
Supplemental data for this article can be accessed online at https://doi.org/10.1080/21505594.2024.2367660.
Additional information
Funding
References
- Cai X, Zhou Z, Zhu J, et al. Zebrafish HIF-3α modulates erythropoiesis via regulation of gata1 to facilitate hypoxia tolerance. Development. 2020;147(22):dev185116. doi: 10.1242/dev.185116
- Choudhry H, Harris AL. Advances in hypoxia-inducible factor biology. Cell Metab. 2018;27(2):281–17. doi: 10.1016/j.cmet.2017.10.005
- Law SH, Wu RS, Ng PK, et al. Cloning and expression analysis of two distinct HIF-alpha isoforms – gcHIF-1alpha and gcHIF-4alpha – from the hypoxia-tolerant grass carp, Ctenopharyngodon idellus. BMC Mol Biol. 2006;7(1):15. doi: 10.1186/1471-2199-7-15
- Rissanen E, Tranberg HK, Sollid J, et al. Temperature regulates hypoxia-inducible factor-1 (HIF-1) in a poikilothermic vertebrate, crucian carp (Carassius carassius). J Exp Biol. 2006;209(Pt6):994–1003. doi: 10.1242/jeb.02103
- Poyya J, Joshi CG, Kumar DJ, et al. Sequence analysis and phylogenetic studies of hypoxia-inducible factor-1α. Cancer Inform. 2017;16:1176935117712242. doi: 10.1177/1176935117712242
- He J, Yu Y, Qin XW, et al. Identification and functional analysis of the Mandarin fish (Siniperca chuatsi) hypoxia-inducible factor-1α involved in the immune response. Fish Shellfish Immunol. 2019;92:141–150. doi: 10.1016/j.fsi.2019.04.298
- Lin Y, Miao LH, Liu B, et al. Molecular cloning and functional characterization of the hypoxia-inducible factor-1α in bighead carp (Aristichthys nobilis). Fish Physiol Biochem. 2021;47(2):351–364. doi: 10.1007/s10695-020-00917-2
- Ramberg S, Høyheim B, Østbye TK, et al. A de novo full-length mRNA transcriptome generated from hybrid-corrected pacbio long-reads improves the transcript annotation and identifies thousands of novel splice variants in Atlantic Salmon. Front Genet. 2021;12:656334. doi: 10.3389/fgene.2021.656334
- Li T, Brouwer M. Hypoxia-inducible factor, gsHIF, of the grass shrimp Palaemonetes pugio: molecular characterization and response to hypoxia. Comp Biochem Phys B. 2007;147(1):11–19. doi: 10.1016/j.cbpb.2006.12.018
- Soñanez-Organis JG, Peregrino-Uriarte AB, Gómez-Jiménez S, et al. Molecular characterization of hypoxia inducible factor-1 (HIF-1) from the white shrimp Litopenaeus vannamei and tissue-specific expression under hypoxia. Comp Biochem Phys C. 2009;150(3):395–405. doi: 10.1016/j.cbpc.2009.06.005
- Piontkivska H, Chung JS, Ivanina AV, et al. Molecular characterization and mRNA expression of two key enzymes of hypoxia-sensing pathways in eastern oysters Crassostrea virginica (Gmelin): hypoxia-inducible factor α (HIF-α) and HIF-prolyl hydroxylase (PHD). Comp Biochem Phys D. 2011;6(2):103–114. doi: 10.1016/j.cbd.2010.10.003
- Kawabe S, Yokoyama Y. Role of hypoxia-inducible factor α in response to hypoxia and heat shock in the Pacific oyster Crassostrea gigas. Mar Biotechnol (NY). 2012;14(1):106–119. doi: 10.1007/s10126-011-9394-3
- Cai X, Huang Y, Zhang X, et al. Cloning, characterization, hypoxia and heat shock response of hypoxia inducible factor-1 (HIF-1) from the small abalone Haliotis diversicolor. Gene. 2014;534(2):256–264. doi: 10.1016/j.gene.2013.10.048
- Wang S, Zhang J, Jiao W, et al. Scallop genome provides insights into evolution of bilaterian karyotype and development. Nat Ecol Evol. 2017;1(5):120. doi: 10.1038/s41559-017-0120
- Rimoldi S, Terova G, Ceccuzzi P, et al. HIF-1α mRNA levels in Eurasian perch (perca fluviatilis) exposed to acute and chronic hypoxia. Mol Biol Rep. 2012;39(4):4009–4015. doi: 10.1007/s11033-011-1181-8
- Mohindra V, Tripathi RK, Singh RK, Lal KK. Molecular characterization and expression analysis of three hypoxia-inducible factor alpha subunits, HIF-1α, -2α and -3α in hypoxia-tolerant Indian catfish, Clarias batrachus [Linnaeus, 1758]. Mol Biol Rep. 2013;40(10):5805–5815. doi: 10.1007/s11033-013-2685-1
- Zhang G, Zhao C, Wang Q, et al. Identification of HIF-1 signaling pathway in pelteobagrus vachelli using RNA-Seq: effects of acute hypoxia and reoxygenation on oxygen sensors, respiratory metabolism, and hematology indices. J Comp Physiol B. 2017;187(7):931–943. doi: 10.1007/s00360-017-1083-8
- Sun JL, Zhao LL, Wu H, et al. Acute hypoxia changes the mode of glucose and lipid utilization in the liver of the largemouth bass (Micropterus salmoides). Sci Total Environ. 2020;713:135157. doi: 10.1016/j.scitotenv.2019.135157
- Lee P, Chandel NS, Simon MC. Cellular adaptation to hypoxia through hypoxia inducible factors and beyond. Nat Rev Mol Cell Biol. 2020;21(5):268–283. doi: 10.1038/s41580-020-0227-y
- Hochachka P, Buck L, Doll C, et al. Unifying theory of hypoxia tolerance: molecular/metabolic defense and rescue mechanisms for surviving oxygen lack. Proc Natl Acad Sci U S A. 1996;93(18):9493–9498. doi: 10.1073/pnas.93.18.9493
- Lutz PL, Nilsson GE, Prentice HM. The brain without oxygen: causes of failure-physiological and molecular mechanisms for survival. Kluwer Academic; 1994. doi: 10.16958/drsr.2014.12.3.227
- Nilsson GE, Ostlund-Nilsson S. Does size matter for hypoxia tolerance in fish. Biol Rev Camb Philos Soc. 2008;83(2):173–189. doi: 10.1111/j.1469-185X.2008.00038.x
- Yang S, Yan T, Wu H, et al. Acute hypoxic stress: effect on blood parameters, antioxidant enzymes, and expression of HIF-1alpha and GLUT-1 genes in largemouth bass (Micropterus salmoides). Fish Shellfish Immunol. 2017;67:449–458. doi: 10.1016/j.fsi.2017.06.035
- Chen X, Liu Z, Gu Y, et al. A hexokinase from the oyster Crassostrea gigas is involved in immune recognition as a pattern recognition receptor. Dev Comp Immunol. 2021;122:104083. doi: 10.1016/j.dci.2021.104083
- Brahimi-Horn MC, Pouysségur J. HIF at a glance. J Cell Sci. 2009;122(Pt8):1055–1057. doi: 10.1242/jcs.035022
- So JH, Kim JD, Yoo KW, et al. FIH-1, a novel interactor of mindbomb, functions as an essential anti-angiogenic factor during zebrafish vascular development. PLoS One. 2014;9(10):e109517. doi: 10.1371/journal.pone.0109517
- Bracken CP, Whitelaw ML, Peet DJ. The hypoxia-inducible factors: key transcriptional regulators of hypoxic responses. Cell Mol Life Sci. 2003;60(7):1376–1393. doi: 10.1007/s00018-003-2370-y
- Lawrence JM. Sea urchin life-history strategies. Sea Urchins: biol And Ecol. 2020;43:19–28. doi: 10.1016/b978-0-12-819570-3.00002-0
- Smith LC, Clow LA, Terwilliger DP. The ancestral complement system in sea urchins. Immunol Rev. 2001;180(1):16–34. doi: 10.1034/j.1600-065x.2001.1800102.x
- Smith LC. Diversification of innate immune genes: lessons from the purple sea urchin. Dis Model Mech. 2010;3(5–6):274–279. doi: 10.1242/dmm.004697
- Buchmann K. Evolution of innate immunity: clues from invertebrates via fish to mammals. Front Immunol. 2014;5:459. doi: 10.3389/fimmu.2014.00459
- Zhang Q, Cao X. Epigenetic remodeling in innate immunity and inflammation. Annu Rev Immunol. 2021;39(1):279–311. doi: 10.1146/annurev-immunol-093019-123619
- Auguste M, Melillo D, Corteggio A, et al. Methodological approaches to assess innate immunity and innate memory in marine invertebrates and humans. Front Toxicol. 2022;4:842469. doi: 10.3389/ftox.2022.842469
- Miranda-Cruz MM, Poom-Llamas JJ, Godoy-Lugo JA, et al. Silencing of HIF-1 in WSSV-infected white shrimp: effect on viral load and antioxidant enzymes. Comp Biochem Phys C. 2018;213:19–26. doi: 10.1016/j.cbpc.2018.07.004
- Cao Y, Fang T, Du Y, et al. MiR-2013 negatively regulates phylogenetically conserved PIP5K involved in TLR4 mediated immune responses of amphioxus (Branchiostoma belcheri Tsingtaunese). Dev Comp Immunol. 2022;133:104430. doi: 10.1016/j.dci.2022.104430
- Thomas JR, Lee A. Measuring Ca2+-dependent modulation of voltage-gated Ca2+ channels in HEK-293T cells. Cold Spring Harb Protoc. 2016;2016(9):db.prot087213. doi: 10.1101/pdb.prot087213
- Mügge FLB, Morlock GE. Planar bioluminescent cytotoxicity assay via genetically modified adherent human reporter cell lines, applied to authenticity screening of Saussurea costus root. J Chromatogr A. 2022;1683:463522. doi: 10.1016/j.chroma.2022.463522
- Liu Z, Ma F, Kang Y, et al. Gene ssa-MiR-301a-3p improves rainbow trout (Oncorhynchus mykiss) resistance to heat stress by targeting hsp90b2. PeerJ. 2022;10:e13476. doi: 10.7717/peerj.13476
- He L, Zhao M, Yu X, et al. MicroRNA-182-3p negatively regulates cytokines expression by targeting TLR5M in orange-spotted grouper, Epinephelus coioides. Fish Shellfish Immunol. 2019;93:589–596. doi: 10.1016/j.fsi.2019.07.063
- Ren L, Li L, Zhan Y, et al. Isolation of a new PAK1 gene from sea cucumber (Apostichopus japonicus) and its expression analysis and function characterization. J Ocean Univ China. 2019;18(5):1147–1157. doi: 10.1007/s11802-019-4034-z
- Liu L, Zhao T, Lin K, et al. Identification of a novel RhoA gene in the sea cucumber Apostichopus japonicus and its immune regulatory function via interacting with MiR-2012-5p. Int j biol macromol. 2022;203:572–582. doi: 10.1016/j.ijbiomac.2022.01.176
- Ryu TK, Lee G, Rhee Y, et al. Identification of nickel response genes in abnormal early developments of sea urchin by differential display polymerase chain reaction. Ecotoxicol Environ Saf. 2012;84:18–24. doi: 10.1016/j.ecoenv.2012.06.018
- Cui D, Liu L, Zhao T, et al. Responses of sea urchins (Strongylocentrotus intermedius) with different sexes to CO2-induced seawater acidification: histology, physiology, and metabolomics. Mar Pollut Bull. 2022;178:113606. doi: 10.1016/j.marpolbul.2022.113606
- Guo C, Zhang Z, Zhang M, et al. Screening and stability analysis of reference genes for gene expression normalization in hybrid yellow catfish (Pelteobagrus fulvidraco ♀ × Pelteobagrus vachelli ♂) fed diets containing different soybean meal levels. Aquac Nutr. 2023;2023:1–17. doi: 10.1155/2023/1232518
- Jiao R, Wu B, Han S, et al. miRn-3 inhibits cutaneous wound healing by targeting gelsolin in the sea cucumber Apostichopus japonicus. Int J Biol Macromol. 2024;254(Pt 2):127801. doi: 10.1016/j.ijbiomac.2023.127801
- Livak KJ, Schmittgen TD. Analysis of relative gene expression data using real-time quantitative PCR and the 2−ΔΔct method. Methods. 2001;25(4):402–408. doi: 10.1006/meth.2001.1262
- Zhao T, Ren L, Li C, et al. MiR-7 regulates pathogen-induced immune response via PAK1 in the sea cucumber Apostichopus japonicus. Front Immunol. 2022;13:927796. doi: 10.3389/fimmu.2022.927796
- Li C, Zhao T, Ren L, et al. Characterization of a novel glutamate dehydrogenase gene and its response to heat stress in the sea urchin Strongylocentrotus intermedius. Aquacult Rep. 2023;28:101446. doi: 10.1016/j.aqrep.2022.101446
- Zhao T, Liu L, Li C, et al. Isolation and characterization of four pathogenic strains of Vibrio spp. From farmed sea urchins. Strongylocentrotus Intermedius, China Microb Pathog. 2022;169:105651. doi: 10.1016/j.micpath.2022.105651
- Wang T, Meng J, Li L, et al. Characterization of CgHIFα-Like, a novel bHLH-PAS transcription factor family member, and its role under hypoxia stress in the pacific oyster Crassostrea gigas. PLoS One. 2016;11(11):e0166057. doi: 10.1371/journal.pone.0166057
- Jiang H, Guo R, Powell-Coffman JA. The Caenorhabditis elegans hif-1 gene encodes a bHLH-PAS protein that is required for adaptation to hypoxia. Proc Natl Acad Sci U S A. 2001;98(14):7916–7921. doi: 10.1073/pnas.141234698
- Okamura Y, Mekata T, Elshopakey GE, et al. Molecular characterization and gene expression analysis of hypoxia-inducible factor and its inhibitory factors in kuruma shrimp Marsupenaeus japonicus. Fish Shellfish Immunol. 2018;79:168–174. doi: 10.1016/j.fsi.2018.05.015
- Guan F, Lu XJ, Li CH, et al. Molecular characterization of mudskipper (Boleophthalmus pectinirostris) hypoxia-inducible factor-1α (HIF-1α) and analysis of its function in monocytes/macrophages. PLoS One. 2017;12(5):e0177960. doi: 10.1371/journal.pone.0177960
- Wang C, Wu X, Hu X, et al. Hypoxia-inducible factor 1α from a high-altitude fish enhances cytoprotection and elevates nitric oxide production in hypoxic environment. Fish Physiol Biochem. 2020;46(1):39–49. doi: 10.1007/s10695-019-00694-7
- National Center for Biotechnology Information. National Library of Medicine. NCBI. 2023. Available from: https://www.ncbi.nlm.nih.gov/taxonomy
- Stroka DM, Burkhardt T, Desbaillets I, et al. HIF-1 is expressed in normoxic tissue and displays an organ-specific regulation under systemic hypoxia. FASEB J. 2001;15(3):2445–2453. doi: 10.1096/fj.01-0125com
- Chen N, Chen LP, Zhang J, et al. Molecular characterization and expression analysis of three hypoxia-inducible factor alpha subunits, HIF-1α/2α/3α of the hypoxia-sensitive freshwater species, Chinese sucker. Gene. 2012;498(1):81–90. doi: 10.1016/j.gene.2011.12.058
- Liu S, Zhu K, Chen N, et al. Identification of HIF-1α promoter and expression regulation of HIF-1α gene by LPS and hypoxia in zebrafish. Fish Physiol Biochem. 2013;39(5):1153–1163. doi: 10.1007/s10695-013-9771-0
- Wang SY, Lau K, Lai KP, et al. Hypoxia causes transgenerational impairments in reproduction of fish. Nat Commun. 2016;7(1):12114. doi: 10.1038/ncomms12114
- Rahman MS, Thomas P. Molecular cloning, characterization and expression of two hypoxia-inducible factor alpha subunits, HIF-1alpha and HIF-2alpha, in a hypoxia-tolerant marine teleost, Atlantic croaker (Micropogonias undulatus). Gene. 2007;396(2):273–282. doi: 10.1016/j.gene.2007.03.009
- Wang J, Xu Z, He J. The role of HIF-1α in the energy metabolism and immune responses of hypoxic Scylla paramamosain. Aquacult Rep. 2021;20:100740. doi: 10.1016/j.aqrep.2021.100740
- Carthew RW, Sontheimer EJ. Origins and mechanisms of miRnas and siRnas. Cell. 2009;136(4):642–655. doi: 10.1016/j.cell.2009.01.035
- Li C, Zhao M, Zhang C, et al. MiR210 modulates respiratory burst in Apostichopus japonicus coelomocytes via targeting Toll-like receptor. Dev Comp Immunol. 2016;65:377–381. doi: 10.1016/j.dci.2016.08.008
- Zhang Y, Shao Y, Lv Z, et al. MiR-210 regulates coelomocyte proliferation through targeting E2F3 in Apostichopus japonicus. Fish Shellfish Immunol. 2020;106:83–590. doi: 10.1016/j.fsi.2020.08.036
- Chen K, Shao Y, Li C. MiR-137 modulates coelomocytes autophagy by targeting Atg13 in the sea cucumber Apostichopus japonicus. Dev Comp Immunol. 2022;135:104486. doi: 10.1016/j.dci.2022.104486
- Coates CJ, McCulloch C, Betts J, et al. Echinochrome a release by red spherule cells is an iron-withholding strategy of sea urchin innate immunity. J Innate Immun. 2018;10(2):119–130. doi: 10.1159/000484722
- Palazon A, Goldrath AW, Nizet V, et al. HIF transcription factors, inflammation, and immunity. Immunity. 2014;41(4):518–528. doi: 10.1016/j.immuni.2014.09.008
- Krzywinska E, Stockmann C. Hypoxia, metabolism and immune cell function. Biomedicines. 2018;6(2):56. doi: 10.3390/biomedicines6020056
- Lee AH, Ledderose C, Li X, et al. Adenosine triphosphate release is required for toll-like receptor-induced monocyte/macrophage activation, inflammasome signaling, interleukin-1β production, and the host immune response to infection. Crit Care Med. 2018;46(12):e1183–e1189. doi: 10.1097/CCM.0000000000003446
- Liang Y, Xu ML, Wang XW, et al. ATP synthesis is active on the cell surface of the shrimp Litopenaeus vannamei and is suppressed by WSSV infection. Virol J. 2015;12(1):49. doi: 10.1186/s12985-015-0275-7
- Courtnay R, Ngo DC, Malik N, et al. Cancer metabolism and the Warburg effect: the role of HIF-1 and PI3K. Mol Biol Rep. 2015;42(4):841–851. doi: 10.1007/s11033-015-3858-x
- Kierans SJ, Taylor CT. Regulation of glycolysis by the hypoxia-inducible factor (HIF): implications for cellular physiology. J Physiol. 2021;599(1):23–37. doi: 10.1113/JP280572
- Chen ML, Cheng T, Xu C, et al. Sodium houttuyfonate enhances the mono-therapy of fluconazole on oropharyngeal candidiasis (OPC) through HIF-1α/IL-17 axis by inhibiting cAMP mediated filamentation in Candida albicans-Candida glabrata dual biofilms. Virulence. 2022;13(1):428–443. doi: 10.1080/21505594.2022.2035066
- Yin X, Peng J, Gu L, et al. Targeting glutamine metabolism in hepatic stellate cells alleviates liver fibrosis. Cell Death Dis. 2022;13(11):955. doi: 10.1038/s41419-022-05409-0
- Yang S, Zhao J, Cui X, et al. TCA-phospholipid-glycolysis targeted triple therapy effectively suppresses ATP production and tumor growth in glioblastoma. Theranostics. 2022;12(16):7032–7050. doi: 10.7150/thno.74197