ABSTRACT
Lipid-modified GTPases in the Ras superfamily that mediate a variety of cell signaling processes were thought to be passively anchored to membranes. However, an increasing number of recent studies are finding that membrane binding of these proteins is hardly a passive process, and it involves the soluble catalytic domain as well as the lipid anchor. The catalytic domain adopts multiple orientations on the membrane surface due to internal fluctuations that are modulated by activation status and mutations. Distinct orientation preferences among small GTPases likely lead to differential signaling outcomes, as downstream effectors can sense different orientations. We review recent studies behind this important conclusion.
Introduction
The plasma membrane (pm) is made up of a large number of lipids—predominantly phospholipids—and a variety of proteins that either span the membrane thickness or attach peripherally to the surface.Citation1 Some membrane proteins are constitutively membrane associated while others (e.g. lipidated GTPases and kinases) bind conditionally and shuttle between the cytosol and the plasma membrane.Citation2 Peripheral membrane proteins such as the pleckstrin homology (PH) domainCitation3 harbor lipid-binding pockets for recognition of specific pm lipids.Citation2,4 In contrast, post-translationally lipid-modified proteins do not have a defined pocket for lipid binding.Citation5 As a result, it was thought that this class of proteins interacts with the pm through non-specific hydrophobic protein-lipid interactions involving only the lipid-modified N- or C-terminus, occasionally complemented by generalized electrostatic complementarity between the surface of the host membrane and the lipid anchor (note: lipid modification is important for binding to other membranes as well but here we focus only on its role in pm binding). However, a number of recent studies on several lipidated proteins in the Ras superfamily found that their membrane-interaction involves the soluble catalytic domain (G-domain) in addition to the lipid anchor.Citation6-15 The catalytic domain-lipid interactions appear to be semi-specific and involve a few selected residues outside the terminal lipid anchor (e.g., refs. Citation7, 8, 13, 15). These interactions can be modulated by the bound nucleotideCitation8,13,14 or activation status of the proteinCitation8,12,13 as well as by mutations.Citation13 Conversely, the host membrane modulates the function of these proteins.Citation6-8,11-16 We review recent developments that gave rise to these conclusions focusing on the Ras superfamily of proteins.
Lipid modification of Ras superfamily proteins
The Ras superfamily of guanosine triphosphate (GTPases) is comprised of a large number of molecular switches involved in signaling pathways controlling many different critical cellular events.Citation5,17-19 These include cell growth, motility, trafficking and other functions.Citation5,17,18 The Ras superfamily has 154 members grouped into 5 families: Ras, Arf, Rab, Rho and Ran ().Citation5,20-24 The Rab family represents the largest branchCitation24,25 with more than 60 members.Citation24,26 They undergo posttranslational geranylgeranylation at one or more Cys residues near or at the C-terminus.Citation24,27 Members of the Ras family contain a highly conserved G-domain and a C-terminal hypervariable region (HVR) that is isoprenylated at the C-terminus for association with the pm.Citation28,29 Prototypical examples of the Ras family members include Ras (including the H-, N-, K-Ras isoforms), Ral (Ras-like) and Rheb (Ras homolog enriched in brain).Citation5,19,20 The nature of lipidation is believed to contribute to functional specialization.Citation10,30 For instance, all H-, N- and K-Ras are farnesylated but only N- and H-Ras are palmitoylated at one and 2 cysteine residues, respectively, whereas K-Ras has 6 lysine residues immediately upstream of the farnesylated cysteine;Citation20 these differences partially explain the observed lateral segregation of Ras isoforms into different lipid micro-domains on the pm and thereby their functional diversity.Citation6,10,30-33 Arf (ADP-ribosylation factor) family comprises Arf proteins (Arf1/Arf2/Arf3 (class 1), Arf4/Arf5 (class 2) and Arf6 (class 2)),Citation23 Arf-like proteins (Arl) and Sar1, and almost all contain a myristoylated amphipathic N-terminal α-helix, except for Sar and some Arl that lack lipid-modification or are acetylated.Citation23 The Rho family is sub-classified into 6 subfamilies: Rac1, Rnd3, TC10, RhoB, RhoBTB and Miro.Citation22 Rac1, Rnd3, TC10 and RhoB are C-terminally farnesylated or geranylgeranylated, with some harboring additional fatty acid modification(s). RhoBTB and Miro do not undergo posttranslational lipid modification.Citation22 Similarly, Ran does not have a posttranslational lipid modification sequence motif and therefore resides in the cytosol or the nucleus.Citation34
Conformational dynamics of Ras superfamily proteins
Members of the Ras superfamily share many structural and mechanistic features. Their structure consists of a catalytic G-domain containing a nucleotide-binding site (NBS) and switch loops that interact with a variety of upstream and downstream binding partners (). They are “on” or active when the NBS is occupied by guanosine triphosphate (GTP) and “off” or inactive when GTP is hydrolyzed to guanosine diphosphate (GDP).Citation29,35 The on/off cycling is tightly regulated by the activity of guanine nucleotide exchange factors (GEFs) and GTPase activating proteins (GAPs), respectively.Citation36,37 Finally, most members of the Ras superfamily can be aberrantly regulated or rendered constitutively active by, among other factors, somatic point mutations (e.g. Ras) or overexpression (e.g., Rho).Citation38,39 This leads to a variety of diseases,Citation40-43 with some members playing critical roles in some of the deadliest cancers, such as K-Ras in pancreatic cancer.Citation44 Therefore, these lipidated small G-proteins represent an attractive, albeit challenging, drug targets.Citation37,45-48 The absence of marketed drugs that target this class of proteins underscores the need for further investigation. Of particular importance in this regard is the least understood role of membrane in their dynamics and function.
As described above, the majority of proteins in the Ras superfamily utilize a lipid-modified anchor at their N- (e.g. Arf) or C-terminus (e.g., Ras) for membrane binding ().Citation49,50 In some cases, for example in K-Ras, lipid modification is complemented by a stretch of positively charged residues that facilitates pm binding.Citation51 A flexible linker in the HVR connects the soluble G-domain with the lipid anchor. In contrast to the relatively well-understood phenomenon of nucleotide-dependent conformational change and its critical role in biological activity,Citation35 the functional significance of conformational changes upon membrane binding is less well understood. Moreover, conformational fluctuations in small GTPases are not limited to those accompanying GDP/GTP exchange. For instance, intrinsic fluctuations in the catalytic domain of GTP-bound H-, N- and K-Ras proteins lead to at least 2 distinct conformational sub-states,Citation52 with only one of these sub-states being able to activate effector proteins.Citation15,52 Moreover, long-range coupled motions modulate membrane binding, as documented by a number of recent computational and experimental studies.Citation53-56 It is important to emphasize that, in addition to the conformational dynamics of the GTPases themselves, the host membrane also exerts an important general effect on their activity. For example, the plasma membrane accelerates molecular interactions by facilitating productive encounters between the surface-bound GTPases and their soluble or membrane-bound reaction partners, thus providing a convenient platform for signaling events to take place. This has been the topic of several excellent recent reviewsCitation57-60 and is therefore not covered here in detail.
Conformational dynamics and membrane binding: Case studies of membrane orientation
A number of studies found that conformational dynamics of the most commonly studied Ras proteins,Citation10-13,15 Arf1Citation9 and RhebCitation14 leads to a direct interaction of the catalytic domain with membrane lipids. This interaction involves multiple distinct sets of—typically basic—surface residues located at different regions of the catalytic domain and the HVR. As a result, the catalytic domain can adopt multiple orientations with respect to the membrane plane. In some of these orientations, the reactive switch loops become occluded by the membrane and thus unavailable for engagement with effectors or exchange factors.Citation13-15 This suggests a novel mechanism of regulation whereby the switching function of these proteins is controlled not only by conformational change accompanying nucleotide exchange but also by the reorientation of the catalytic domain at the membrane surface. The latter is underpinned by the differential interaction of surface residues with membrane lipids, and has the capacity to control accessibility of the catalytic domain, particularly the functionally responsive switch loops, to interaction partners. The key studies that have provided the major milestones in the genesis of this conclusion are highlighted in , and are summarized below using the nomenclature of Ras proteins for the numbering of helices and β strands.Citation7-15
Figure 2. Orientational preferences of (A) GDP-bound (left panel) and GTP-bound (right panel) H-Ras and Rheb, and (B) GTP-bound Arf and K-Ras with respect to the membrane surface. Helices 3–5 are in red and β strands 1–3 in yellow. Membrane is shown in white surface representation.
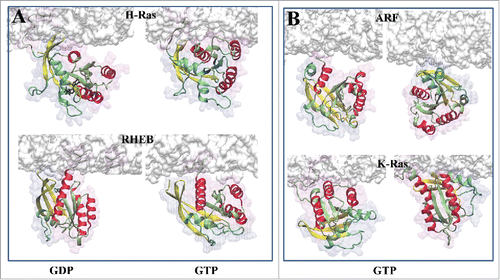
Figure 3. (A) Summary of G-domain-membrane interactions from the literature. (B) A proposed mechanism whereby membrane plays a key role in the switching function of lipidated small GTPases.
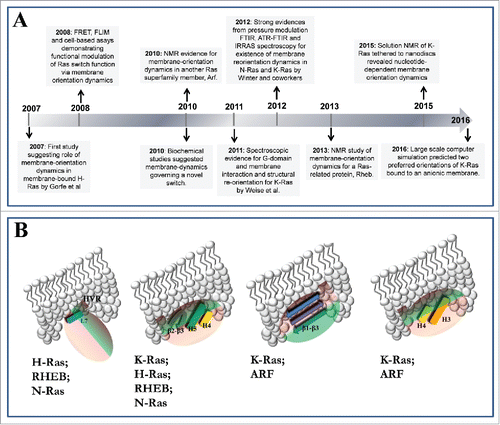
H-Ras
Along with N- and K-Ras, H-Ras is one of the 3 most common isoforms of Ras proteins within the Ras family. H-Ras attaches to the inner leaflet of the plasma membrane via a C-terminal lipid anchor that is farnesylated and dually palmitoylated. Membrane binding of H-Ras was thought to involve only the hydrophobic lipid anchor acting as non-specific glue that is connected to the soluble catalytic domain via a flexible linker. In 2007, we reported data from what was then the first of its kind molecular dynamics simulation of GDP- and GTP-bound full-length H-Ras bound to a DMPC model bilayer membrane.Citation8 The simulations were about 50ns long, which is rather short by current standards. Moreover, DMPC bilayer is a poor mimic of the highly heterogeneous plasma membrane. Despite these limitations, the simulations yielded completely unexpected insights into how an intricate interplay of fluctuations at the HVR and the G-domain of H-Ras can couple different nucleotide-dependent conformational changes with bilayer interaction. The study revealed not only the ability of the catalytic domain and the HVR to directly interact with lipids via positively charged surface amino acids but also the existence of 2 predominant membrane orientations ().Citation8 In GTP-bound H-Ras, R128 and R135 from helix 4 make direct contact with the membrane; conversely, HVR residues R169 and K170 interact with membrane in GDP-bound H-Ras.Citation8 This prediction was confirmed by mutagenesis using cell-based functional assays and FLIM/FRET (fluorescence lifetime imaging microscopy/Forster resonance energy transfer) measurements.Citation6,7 Alanine substitution of R169 and K170 in GTP H-Ras led to enhanced activity as measured by phospho-ERK levels, whereas mutation of the helix-4 residues R128 and R135 to alanine diminished H-Ras activity.Citation7,8,16 This consequence of disrupting the interaction of R128/R135 and R169/K170 with membrane was described by a “balance model,”Citation16 whereby perturbation of one pair of specific protein-lipid interaction stabilizes (or shifts the population toward) an orientation stabilized by the other pair. This observation suggests a direct link between the nature of the host membrane and the switching function of Ras, and led to the realization of a phenomenon that we refer to as membrane orientation dynamics. Subsequent to these landmark studies conducted in silico and in cells, a number of spectroscopic studies of N-Ras,Citation11 K-Ras,Citation13,15 RhebCitation14 and ArfCitation9 in synthetic bilayers or lipid nanodiscs provided support to the idea of membrane orientation dynamics (see below).
N-Ras
N-Ras has a farnesylated and palmitoylated C-terminus and, like H-Ras, harbors solvent exposed basic residues (all of them lysine) at positions 128 and 135 of helix 4 as well as 169 and 170 of the HVR. Therefore, its membrane orientation dynamics can be expected to be similar to those of H-Ras. This is supported by data from infrared reflection absorption spectroscopy (IRRAS),Citation10,12 atomic force microscopy (AFM),Citation12 and attenuated total reflectance Fourier transform infrared spectroscopy (ATR-FTIR) measurements.Citation11,12,62 Perhaps the most compelling evidence for a direct interaction of N-Ras catalytic domain with membrane, and more broadly Ras membrane orientation dynamics, came from a pressure perturbation study.Citation11 This work showed that, in addition to the 2 previously described conformational sub-states of GTP-bound Ras,Citation52 there exists a third state that is induced by membrane binding.Citation11 This was explained by a parallel membrane orientation of the catalytic domain helices that allows them to directly contact the bilayer. This conclusion matches exactly with one of the MD-derived orientations of H-Ras.Citation8 Studies with FTIR, ATR-FTIR and IRRACitation11,12 also found that GTP and GDP-bound N-Ras sample distinct conformational sub-states upon binding to synthetic membranes. In fact, the GDP and GTP sub-states were found to correspond to distinct catalytic domain membrane orientations, whereby helices 3–5 are roughly perpendicular and parallel to the bilayer plane, respectively. This suggests that N-Ras interacts with membrane in an activation-state dependent manner, emulating H-Ras.Citation8,11 The behavior of N-Ras orientation dynamics in cells appears to be more complicated and potentially different from both H- and K-Ras4B with respect to nanoscale clustering and effector domain engagement.Citation6,61 Moreover, it is important to note that both parallel and perpendicular membrane orientations are likely accessible to the GTP- and GDP-bound N- and H-Ras, with nucleotide exchange simply altering the population distribution resulting in a dominant orientation. Consistent with this hypothesis, GDP-bound N-Ras was found to sample parallel orientation during MD simulationsCitation62 whereas IR spectroscopic analysis of the same system embedded in a supported bilayer suggested a perpendicular orientation.Citation62 These 2 observations were reconciled if N-Ras forms a dimer, a notion that is attracting increasing attention but one outside the scope of this review.
K-RasB
The lipid anchor of K-Ras4B (here after K-Ras) is prenylated but not palmitoylated. Instead, pm binding is supplemented by electrostatic interactions with the head group of negatively charged lipids via a hexa-lysine segment immediately upstream of the farnesylated cysteine. Here we focus on the comparatively well-studied K-Ras4B, because its splice variant, K-Ras4A, has not yet been studied in terms of its membrane binding and orientation dynamics. (We speculate that the membrane reorientation of K-Ras4A may resemble N-Ras since both are singly palmitoylated and farnesylated, though K-Ras4A also contains basic residues.) Earlier MD simulations of a K-Ras homology model in a DMPC bilayer found no major conformational changes that would lead to membrane reorientation.Citation6 In addition to the short (30ns) length of those simulations and the lack of a high resolution X-Ray structure at the time, the study was also hampered by the use of a zwitterionic DMPC lipid bilayer while cellular K-Ras preferentially targets negatively charged membrane patches.Citation63 Recent experimental and computational studies in more realistic model membranes demonstrated that, just like H- and N-Ras, membrane-bound K-Ras undergoes substantial conformational dynamics and adopts 2 predominant membrane orientation states ().Citation15 Earlier spectroscopic studies from Roland Winter's laboratoryCitation12 arrived at a similar conclusion while a more recent work by Ikura and co-workersCitation13 using NMR provided evidence for a nucleotide dependent orientation change of K-Ras upon binding to lipid nanodiscs.Citation13 It was found that the dominant orientation of inactive K-Ras is similar to that of active H-Ras, with helices 4 and 5 roughly lying on the surface of the membraneCitation13 (). The functional significance of the globally opposite orientations of active/inactive H-Ras and K-Ras was discussed previously.Citation6 Interestingly, the effector-interacting switches were occluded by the membrane in one of the most populated orientations of GTP-bound K-Ras.Citation13 Similar results were obtained from microsecond scale MD simulations conducted on GTP-bound G12D K-Ras in complex with a POPC bilayer containing 20% POPS.Citation15 However, there were some critical differences. One was that a partially positively charged surface spanning helices 3 and 4 engages the bilayer in the simulations more frequently than helices 4 and 5, which were dominant in the NMR study. The other difference was that, in the simulations, orientation states 1 and 2Citation15 were nearly equally accessible to GTP-bound G12D K-Ras while in the NMR study one orientation appears to dominate. In orientation state 1, the effector-binding surface is solvent exposed and available for interaction but it is occluded by the bilayer in orientation state 2.Citation15 That both states are accessible to GTP K-Ras is consistent with the idea that membrane reorientation is driven by the intrinsic dynamics of the protein which in turn is consistent with previous observations about the substantial flexibility of GTP-bound K-Ras in solutionCitation64 as well as in membrane.Citation12 It is expected, however, that the intrinsic K-Ras dynamics would be modulated by the bound nucleotide or a mutation.
Rheb
Rheb is another member of the Ras family that is C-terminally farnesylated and anchors to the inner leaflet of the pm. NMR analysis of Rheb tethered to lipid nanodiscs provided the first direct experimental evidence – from a structural perspective – for both the direct interaction of the catalytic domain with membrane surfaces and the existence of at least 2 dominant orientations.Citation14 In this study, G-domain helices 3–5 were found to be predominantly oriented perpendicular to the bilayer plane when Rheb is GDP-bound. In the GTP-bound form, the helices adopted a parallel orientation (). In short, the membrane orientation dynamics of Rheb replicates almost exactly the reorientation of H-Ras on membrane surfaces in an activation state dependent manner.
Arf1
G-domain reorientation on membrane surfaces has also been reported for small GTPases other than those in the Ras family. One example is the ADP ribosylation factor (Arf).Citation9 The N- terminally myristoylated Arf is a regulator of the binding and release of membrane coat proteins.Citation23 A recent NMR study investigated the dynamics of the active (GTP-bound) myristoylated Arf bound to membrane mimetic bicelles composed of DMPC and DHPC lipids.Citation9 The study found 3 dominant ensembles where different regions of the catalytic domain and/or the HVR interact with the surface of the bicelle.Citation9 In order to illustrate the membrane orientation of the catalytic domain in these ensembles, we manually built membrane-bound Arf models based on the crystal structure of myristoylated full-length Arf1 structure (from yeast) and a POPC/POPS bilayer, guided by Figure 4 of ref. Citation9. The results shown in demonstrate the remarkable similarity between the membrane orientations of Arf1 and Ras catalytic domains. As in Ras, the switch loops are occluded by the bilayer in one orientation while they are solvent exposed and available for effector binding in the other orientations ().
Figure 4. (A) Structural regions of the G-domain or the HVR that are in direct contact with the plasma membrane in a non-effector interacting orientation (occluded) and effector interacting orientation (exposed). K-Ras.GDP (gray) is an outlier since, although inactive, it interacts directly with the membrane via helices 4 and 5 and turn β2-β3 loop. * Represents cases where turn β2-β3 and helix3 interact with membrane in the inactive state. The helices are numbered according to Ras protein i.e. H1 corresponds to helix1 etc. (B) Sequence alignment of G-domain helices 3–5 from ref. Citation5 for Arf1, Rheb and the 3 isoforms of Ras. Basic residues are in blue and acidic residues in red. The boxes to the right highlight the total number of positive (blue) and negative (red) charges as well as the net charge (black) on each helix.
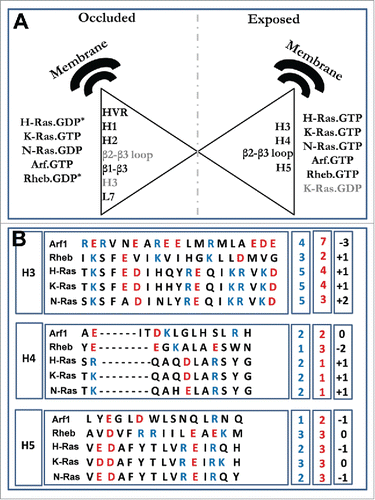
Origin and functional implication of membrane reorientation
At least 4 distinct membrane orientations of the G-domain have been observed thus far: (i) helices perpendicular to the membrane with few residues from the linker and/or the loop between helix 3 and helix 4 contacting lipid head groups; (ii) the C-terminal helices 4/5 and the turn β2-β3 in contact with the membrane in a parallel orientation; (iii) β-strands β1-β3 in direct contact with the membrane; (iv) helices 3/4 in direct contact and parallel to the membrane plane (). In each protein investigated thus far, however, only 2 of the 4 possible modes appear to be the dominant orientation states, with the other 2 sometimes acting as “intermediate” modes of membrane interaction as the protein transitions between the dominant orientations. More broadly, the examples described above demonstrate that lipidated small GTPases undergo substantial conformational changes upon membrane binding. Although the primary determinant of the different biological properties of H-, N- and K-Ras is likely the most divergent HVR, the second most divergent region, helix 4 (), also plays an important role in membrane reorientation. This has been demonstrated for Ras proteins in Abankwa et al.Citation6,16 Other divergent surface patches on lobe 2, particularly helices 3 and 5 that harbor several amino acid substitutions (), are also involved in membrane orientation dynamics. In fact, GDP-bound H-Ras, N-Ras and Rheb, as well as one of the 2 major orientation states of GTP-bound K-Ras, adopt an orientation in which their helices are roughly perpendicular to the membrane plane (). In contrast, GTP-bound H-Ras, N-Ras, Rheb and the other dominant orientation of GTP-bound K-Ras have their helices roughly parallel to and in contact with the membrane surface (). GTP-bound Arf1 adopts at least 2 distinct orientations that mirror GTP-bound K-Ras. Different sets of residues contact membrane in the active (i.e., able to interact with effector) and inactive or occluded orientations (): in the inactive state residues from the HVR, the switch loops and β-strands 1–3 contribute to G-domain-lipid interaction; in the active orientation, residues from helices 3/4 or 4/5 and turn β2-β3 interact with lipids (). Note that alternative orientations are also possible, examples being GDP-bound H-Ras and Rheb where helix 3 and/or β2-β3 loop makes close contact with the bilayer membrane ().
Table 1. Summary of the main observations from previous studies of membrane orientation dynamics of lipidated small GTPases.
The conformational changes underlying alternative modes of membrane binding of Ras, Rheb and Arf appear to depend on fluctuations of the linker region of the HVR and the resultant changes in its interactions with lipids and the rest of the protein.Citation8,15 These interactions are modulated by the bound nucleotide through a 2-way propagation of perturbative signals across long distances. It appears that the interplay between the G-domain and membrane is affected by the propensity of specific G-domain surface patches to interact with lipid head groups () as well as helix dipoles. These may play a role in the availability of the switch regions for interaction with partner proteins, and predict that small variations in sequence and/or structure may alter function by modulating the mode of membrane binding. A recent report on Ras provides a strong support for this hypothesis.Citation65 This study found a direct link between switch III residues located on the HVR/helix 4 and nanoclustering, and thereby function.Citation65 This suggests a general functional relevance of switch III, which is conserved among the cancer-associated Ras isoforms. Another strong evidence for a direct link between function and membrane orientation is provided by a recent NMR study of K-Ras bound to a lipid nanodisc, where it was shown that some RASopathy mutations stabilize different membrane orientation states than wild type K-Ras.Citation13 Moreover, it is conceptually plausible that the population of effector-interacting membrane orientation states, where the canonical switch loops are solvent exposed, might be stabilized by binding partners. Also, oligomer formation, including dimerizationCitation62,66-68 and nanoclustering,Citation30,69-71 is likely to affect the population distribution of different orientation states. Of course, these have yet to be shown and represent an active area of investigation, as pointed out in an excellent commentary by Matthias Buck and colleagues.Citation72
Conclusion
We have summarized recent reports that documented how the G-domain of lipid-modified small GTPases, which cycles between GDP-bound inactive and GTP-bound active conformational states to control many diverse signaling pathways, is directly involved in membrane binding. This was made possible by intrinsic fluctuations and/or nucleotide dependent conformational changes leading to at least 2 distinct membrane orientations. The studies reported thus far suggest that this phenomenon is independent of the sequence or structure of the catalytic domain or the type and location of the lipid modification. We propose that the functionally important process of membrane reorientation is generalizable to all members of the Ras superfamily that attach to the plasma membrane via a lipid-modified motif. Membrane orientation can play a key functional role by modulating the availability of functionally responsive regions on the catalytic domain for interaction with binding partners. This suggests that the switching function of lipidated small G-proteins might be regulated not only by the well characterized nucleotide-dependent conformational changes but also by membrane reorientation. However, additional studies on more systems will be required to fully establish the broader pathophysiological impact of membrane reorientation.
Disclosure of potential conflicts of interest
No potential conflicts of interest were disclosed.
Funding
This work was supported by a grant from the National Institutes of Health General Medical Sciences (NIGMS grant No. R01GM100078 to AAG).
References
- van Meer G, Voelker DR, Feigenson GW. Membrane lipids: where they are and how they behave. Nat Rev Mol Cell Biol 2008; 9:112-24; PMID:18216768; https://doi.org/10.1038/nrm2330
- Moravcevic K, Oxley CL, Lemmon MA. Conditional peripheral membrane proteins: facing up to limited specificity. Structure 2012; 20:15-27; PMID:22193136; https://doi.org/10.1016/j.str.2011.11.012
- Lemmon MA, Ferguson KM, O'Brien R, Sigler PB, Schlessinger J. Specific and high-affinity binding of inositol phosphates to an isolated pleckstrin homology domain. Proc Natl Acad Sci U S A. 1995; 92:10472-6; PMID:7479822; https://doi.org/10.1073/pnas.92.23.10472
- Lemmon MA. Membrane recognition by phospholipid-binding domains. Nat Rev Mol Cell Biol 2008; 9:99-111; PMID:18216767; https://doi.org/10.1038/nrm2328
- Wennerberg K, Rossman KL, Der CJ. The Ras superfamily at a glance. J Cell Sci. 2005; 118:843-6; PMID:15731001; https://doi.org/10.1242/jcs.01660
- Abankwa D, Gorfe AA, Inder K, Hancock JF. Ras membrane orientation and nanodomain localization generate isoform diversity. Proc Natl Acad Sci U S A 2010; 107:1130-5; PMID:20080631; https://doi.org/10.1073/pnas.0903907107
- Abankwa D, Hanzal-Bayer M, Ariotti N, Plowman SJ, Gorfe AA, Parton RG, McCammon JA, Hancock JF. A novel switch region regulates H-ras membrane orientation and signal output. EMBO J 2008; 27:727-35; PMID:18273062; https://doi.org/10.1038/emboj.2008.10
- Gorfe AA, Hanzal-Bayer M, Abankwa D, Hancock JF, McCammon JA. Structure and dynamics of the full-length lipid-modified H-Ras protein in a 1,2-dimyristoylglycero-3-phosphocholine bilayer. J Med Chem 2007; 50:674-84; PMID:17263520; https://doi.org/10.1021/jm061053f
- Liu Y, Kahn RA, Prestegard JH. Dynamic structure of membrane-anchored Arf*GTP. Nat Struct Mol Biol 2010; 17:876-81; PMID:20601958; https://doi.org/10.1038/nsmb.1853
- Weise K, Kapoor S, Denter C, Nikolaus J, Opitz N, Koch S, Triola G, Herrmann A, Waldmann H, Winter R. Membrane-mediated induction and sorting of K-Ras microdomain signaling platforms. J Am Chem Soc 2011; 133:880-7; PMID:21141956; https://doi.org/10.1021/ja107532q
- Kapoor S, Triola G, Vetter IR, Erlkamp M, Waldmann H, Winter R. Revealing conformational substates of lipidated N-Ras protein by pressure modulation. Proc Natl Acad Sci U S A 2012; 109:460-5; PMID:22203965; https://doi.org/10.1073/pnas.1110553109
- Kapoor S, Weise K, Erlkamp M, Triola G, Waldmann H, Winter R. The role of G-domain orientation and nucleotide state on the Ras isoform-specific membrane interaction. Eur Biophys J 2012; 41:801-13; PMID:22851002; https://doi.org/10.1007/s00249-012-0841-5
- Mazhab-Jafari MT, Marshall CB, Smith MJ, Gasmi-Seabrook GM, Stathopulos PB, Inagaki F, Kay LE, Neel BG, Ikura M. Oncogenic and RASopathy-associated K-RAS mutations relieve membrane-dependent occlusion of the effector-binding site. Proc Natl Acad Sci U S A 2015; 112(21):6625-30; PMID:25941399; https://doi.org/10.1073/pnas.1419895112
- Mazhab-Jafari MT, Marshall CB, Stathopulos PB, Kobashigawa Y, Stambolic V, Kay LE, Inagaki F, Ikura M. Membrane-dependent modulation of the mTOR activator Rheb: NMR observations of a GTPase tethered to a lipid-bilayer nanodisc. J Am Chem Soc 2013; 135:3367-70; PMID:23409921; https://doi.org/10.1021/ja312508w
- Prakash P, Zhou Y, Liang H, Hancock JF, Gorfe AA. Oncogenic K-Ras binds to an anionic membrane in two distinct orientations: A molecular dynamics analysis. Biophys J 2016; 110:1125-38; PMID:26958889; https://doi.org/10.1016/j.bpj.2016.01.019
- Abankwa D, Gorfe AA, Hancock JF. Mechanisms of Ras membrane organization and signalling: Ras on a rocker. Cell Cycle 2008; 7:2667-73; PMID:18758236; https://doi.org/10.4161/cc.7.17.6596
- Macara IG, Lounsbury KM, Richards SA, McKiernan C, Bar-Sagi D. The Ras superfamily of GTPases. FASEB J 1996; 10:625-30; PMID:8621061
- Goitre L, Trapani E, Trabalzini L, Retta SF. The Ras superfamily of small GTPases: the unlocked secrets. Methods Mol Biol 2014; 1120:1-18; PMID:24470015; https://doi.org/10.1007/978-1-62703-791-4_1
- Rojas AM, Fuentes G, Rausell A, Valencia A. The Ras protein superfamily: evolutionary tree and role of conserved amino acids. J Cell Biol 2012; 196:189-201; PMID:22270915; https://doi.org/10.1083/jcb.201103008
- Cox AD, Der CJ. Ras history: The saga continues. Small GTPases 2010; 1:2-27; PMID:21686117; https://doi.org/10.4161/sgtp.1.1.12178
- Clarke PR, Zhang C. Ran GTPase: a master regulator of nuclear structure and function during the eukaryotic cell division cycle? Trends Cell Biol 2001; 11:366-71; PMID:11514190; https://doi.org/10.1016/S0962-8924(01)02071-2
- Wennerberg K, Der CJ. Rho-family GTPases: it's not only Rac and Rho (and I like it). J Cell Sci 2004; 117:1301-12; PMID:15020670; https://doi.org/10.1242/jcs.01118
- Donaldson JG, Jackson CL. ARF family G proteins and their regulators: roles in membrane transport, development and disease. Nat Rev Mol Cell Biol 2011; 12:362-75; PMID:21587297; https://doi.org/10.1038/nrm3117
- Li G, Marlin MC. Rab family of GTPases. Methods Mol Biol 2015; 1298:1-15; PMID:25800828; https://doi.org/10.1007/978-1-4939-2569-8_1
- Diekmann Y, Seixas E, Gouw M, Tavares-Cadete F, Seabra MC, Pereira-Leal JB. Thousands of rab GTPases for the cell biologist. PLoS Comput Biol 2011; 7:e1002217; PMID:22022256; https://doi.org/10.1371/journal.pcbi.1002217
- Klopper TH, Kienle N, Fasshauer D, Munro S. Untangling the evolution of Rab G proteins: implications of a comprehensive genomic analysis. BMC Biol 2012; 10:71; PMID:22873208; https://doi.org/10.1186/1741-7007-10-71
- Chavrier P, Gorvel JP, Stelzer E, Simons K, Gruenberg J, Zerial M. Hypervariable C-terminal domain of rab proteins acts as a targeting signal. Nature 1991; 353:769-72; PMID:1944536; https://doi.org/10.1038/353769a0
- Hancock JF, Magee AI, Childs JE, Marshall CJ. All ras proteins are polyisoprenylated but only some are palmitoylated. Cell 1989; 57:1167-77; PMID:2661017; https://doi.org/10.1016/0092-8674(89)90054-8
- Wittinghofer A, Vetter IR. Structure-function relationships of the G domain, a canonical switch motif. Annu Rev Biochem 2011; 80:943-71; PMID:21675921; https://doi.org/10.1146/annurev-biochem-062708-134043
- Hancock JF. Ras proteins: different signals from different locations. Nat Rev Mol Cell Biol 2003; 4:373-84; PMID:12728271; https://doi.org/10.1038/nrm1105
- Prior IA, Hancock JF. Compartmentalization of Ras proteins. J Cell Sci 2001; 114:1603-8; PMID:11309191
- Prior IA, Harding A, Yan J, Sluimer J, Parton RG, Hancock JF. GTP-dependent segregation of H-ras from lipid rafts is required for biological activity. Nat Cell Biol 2001; 3:368-75; PMID:11283610; https://doi.org/10.1038/35070050
- Weise K, Triola G, Brunsveld L, Waldmann H, Winter R. Influence of the lipidation motif on the partitioning and association of N-Ras in model membrane subdomains. J Am Chem Soc 2009; 131:1557-64; PMID:19133719; https://doi.org/10.1021/ja808691r
- Nishimoto T. Upstream and downstream of ran GTPase. Biol Chem 2000; 381:397-405; PMID:10937870; https://doi.org/10.1515/BC.2000.052
- Vetter IR, Wittinghofer A. The Guanine Nucleotide-Binding Switch in Three Dimensions. Science 2001; 294:1299-304; PMID:11701921; https://doi.org/10.1126/science.1062023
- Bos JL, Rehmann H, Wittinghofer A. GEFs and GAPs: critical elements in the control of small G proteins. Cell 2007; 129:865-77; PMID:17540168; https://doi.org/10.1016/j.cell.2007.05.018
- Vigil D, Cherfils J, Rossman KL, Der CJ. Ras superfamily GEFs and GAPs: validated and tractable targets for cancer therapy? Nat Rev Cancer 2010; 10:842-57; PMID:21102635; https://doi.org/10.1038/nrc2960
- Karnoub AE, Weinberg RA. Ras oncogenes: split personalities. Nat Rev Mol Cell Biol 2008; 9:517-31; PMID:18568040; https://doi.org/10.1038/nrm2438
- Vasiliev JM, Omelchenko T, Gelfand IM, Feder HH, Bonder EM. Rho overexpression leads to mitosis-associated detachment of cells from epithelial sheets: a link to the mechanism of cancer dissemination. Proc Natl Acad Sci U S A 2004; 101:12526-30; PMID:15304643; https://doi.org/10.1073/pnas.0404723101
- Seixas E, Barros M, Seabra MC, Barral DC. Rab and Arf proteins in genetic diseases. Traffic 2013; 14:871-85; PMID:23565987; https://doi.org/10.1111/tra.12072
- Saxena S, Gandhi A, Lim PW, Relles D, Sarosiek K, Kang C, Chipitsyna G, Sendecki J, Yeo CJ, Arafat HA. RAN GTPase and Osteopontin in Pancreatic Cancer. Pancreat Disord Ther 2013; 3:113; PMID:24749004; https://doi.org/10.4172/2165-7092.1000113
- Schubbert S, Shannon K, Bollag G. Hyperactive Ras in developmental disorders and cancer. Nat Rev Cancer 2007; 7:295-308; PMID:17384584; https://doi.org/10.1038/nrc2109
- Vega FM, Ridley AJ. Rho GTPases in cancer cell biology. FEBS Lett 2008; 582:2093-101; PMID:18460342; https://doi.org/10.1016/j.febslet.2008.04.039
- Zeitouni D, Pylayeva-Gupta Y, Der JC, Bryant LK. KRAS Mutant Pancreatic Cancer: No Lone Path to an Effective Treatment. Cancers 2016; 8:E45; PMID:27096871; https://doi.org/10.3390/cancers8040045
- Cox AD, Fesik SW, Kimmelman AC, Luo J, Der CJ. Drugging the undruggable RAS: Mission Possible? Nat Rev Drug Discov 2014; 13:828-51; PMID:25323927; https://doi.org/10.1038/nrd4389
- Doherty KJ, McKay C, Chan KK, El-Tanani MK. RAN GTPase as a target for cancer therapy: Ran binding proteins. Curr Mol Med 2011; 11:686-95; PMID:21902650; https://doi.org/10.2174/156652411797536688
- Spiegel J, Cromm PM, Zimmermann G, Grossmann TN, Waldmann H. Small-molecule modulation of Ras signaling. Nat Chem Biol 2014; 10:613-22; PMID:24929527; https://doi.org/10.1038/nchembio.1560
- Wang W, Fang G, Rudolph J. Ras inhibition via direct Ras binding—is there a path forward?. Bioorg Med Chem Lett 2012; 22:5766-76; PMID:22902659; https://doi.org/10.1016/j.bmcl.2012.07.082
- Konstantinopoulos PA, Karamouzis MV, Papavassiliou AG. Post-translational modifications and regulation of the RAS superfamily of GTPases as anticancer targets. Nat Rev Drug Discov 2007; 6:541-55; PMID:17585331; https://doi.org/10.1038/nrd2221
- Nguyen UT, Goodall A, Alexandrov K, Abankwa D. Isoprenoid modifications. In Post- translational modifications in health and disease. (Vidal CJ ed.), 1st Ed., Springer. pp 486.
- Williams CL. The polybasic region of Ras and Rho family small GTPases: a regulator of protein interactions and membrane association and a site of nuclear localization signal sequences. Cell Signal 2003; 15:1071-80; PMID:14575862; https://doi.org/10.1016/S0898-6568(03)00098-6
- Spoerner M, Herrmann C, Vetter IR, Kalbitzer HR, Wittinghofer A. Dynamic properties of the Ras switch I region and its importance for binding to effectors. Proc Natl Acad Sci U S A 2001; 98:4944-9; PMID:11320243; https://doi.org/10.1073/pnas.081441398
- Gorfe AA, Grant BJ, McCammon JA. Mapping the nucleotide and isoform-dependent structural and dynamical features of Ras proteins. Structure 2008; 16:885-96; PMID:18547521; https://doi.org/10.1016/j.str.2008.03.009
- Grant BJ, McCammon JA, Gorfe AA. Conformational selection in G-proteins: lessons from Ras and Rho. Biophys J 2010; 99:L87-9; PMID:21112273; https://doi.org/10.1016/j.bpj.2010.10.020
- Raimondi F, Orozco M, Fanelli F. Deciphering the deformation modes associated with function retention and specialization in members of the Ras superfamily. Structure 2010; 18:402-14; PMID:20223222; https://doi.org/10.1016/j.str.2009.12.015
- Buhrman G, Holzapfel G, Fetics S, Mattos C. Allosteric modulation of Ras positions Q61 for a direct role in catalysis. Proc Natl Acad Sci U S A 2010; 107:4931-6; PMID:20194776; https://doi.org/10.1073/pnas.0912226107
- Grecco HE, Schmick M, Bastiaens P. Signaling from the living plasma membrane. Cell 2011; 6:897-909; https://doi.org/10.1016/j.cell.2011.01.029
- Vartak N, Bastiaens P. Spatial cycles in G-protein crowd control. Embo J 2010; 29:2689-99; PMID:20717139; https://doi.org/10.1038/emboj.2010.184
- Iversen L, Tu HL, Lin WC, Christensen SM, Abel SM, Iwig J, Wu HJ, Gureasko J, Rhodes C, Petit RS, et al. Molecular kinetics. Ras activation by SOS: allosteric regulation by altered fluctuation dynamics. Science 2014; 345:50-4; PMID:24994643; https://doi.org/10.1126/science.1250373
- Groves JT, Kuriyan J. Molecular mechanisms in signal transduction at the membrane. Nat Struct Mol Biol 2010; 17:659-65; PMID:20495561; https://doi.org/10.1038/nsmb.1844
- Blazevits O, Mideksa YG, Solman M, Liqabue A, Ariotti N, Nakhaeizadeh H, et al. Galectin-1 dimers can scaffold Raf-effectors to increase H-Ras nanoclustering. Sci Rep 2016; 6:24165; PMID:27087647; https://doi.org/10.1038/srep24165
- Guldenhaupt J, Rudack T, Bachler P, Mann D, Triola G, Waldmann H, Kötting C, Gerwert K. N-Ras forms dimers at POPC membranes. Biophys J 2012; 103:1585-93; PMID:23062351; https://doi.org/10.1016/j.bpj.2012.08.043
- Hancock JF, Paterson H, Fau-Marshall CJ, Marshall CJ. A polybasic domain or palmitoylation is required in addition to the CAAX motif to localize p21ras to the plasma membrane. Cell 1990; 63:133-9; PMID:2208277; https://doi.org/10.1016/0092-8674(90)90294-O
- Lukman S, Grant BJ, Gorfe AA, Grant GH, McCammon JA. The distinct conformational dynamics of K-Ras and H-Ras A59G. PLoS Comput Biol 2010; 6:e1000922; PMID:20838576; https://doi.org/10.1371/journal.pcbi.1000922
- Solman M, Liqabue A, Blazevits O, Jaiswal A, Zhou Y, Liang H, Lectez B, Kopra K, Guzmán C, Härmä H, et al. Specific cancer-associated mutations in the switch-III region of Ras increase tumorigenicity by nanocluster augmentation. Elife 2015; 4:e08905; PMID:26274561; https://doi.org/10.7554/eLife.08905
- Muratcioglu S, Chavan TS, Freed BC, Jang H, Khavrutskii L, Freed RN, Dyba MA, Stefanisko K, Tarasov SG, Gursoy A, et al. GTP-dependent K-Ras dimerization. Structure 2015; 23:1325-35; PMID:26051715; https://doi.org/10.1016/j.str.2015.04.019
- Nan X, Tamguney TM, Collisson EA, Lin LJ, Pitt C, Galeas J, Lewis S, Gray JW, McCormick F, Chu S. Ras-GTP dimers activate the mitogen-activated protein kinase (MAPK) pathway. Proc Natl Acad Sci U S A 2015; 112:7996-8001; PMID:26080442; https://doi.org/10.1073/pnas.1509123112
- Sayyed-Ahmad A, Cho KJ, Hancock JF, Gorfe AA. Computational equilibrium thermodynamic and kinetic analysis of K-Ras dimerization through an effector binding surface suggests limited functional role. J Phys Chem B 2016; PMID:27072779
- Cho KJ, Hancock JF. Ras nanoclusters: a new drug target. Small GTPases 2013; 4:57-60; PMID:23419283; https://doi.org/10.4161/sgtp.23145
- Zhou Y, Hancock JF. Ras nanoclusters: Versatile lipid-based signaling platforms. Biochim Biophys Acta 2015; 1853:841-9; PMID:25234412; https://doi.org/10.1016/j.bbamcr.2014.09.008
- Abankwa D, Gorfe AA, Hancock JF. Ras nanoclusters: molecular structure and assembly. Semin Cell Dev Biol 2007; 18:599-607; PMID:17897845; https://doi.org/10.1016/j.semcdb.2007.08.003
- Li Z, Cao S, Buck M. K-Ras at anionic membranes: Orienation, orientation…orientation. Recent simulations and experiments. Biophys J 2016; 110:1033-5; PMID:26958879; https://doi.org/10.1016/j.bpj.2016.01.020