ABSTRACT
Rab molecular switches are key players in defining membrane identity and regulating intracellular trafficking events in eukaryotic cells. In spite of their global structural similarity, Rab-family members acquired particular features that allow them to perform specific cellular functions. The overall fold and local sequence conservations enable them to utilize a common machinery for prenylation and recycling; while individual Rab structural differences determine interactions with specific partners such as GEFs, GAPs and effector proteins. These interactions orchestrate the spatiotemporal regulation of Rab localization and their turning ON and OFF, leading to tightly controlled Rab-specific functionalities such as membrane composition modifications, recruitment of molecular motors for intracellular trafficking, or recruitment of scaffold proteins that mediate interactions with downstream partners, as well as actin cytoskeleton regulation.
In this review we summarize structural information on Rab GTPases and their complexes with protein partners in the context of partner binding specificity and functional outcomes of their interactions in the cell.
The Rab GTPases family
The hallmark of eukaryotic cells is their wealth of intracellular compartments bound by membranes. Trafficking between these cellular compartments is complex and involves selective sorting of cargos, formation and transport of vesicles, and specific fusion with the target membranes at the final destination. Rabs (Ras-related protein in brain)Citation1 are essential regulators of intracellular trafficking. They mediate specific exchange of proteins and lipids between compartments, thus define compartment identity. Rabs carry out these processes by localizing to specific intracellular membranes and recruiting a diverse set of Rab effectors (e.g. tethering factors, molecular motors, phospholipid modulators, etc.) to control vesicular trafficking events.Citation2,3
Rabs are ancient and diverse, and the number of Rabs varies widely among organisms across phylogeny. In humans, there are 70 Rabs that belong to 44 subfamilies (), representing the largest small GTPase family and reflecting the complexity of membrane transport events in which they participate.Citation4 Rab functional subgroups with shared ancestry often participate in related but non-overlapping cellular activities.Citation5 Five Rabs (Rab1, Rab5, Rab6, Rab7 and Rab11) are found in all eukaryotic genomes and thus seem indispensable.Citation6
Figure 1. Sequence alignment of human Rab G-domains. Conserved nucleotide binding motifs are highlighted: PM1-PM3 - phosphate, magnesium binding motifs; G1-G3 - guanine moiety binding motifs. Rab family specific motifs (RabF1–5), Rab subfamily specific motifs (RabSF1–4) as well as C-terminal interacting motif (CIM) are highlighted. C-terminal cysteines (red) within geranylgeranylation motifs (GGM) are found in one of the following combinations: XXXCC, XXCCX, XCCXX, CCXXX, XXCXC and XCXXX. Hydrophobic triad residues (see ) are marked in yellow. Secondary structure elements corresponding to the Rab3 structure (3RAB) are shown on the top, as well as Switch-1, Interswitch, Switch-2, complementarity-determining regions (CDR1–5) and the hyper-variable C-terminal domain (HVD). Rab sequences are presented in an order of proximity in the phylogenetic tree of human Rabs.Citation4 The G-domains of closely related Rabs (Rab26 and Rab37) share 76% sequence identity while the more diverse family members (Rab1A and Rab20) exhibit as low as 16% G-domain identity. Among the human Rabs the protein lengths vary between 194 (Rab22a) to 740 residues (RasEF45 or Rab45). Residue conservation color code: red -negatively charged, blue - positively charged, polar - magenta, hydrophobic - green, prolines and glycines -brown. The protein sequences Uniprot database accession numbers: RAB23 Q9ULC3; RAB29 O14966; RAB38 P57729; RAB32 Q13637; RAB9A P51151; RAB7A P51149; RAB28 P51157; RAB20 Q9NX57; RAB34 Q9BZG1; RAB36 O95755; RAB22A Q9UL26; RAB5A P20339; RAB17 Q9H0T7; RAB21 Q9UL25; RAB24 Q969Q5; RAB41 Q5JT25; RAB6A P20340; RAB30 Q15771; RAB33A Q14088; RAB43 Q86YS6; RAB19 A4D1S5; RAB25 P57735; RAB11A P62491; RAB42 NP_001180461.1; RAB39A Q14964; RAB2A P61019; RAB14 P61106; RAB4A P20338; RASEF45 Q8IZ41; RAB44 Q7Z6P3; RAB12 Q6IQ22; RAB18 Q9NP72; RAB3A P20336; RAB27A P51159; RAB26 Q9ULW5; RAB37 Q96AX2; RAB10 P61026; RAB13 P51153; RAB8A P61006; RAB35 Q15286; RAB1A P62820; RAB15 P59190; RAB40A Q8WXH6.
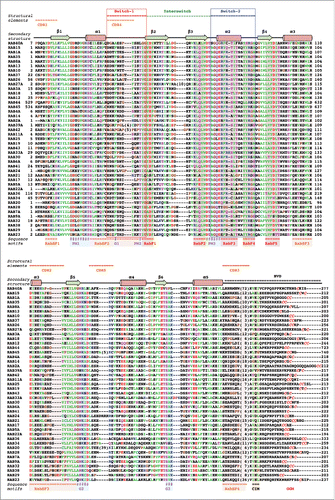
Rabs work as typical Ras-like small GTPases. They are nucleotide dependent molecular switches that are ON in the GTP-bound (active) form and OFF in the GDP-bound (inactive) form. All Rabs share a conserved fold (G-domain) compatible with strong binding of Mg2+GDP and Mg2+GTP and with GTP hydrolysis.Citation7 In spite of considerable structural and biochemical similarities, Rabs play multiple and divergent roles in many fundamental cellular processes.Citation8 Over the past 20 years, significant progress has been made in understanding how the diversity of Rab proteins controls the trafficking and exchange between cellular compartments at the molecular level. Studies have revealed how Rab proteins are regulated and how they coordinate trafficking events in cooperation with their binding partners. Interestingly, some intracellular bacterial pathogens subvert Rabs to evade degradation. They introduce virulence factors into the host cell cytoplasm that interact with Rabs to re-direct membrane trafficking pathways and protect the newly formed bacteria-containing vacuole from destruction by the host cell.Citation9 In this review, we summarize the available structural information for Rabs (Table S1) and illustrate their overall structural conservation as well as their structural and functional distinctions that allow them to achieve their diverse yet specific functions in intracellular trafficking. Selected examples will highlight how coordination between Rab and partners and Rab cross-talk maintain cellular compartment identity and regulate membrane trafficking in eukaryotic cells. They also highlight how disruption of Rab function can lead to disease and how Rab function can be hijacked by pathogens.
Basis of a conserved switch mechanism: Nucleotide-dependence of the Rab G-domain structure and dynamics
The nucleotide-dependent conformational change that each Rab undergoes is at the heart of its functionality as a switch. All Rabs contain a conserved globular G-domain, consisting of about 180 residues, that is related to all other Ras-superfamily membersCitation10 (). Conserved fingerprint sequence motifs surround the nucleotide-binding site: 3 phosphate/magnesium-binding motifs (PM1-PM3) conserved in P-loop NTPases and 3 guanine-binding motifs (G1-G3) that recognize specifically the purine base ( and ). Differences between structures of GTP- and GDP- bound G-domains suggest a “loaded-spring” conformational switch mechanism,Citation11 as first observed for Ras structures.Citation12 The GTP gamma-phosphate mediates constraining interactions between PM motifs, thereby stabilizing 2 protein regions, Switch1 and Switch2, and keeping the protein in an active “ON” state (). Nucleotide hydrolysis and Pi release destabilize the Switches, relaxing them into the GDP-bound “OFF” conformation. As a result, these 2 states exhibit pronounced structural differences (), allowing selective recognition of Rabs by regulatory proteins and effectors in a nucleotide-dependent manner.
Figure 2. Structures of Rab GTPase and the diversity of their conformational switch. (A) Crystal structures of yeast Rab Sec4p in its GTP- and GDP-bound forms (1G17 and 1G16), the first Rab structures determined in both nucleotide bound states,Citation149 are shown. Conserved PM and G motifs are highlighted in cyan. Switch1, Switch2 and Interswitch (sequence between the 2 Switches) regions are shown in red, blue, and green, respectively. The nucleotides and magnesium ions are shown as dark-gray sticks and spheres, respectively. The color scheme is used throughout all figures. (B) Diverse nucleotide-dependent conformational changes in Rabs. Ypt32Citation13 and Rab28Citation14 structures in GTP- and GDP-bound states are shown. (C) Comparison of the conformations of GTP-bound Rabs and GDP-bound Rabs. Left: superimposition of GTP-bound structures of Rab1b (3NKV), Rab2 (4RKE), Rab3a (3RAB), Rab4a (2BME), Rab5c (1HUQ), Rab6a (1YZQ), Rab7a (1T91), Rab8a (4LHW), Rab9a (1YZL), Rab11a (1OIW), Rab18(1×3S), Rab21 (1YZT), Rab22a (1YVD), Rab26 (2G6B), Rab28 (3E5H), Rab30 (2EW1), Rab33b (1Z06), Sec4p (1G17), Ypt1 (1YZN), Ypt32 (3RWM), Ypt51 (1EK0), and Ypt7 (1KY2). Right: superimposition of GDP-bound structures of Rab1a (2FOL), Rab2a (1Z0A), Rab3d (2GF9), Rab4a (2O52), Rab5a (1TU4), Rab6b (2E9S), Rab7a (1VG1), Rab8a (4LHV), Rab9a (1S8F), Rab11a (1OIV), Rab12 (2IL1), Rab14 (1Z0F), Rab21 (1Z0I), Rab23 (1Z22), Rab25 (2OIL), Rab28 (2HXS), Rab45 (2P5S), Sec 4 (1G16), Ypt32 (3RWM), and Ypt7 (1KY3).
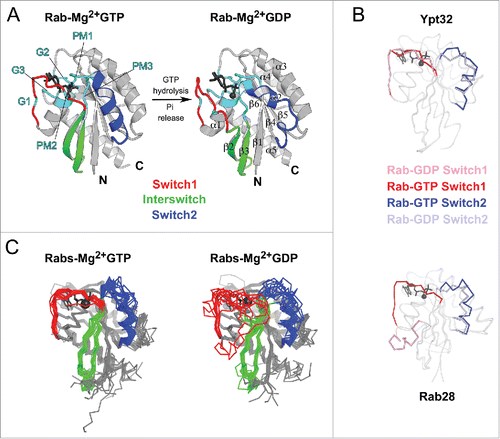
The degree of the nucleotide-dependent conformational change that a Rab undergoes during the ON to OFF transition varies, as revealed by crystal structures of different Rabs (). For example, the structures of GTP- and GDP-bound yeast Rab Ypt32 show local rearrangement of Switch1 and Switch2 regions.Citation13 In contrast, Rab28 undergoes dramatic conformational changes upon GTP hydrolysis, with Switch1 folded as a helix and displaced by 25 Å in the GDP-bound structure.Citation14 Although crystal structures often capture a single conformation of the Switches and may artificially stabilize particular conformations, they nonetheless show that large flexibility and variety of conformational changes can occur among different Rabs when switching between their ON and OFF states.
Overall, various Rab GDP-bound structures have demonstrated a high degree of flexibility and disorder for the Switch1 and Switch2 regions, allowing them to explore a wide array of conformations (). In contrast, Rab GTP-bound structures show that the Switch regions are stabilized by GTP binding, resulting in restricted conformational flexibility compared with the inactive form (). These differences in structures as well as the conformational compliance of the GDP- and GTP-bound forms of Rab are key to their ability to specifically recognize their cellular partners. Despite their overall structural similarity, critical sequence and local structural differences among the active form of Rabs enable them to act as key determinants of membrane identity.
The regulation of switching ON and OFF of Rab activity is essential for their function. The active form of Rabs acts as a timer of specific activity on the membrane and thus controls how long their effectors can stay bound for defined functionalities. Rab's catalytic site is adapted so that it binds both GDP and GTP strongly. Thus, there is no significant spontaneous exchange of nucleotide, which excludes Rab self-activation and explains the requirement of GEF (guanine nucleotide exchange factor) to stimulate Rab nucleotide exchange and to control Rab activation in cells. The intrinsic GTP hydrolysis rates of Rabs that would lead to switching off of their activity are also slow, although they vary significantly among Rab GTPasesCitation15 in spite of a high degree of sequence conservation of the nucleotide binding pocket. Interestingly, the timing of biological processes regulated by Rabs appears to correlate with their intrinsic GTP hydrolysis rates. The low intrinsic Rab6 GTPase activity can ensure its role in regulating long-range vesicular transport.Citation16 In contrast, the fast GTPase Rab5 regulates rapid kiss-and-run membrane fusion events.Citation17 The intrinsic GTPase rate may control the lifetime of Rab's association with their effectors; while the binding of some effectors slows down Rab GTP hydrolysis rates in vitro.Citation18,19 The importance of Rab intrinsic GTP hydrolysis in cellular processes has not yet been tested in vivo. Stimulation of Rab GTPase activity by GAPs (GTPase activating proteins) timely switches off Rabs and subsequently the activities they coordinate. In addition to the timely control of their activity, the spatial regulation of Rab recruitment and deactivation is central to their ability to control functionalities on specific cellular compartments.
Membrane targeting and spatiotemporal control of Rab functions require specific partners
Cellular partners are essential to control the precise localization of Rabs on diverse membranes and to temporally and spatially control their activities in a tightly regulated manner (). The majority of Rab partners associate with each Rab in a nucleotide-dependent manner and regulate the Rab functional cycle (). The functional diversity of Rabs as well as their overall conservation raises the question of how these partners can selectively interact with and define the proper subcellular localization, the precise spatial and temporal cycling between GDP/GTP nucleotide bound forms as well as the nucleotide-dependent specific recruitment of effectors for each Rab.
Figure 3. Rab functional cycle. The newly synthesized GDP-bound Rab is recognized by REP and presented to RabGGT, which geranylgeranylates the Rab on one or two C-terminal Cys residues. Targeting of the Rab/REP (or Rab/GDI) complex to specific membranes is mediated by the interaction with a membrane-associated GEF, which stimulates GDP to GTP exchange of Rab; or in few cases by interaction with a GDF first, followed by GEF stimulated activation. The GTP-bound (active) Rab conformation is recognized by multiple effector proteins, which then carry out their specific functions. GAP stimulates Rab GTPase activity and converts Rab back to the inactive, GDP-bound form, resulting in the dissociation of effectors. GDI regulates the cycling of Rab between the membranes by extracting inactive Rab from a membrane into the cytosol and subsequently delivering it back to a donor membrane compartment.
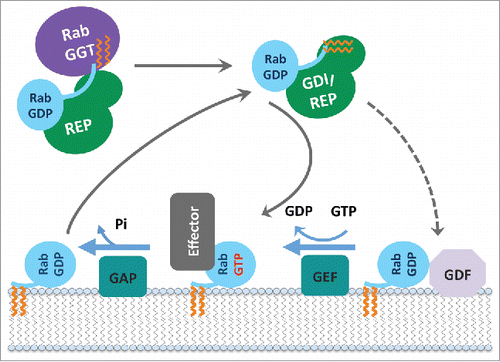
All newly synthesized Rabs (preferentially in their GDP-bound forms) are recognized by REP (Rab escort protein) and presented to RabGGT (Rab geranylgeranyl transferase), which geranylgeranylates the Rab on one or two C-terminal Cys residues. The prenylated Rab can diffuse as a cytosolic complex with universal Rab chaperones, namely REP and GDI (GDP dissociation inhibitor), an evolutionarily conserved REP paralog. Specific targeting of each prenylated Rab-GDP to a particular membrane is then achieved when the cytosolic complex dissociates and Rab is incorporated into this membrane. The mechanisms of the specific membrane targeting are not yet fully understood. It has been proposed to occur via specific GDF (GDI displacement factor) that promotes dissociation of the prenylated Rab from GDI and facilitates its membrane incorporation.Citation20 However, there is more evidence for the role of specific membrane-localized GEFs in Rab membrane targeting,Citation21–23 suggesting that GEF facilitates Rab recruitment from the cytosol via stimulation of the GDP-to-GTP exchange. The C-terminal hyper-variable and intrinsically disordered region of Rab also contributes to membrane delivery specificity of some Rabs,Citation20,24,25 implicating it in binding to membrane markers (including effectors and phospholipids).Citation25 Once on the membrane, the GTP-bound (active) Rab conformation is recognized by multiple effector proteins, which then convey specific functionalities. A GAP then converts Rab back to its inactive GDP-bound form that can no longer recruit effectors, therefore switching off these Rab-specific functionalities on this membrane. GDI enables the extraction of inactive Rab from the membrane and its diffusion back to membrane compartments for another round of activation ().
Most partners that interact with Rab GTPases share a critical Rab surface for their association (). Available structural and biochemical binding studies show that the Switch1, Switch2 and Interswitch (sequence between the 2 Switches) regions of Rab form interactions at the interface with almost all partners. These regions overlap with 4 of 5 conserved Rab-family motifs (RabF1-RabF4)Citation26 () and together, they constitute the surface of the Rab molecule that is the most conserved in sequence but also the most distinct between the GDP and GTP forms. A conserved hydrophobic triad of aromatic residues within this conserved surface () plays an important role in determining Rab-partner binding specificity, with their side chain orientations being influenced by surrounding variable residues.Citation27 Four other motifs conserved only within Rab subfamilies, RabSF1–4 (),Citation26 also cluster around the Switch-Interswitch regions. RabSF1, 3, and 4 overlap with Rab complementarity-determining regions (CDR) 1, 2, and 3,Citation28 respectively. CDRs have been defined based on their contribution to the selective recruitment of effectors by individual Rabs and are variable among Rab subfamilies. The beginning of Switch1, which overlaps with RabSF2, is also important for recognition of some partnersCitation29,30 and can be named CDR4. The conservation of this critical Rab interaction surface is shown in . The central part of the surface is the most conserved while the variable CDRs are found at the periphery.
Figure 4. Rab partner recognition sites. Rab (Rab3a, 3RAB) Mg2+-GTP (dark gray) bound structure with the structural elements contributing to partner binding highlighted. (A) Side view showing the Rab canonical effector binding site composed of Switch1, Interswitch, and Switch2; surrounded by complementarity determining regions (CDR1–5, shown in orange). Conserved Rab hydrophobic triad is shown in yellow sticks. The surface representation is shown in the middle, and conservation of surface residues (based on the sequence alignment in ) generated using ConSurf web server Citation150 is presented on the right. (B) Top view showing a new, secondary Rab effector binding site composed of the CDR4 and CDR5 regions flanking the nucleotide binding pocket.
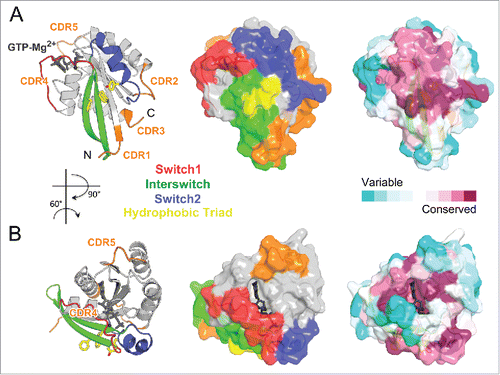
A second effector-binding site adjacent to the main partner-binding surface has been identified recently for 2 Rab11 effectors.Citation31,32 This second site comprises the guanine group of the nucleotide, CDR4, as well as the β5-α4 loop, which differs in Rab sequences and can be named CDR5 ( and ). This non-conserved binding site provides high specificity in Rab effector recognition. It is currently unknown whether this site could also serve for recognition of effectors in other Rab family members.
Key determinants in Rabs for specific partner recognition
Considering the multitude and diversity of the Rab family,Citation2,8,33 it is remarkable that the same critical surface of the G-domain has evolved so that the requirement for sharing common partners such as REP and GDI have been maintained, while sufficient differences in their sequences would allow specific recognition of various effectors and regulators (GEF and GAP). The binding specificity acquired by this surface during evolution is thus of particular interest given its rather important sequence conservation in the Rab family and the large number of partners that must interact with it for a particular Rab. We will present how this conserved interacting surface can be fine-tuned to specifically bind to each of these partners in light of the Rab/partner structures currently available.
Universal recognition of GDP-bound Rabs by REP and GDI: Common machinery to anchor a Rab to the membrane
Rab prenylation is essential for membrane anchoring and uses a shared machinery. RabGGT appears to be required for the geranylgeranylation of C-terminal cysteines of all Rabs.Citation34 Interestingly however, the sequence of the Rab prenylation motifs in the intrinsically disordered C-terminal tail of Rab proteins is not conserved (). Moreover, the RabGGT cannot recognize the Rab C-terminal peptide as a substrate, it requires an accessory adaptor protein, REP, to do so.Citation35,36 Insights into the mechanism of the Rab prenylation machinery assembly and its ability to recognize all Rabs have been provided by crystal structures of Rab7 bound to REP1 isoformCitation37 and REP1 bound to RabGGTCitation38 as well as the model of the tripartite complexCitation39 (). The REP (domain I) preferably binds to GDP-bound Rabs via RabF1-RabF4 motifs present in the Switch-Interswitch region and also interacts with the C-terminal interacting motif (CIM) of the hypervariable tail domain (HVD)Citation38,39 ( and , ). REP domain II directly interacts with the α subunit of the RabGGT and, in doing so, REP orients the Rab C-terminal tail toward the active site of the RabGGT, allowing prenylation of a range of cysteine-containing motifs. The distance between the CIM and the RabGGT catalytic site is consistent with the experimentally defined minimal linker length (9 residues) required for efficient prenylation.Citation39 After prenylation, the modified Rab C-terminus binds to a pocket in the REP domain II, and REP chaperones the prenylated Rab to the membrane.Citation37
Figure 5. Universal Rab partners support Rab prenylation and recycling. (A) A model of Rab prenylation ternary complex based on Rab7a/REP1 (1VG0) and REP1/RabGGT (1LTX) complex structures. Rab7a, REP1 (composed of 2 domains D-I and D-II), and RabGGT are shown in light blue, green, and violet; respectively. Prenyl moiety bound to RabGGT active site is shown in yellow sticks. (B) (Left) Rab7a residues involved in REP1 binding are shown as spheres and colored by conservation. (Right) Rab27b-GDP forms a dimer by swapping Switch1-Interswitch-Switch2 regions between the monomers in the crystal.Citation41 Small angle X-ray scattering (SAXS) data demonstrate, however, that Rab27b-GDP is monomeric in solution and adopts an atypical extended structure.Citation41 (C) Structure of prenylated Ypt1p (light blue), in complex with yeast RabGDI (green). The prenyl moiety bound to RabGDI D-II is shown in yellow sticks (1UKV).
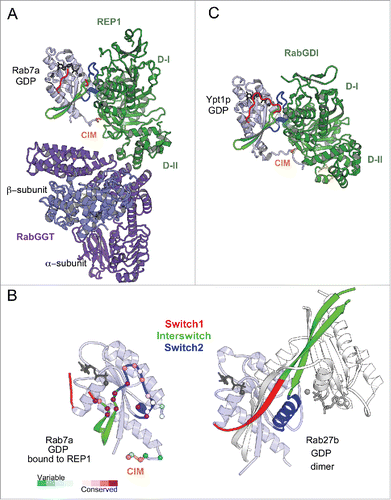
Significant differences in the Switch1 and Switch2 conformations among distinct GDP-bound Rabs (), in addition to the sequence variations in REP binding sites, may explain variable binding affinities of REP1 to different Rabs.Citation37 The competition between intracellular Rabs for binding to the prenylation complex may contribute to under-prenylation of some Rabs, including melanosomal Rab27 and Rab38.Citation40 Rab27b-GDP crystal structure demonstrates an extensive rearrangement of the Switch1-Interswitch-Switch2 region that participates in intermolecular interactions favoring a dimeric form in the crystalCitation41 (). The structural particularity of the Switch-Interswitch region observed for Rab27bCitation41 and the missing CIM in the tail of Rab27a correlates with its low affinity to REPCitation37,40 and likely poses a disadvantage for its prenylation compared with others. The under-prenylation of Rab27a reported in the choroideremia disease in human results from loss-of-function mutations in REP1 isoform.Citation42
RabGDI, a REP paralog, shares with REP the ability to chaperone prenylated Rabs in cytosol. Consistent with this functional similarity, RabGDI is structurally related to REP and binds Rabs using a similar binding mode () involving conserved residues of the G-domain canonical partner binding surface and CIM.Citation43 It is, however, unable to support Rab prenylation.Citation44 In contrast to REP, which binds Rab with similar affinity independently of its prenylation status, RabGDI binds geranylgeranylated Rabs with higher affinity, enabling efficient extraction of Rabs from membranesCitation45,46 and allowing it to play a role in Rab recycling.Citation44
In summary, the conservation of the residues on the critical most conserved Rab surface enables Rabs to share common REP/GDI proteins. However, the variability of the tail, few substitutions in the RabF motifs and differences in the plasticity of this Rab surface are important distinctive features among Rabs that can lead to different binding affinities for their universal partners.
Specific recognition of Rab proteins by GEFs
The key common characteristic of Rabs is their very slow intrinsic nucleotide exchange rate, which allows for the spatial control of the GDP/GTP exchange in cells by specific membrane localized GEFs. The Rab-GEF interactions characterized so far are specific and diverse. Rab GEFs that facilitate nucleotide exchange can belong to different folds (monomeric VPS9,Citation47 DENN,Citation48 bacterial DrrACitation49 and MSS4 that is suggested to function as a nucleotide-free Rab chaperon;Citation50 homodimeric Sec2;Citation30,51 heterodimeric Mon1–Ccz1 complexCitation52 and oligomeric TRAPPI complexCitation53) () and the mechanisms promoting nucleotide exchange are also not conserved among them.Citation46,54,55 The currently available Rab/GEF structures have, however, provided insights into the common general principles used by GEFs. GEF-stimulated nucleotide exchange is driven by allosteric competition between Mg2+-nucleotide and GEF: the initial GEF interactions promote Rab conformational changes to open the nucleotide binding pocket and ultimately establish a tight interface with a nucleotide-free Rab. Owing to the cellular excess of GTP over GDP, subsequent Mg2+-GTP binding converts the Rab conformation back to the active form, which dissociates the Rab/GEF complex and results in a nucleotide-exchanged, activated Rab.
Figure 6. Rab nucleotide exchange factors of different structural folds. Left: Conformational changes in Rabs upon interactions with cognate GEFs. Rab residues contacting GEF (within 4 Å distance) are shown as spheres. PM-G motif residues are cyan, CDR residues are orange. Rab8a-GTP structure (4LHW) is shown as a reference for the Switch1, Switch2 conformations in a GTP-bound Rab (top). Right: Structures of Rab/RabGEF complexes: Rab21/Rabex5-HB-VPS9-domain (2OT3), Rab35/DENND1B-DENN-domain (3TW8), Rab1a/DrrA-GEF-domain (2WWX), Rab8a/Rabin8-GEF-domain (4LHX), Ypt7p/Mon1-Ccz1-complex-core (5LDD), Ypt1p/TRAPPI-complex-core (3CUE). Rabs are shown in light blue and GEFs are shown in green hues.
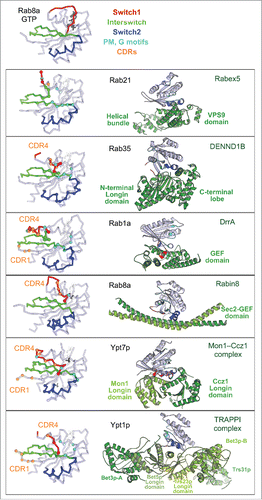
All GEFs first form a low affinity complex with nucleotide-bound Rabs and then convert this complex to a high affinity nucleotide-free Rab/GEF complex, thus releasing nucleotide. GEF specificity for a given Rab molecule is not only linked to its ability to first associate with this particular Rab-GDP molecule but also to its capacity to deform Rab and displace the nucleotide. In each complex, the Rab Switches have been remodeled so as to induce opening of the nucleotide binding pocket, but each GEF destabilizes the Rab surface differently (). The formation of the high affinity nucleotide-free state with GEF involves critical sequence determinants found in the Switch1-Interswitch-Switch2 region and is extended to additional subfamily specific CDR1 and CDR4 regions. Several factors thus influence GEF recognition and activity for a particular Rab, including the ability of the Rab G-domain to be reversibly remodeled. Several structural families of GEFs use related principles but have distinct detailed mechanisms, allowing for exquisite specificity in Rab activation on a particular compartment.
Specific recognition of the Rab active form by cellular effectors
The large number of Rabs present in a cell means that Rab/effector interactions must be highly specific. Effectors associate preferentially with the active form of Rab, mainly recognizing the fairly conserved Switch1-interswitch-Switch2 surface in GTP-bound active form. Their specificity implies that they are able to distinguish between the various active Rabs in spite of their overall structural similarities (). Rab effectors are highly divergentCitation56 – most of which are multi-domain proteins, allowing them to combine different functions with Rab specific recruitment mediated by the so-called Rab binding domain (RBD). Some effectors can also be recruited by several different Rabs,Citation2,56,57 either by involving adjacent RBDs or by sharing a particular RBD. RBDs can correspond to various structural motifs and often contain 2 helices that form the center of the Rab/effector interface with the Rab Interswitch region, but these helices are not positioned with a strict or predictable orientation.Citation58,59 Structures of Rab/RBD complexes, together with quantitative binding assays and mutational analyses, have provided essential insights into the features of the Rab proteins that determine the specificity and promiscuity of Rab/effector recognition. Here we provide structural examples showing how sequence differences in Rabs can modify the properties of this conserved Switch1-Interswitch-Switch2 surface () and thus define effector specificity.
A group of Rabs undergo little-to-no conformational changes in their GTP-bound conformation upon binding to the RBDs of different effectors, suggesting little structural plasticity for their active forms
For instance, minimal changes in the active form of Rab5 functional subgroup members (Rab5 and Rab22) () occur upon binding to specific Rab5 effectors (such as EEA1, Rabaptin5 and Rabenosyn5) that mediate endosomal membrane tethering and fusion;Citation2 or the bacterial effector VipDCitation60 when they are co-opted during intracellular bacterial infection. Structural studies have shown that the recognized epitope on Rab5 is restricted to the Switch1-Interswitch-Switch2 surface for all these effector's RBDsCitation60–63 (). Selectivity for Rab5 results in large part from non-conserved residues found on this Rab epitope that form critical subfamily recognition determinants in RabF1 and Switch2 (circles in ).Citation62 However, a Rab4 mutant, in which all surface residues involved in Rab5 RBD binding are mutated to the corresponding Rab5 sequence, still cannot recognize the Rab5 effectors.Citation63 Mutations of additional core residues are necessary to convert Rab4 to a Rab5 RBD binder.Citation63 Indeed, this crucial binding surface of Rab, in particular the hydrophobic triad at its center (), is influenced by non-conserved core residues that precisely determine the epitopes recognized by the RBDs.Citation27
Figure 7. Rab effector binding surface. Structures of Rab-GTP molecules bound to effectors are shown (cartoon) and superimposed (line) to represent their effector binding sites. Residues changing their solvent assessable area upon binding to the effectors are shown in black lines, hydrophobic triad residues are shown in yellow sticks. (A) Rab5/22-effector complexes. Structures of homologous Rab5 and Rab22 bound to their effectors' RBDs, including EEA1-C2H2-RBD (3MJH), Rabaptin5-Cterminal-RBD (Rapt5; 1TU3), Rabenosyn5-(728–784)-RBD (RBSN5; 1Z0J), and VipD (4KYI), are shown. The superimposed structures illustrate that the effector binding site is similar among different Rab-effector interactions. Rab5/22 specific residues contributing to binding specificity are highlighted with cyan circles. (B) Rab3/27-effector complexes: Rab3a/Rabphilin3a-RBD (RPH3a; 1ZBD), Rab27a/Exophilin4-RBD (EXPH4; 3BC1), and Rab27b/Melanophilin-RBD (MLPH; 2ZET). The effector binding site is extended to the CDR1–3 regions. Cyan circles highlight residues that are different between Rab3 and Rab27, contributing to Rab27 selectivity. (C) Rab11a bound to the RBDs of effectors Rab11FIP2 (4C4P), Rab11FIP3 (2HV8), MyosinVa (MyoVa; 5JCZ), and MyosinVb (MyoVb; 4LX0) are shown. The binding site undergoes remodeling in Switch1, Switch2 and the hydrophobic triad. (D) Rab6a binds 2 effectors RBDs, GCC185 (3BBP) and Rab6IP1 (3CWZ), in different conformations. (E) Rab7a binding to RILP-RBD (1YHN) induces remodeling of the C-terminal CDR3 region that forms an additional β-strand (β7). (F) Rab1b, Rab8a and Rab10 interact with Mical-family (Mical-cl and Mical1) (5SZH, 5SZI, 5LPN, 5SZJ) RBDs using similar binding sites except for the CDR1 region, which modulates the partners binding affinities. In the case of Rab8a, complex structures of it bound to different effectors' RBDs, including OCRL1 (3QBT), Mical-cl (5SZI) and LidA (3TNF), demonstrate subtle structural rearrangements in Switch1 and Switch2.
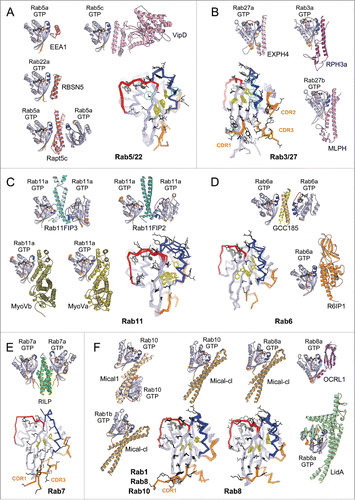
CDRsCitation28 can also contribute to the specificity of Rab/effector recognition (). As for Rab5, no significant conformational change is observed among structures available for the active form of Rab3 and Rab3a/Rabphilin3A;Citation28,64 and phylogenetically related Rab27 binds its Exophilin4 and Melanophilin RBDs in similar conformations.Citation65,66 The CDRs are part of the interface for these 3 complexes; and the role of the CDR1 and Switch2 residues in providing specificity for Rab27 (but not Rab3) binding to Melanophilin () has been demonstrated.Citation66 Thus, some activated Rabs possess exquisite selectivity in effector binding via Rab-specific residues found in their relatively rigid binding epitopes.
For another group of Rab/effector, structures have shown that conformational plasticity of their GTP-bound states is extensive and may broaden their ability to bind different effectors with specificity
Several Rabs undergo distinct conformational changes upon binding to their different effectors. Comparison of the active form of Rab11 bound to the RBDs from Rab11 family interacting proteins (Rab11FIP)Citation67–69 shows large conformational changes in Switch2; while remodeling of both Rab11 Switches is induced upon binding to the MyosinV (MyoV) RBDsCitation70,71 (). Rab6 also interacts with 2 structurally unrelated effectors (GCC185 and Rab6IP1) in different conformationsCitation72,73 (). For these GTPases, rearrangements of the Switches and of the hydrophobic triad residues upon effector binding leads to adaptation of the Rab binding epitope. In addition, the hypervariable tail of Rab6 also participates in binding to GCC185, increasing the affinity of this interaction.Citation73 A drastic remodeling of Rab7 also occurs upon binding to the RILP RBD.Citation74 The Rab7 C-terminal helix and part of the following HVD together refold into an additional β strand that interacts directly with the effectorCitation74 (). On the other hand, binding of Rab8 to different effectors (LidA,Citation75 Mical,Citation76 OCRL1Citation77) leads only to moderate conformational differences in the Switches conformations (). Interestingly, phylogenetically related Rab1, Rab8 and Rab10 adopt very similar conformations when bound to homologous RBDs of the Mical family proteins,Citation76 likely induced (or stabilized) by binding to these RBDs (). The sequence variability in the CDR1 of these Rabs modulates their binding affinities to the Mical-family RBDs.Citation76 The ability of these Rabs to undergo remodeling may promote their promiscuity for effector binding, while the selectivity may result from unique determinants in their sequences that control the degree of their conformational pliancy or allow selective extension of the binding site.
Some Rab effectors can bind to a wide range of Rabs
OCRL1 is a promiscuous effector that binds Rabs from different functional groups: Rab1, Rab5, Rab6, Rab8, Rab14, and Rab35.Citation78–80 Rabs localized to the Golgi and endosomal membrane compartments recruit OCRL1,Citation78,79 promoting changes in the phosphoinositide composition of associated membranes by using the 5-phosphatase domain activity of OCRL1. The crystal structure of Rab8 in complex with OCRL1-RBDCitation77 indicates that the OCRL1-RBD achieves Rab binding promiscuity by a combination of tight contacts with conserved Rab residues of the Switch-Interswitch surface (although the hydrophobic triad is not fully engaged in the interactions) as well as contacts with main chain atoms, reducing the complex interface dependence on the Rab sequence.Citation77,81
Thus, a few non-conserved substitutions in the core or the surface of an individual Rab modulate the conformational plasticity and the properties of the most conserved surface of the Rab G-domain. Structural remodeling can however contribute to either promiscuity or specificity in Rab effectors recognition.
Specific recognition of Rab proteins by GAP: Deactivation by stimulating GTP hydrolysis
Rab GAP proteins associate with a GTP-bound Rab and promote GTP hydrolysis, resulting in the GDP-bound inactive Rab and thus controlling the lifetime of Rab's active form. Most Rab GAPs identified so far in eukaryote contain a catalytic α-helical TBC (Tre-2/Cdc16/Bub2) domainCitation82,83 (). TBC domain stimulates Rab GTP hydrolysis using the dual trans-finger mechanism.Citation84 The trans-Gln-finger in the conserved YxQ motif helps positioning a nucleophilic water, while the trans-Arg-finger in the IxxDxxR motif stabilizes the partial negative charge on the GTP γ-phosphateCitation84 (). Most TBC Rab GAPs act promiscuously toward several Rabs in vitro.Citation82–84 Importantly, however, they have specificity in vivo, either due to their localization or additional regulatory factors.Citation82,83 GAP localization thus defines their specificity and the termination point of Rab's activity. In cells, GAP localization is often determined by the interaction of the domains adjacent to the GAP-domain with other partners such as specific phospholipid.Citation82,83
Figure 8. Structures of Rab-GAP complexes and the mechanisms of GAP stimulated GTP hydrolysis. Representative Rab/RabGAP complexes: Rab33b/Gyp1-TBC-domain (2G77), Rab1a/VirA-TBC-like-domain (4FMB), and Rab1a/LepB-GAP-domain (4IRU). (A) TBC domain Gyp1 and bacterial RabGAP VirA have different folds but stimulate Rab GTP hydrolysis using the same, dual trans-finger mechanism exploiting the trans-Gln-finger and trans-Arg-finger from the conserved TBC YxQ and IxxDxxR motifs, respectively. The nucleophilic water is shown as a pink sphere. The cis-Gln in the Rab Switch2 PM3 motif contributes to GAP binding. (B) LepB, a bacterial RabGAP, binds to Rab1a and generates a trans-cis polar network where the GAP supplies the trans-Arg-finger and trans-Glu to the GTPase hydrolytic site and the Rab provides the cis-Gln from the PM3 motif, Ser residues from the P-loop and Switch1, and the Arg from Switch2. The Switch1 Tyr contributes to destabilization of the Rab ground state as well as binding to LepB.
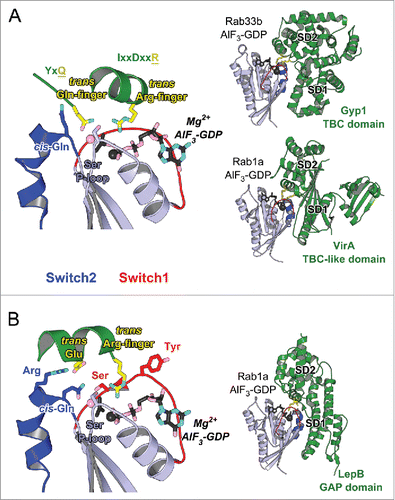
Rab GAPs of different folds are also found in pathogenic bacteria that can manipulate host Rab pathways. Two such Rab1 GAPs, VirA from Shigella flexneri and EspG from enteropathogenic Escherichia coli,Citation85 adopt an α/β fold. However, they possess the characteristic TBC sequence motifs, including the catalytic glutamine- and arginine-fingers,Citation85 and use a dual trans-finger catalytic mechanism analogous to TBCCitation46,84-86 (). In contrast to the broader specificity of these TBC and TBC-like GAPs, LepB from Legionella pneumophila (L. pneumophila) has been shown to be a specific bacterial GAP for Rab1.Citation87 Structures of Rab1 in complex with LepB show that it uses a cis-glutamine from Switch2 PM3 motif to position the hydrolytic water molecule in the active site, and a trans-arginine-finger to help stabilizing the partial negative charge on the GTP γ-phosphateCitation87–89 (). A polar network involving a trans-glutamate and a cis-arginine from Switch2 RabF3 motif as well as non-conserved Rab serines from P-loop and Switch1 extends the hydrolytic site in LepB/Rab1 and, together with the non conserved Switch1 Tyr residue involved in GAP binding and destabilizing the Rab GTP-bound state, they contribute to the selectivity of the GAP activity for Rab1Citation87 (). The variability in the Rab residues surrounding the GTP γ-phosphate suggests the potential existence of other GTP hydrolysis mechanisms that may not use arginine- or glutamine-fingers.Citation83
The Rab GAP folds are composed of 2 subdomains that together surround the Rab G-domain. One of them contributes to Rab binding via the critical binding surface used by effectors (Switch1-Interswitch-Switch2), including the hydrophobic triad (). The other domain completes the interaction surface via contacts with Switch1 and Switch2 as well as the PM1 loop, and it positions the catalytic fingers and stabilizes the active site in a hydrolysis competent configuration. Pronounced remodeling of the Rab structure occurs upon GAP binding. The Gyp1-TBC remodels the Rab33 Switch2 conformation upon binding while other TBC-like GAPs change the conformation of Switch1 in Rab1. GAP binding thus displaces residues, such as the Switch1 tyrosine in Rab1, so as to position the arginine-finger in the active site.Citation85,87 Comparison of these structures highlights how the Rab can be differently remodeled to adopt a catalytic competent conformation.
In conclusion, investigations to delineate cellular Rab/partners interactions are essential since prediction of specificity is not possible from sequence alone. The critical Switch1-Interswitch-Switch2 surface of the G-domain regulates how a particular Rab controls its association with diverse protein effectors in a regulated manner. Some determinants of specificity, unique for Rab regulators and Rab effectors, have been discovered. Despite its rather high sequence conservation, the ability of the Switch1-Interswitch-Switch2 surface to undergo conformational plasticity drastically broadens its potential for partner binding, likely promoting both specificity and promiscuity. This small survey of structural insights illustrates the wealth of opportunities for Rab G-domain to form specific interactions. The Rab family thus represents an exquisite set of compartment identifiers that can regulate multiple events required for complex trafficking in cells.
Integration of Rab activities: Control of complex cellular events
How Rabs coordinate specific functionalities on membranes
Rabs coordinate diverse membrane functionalities, requiring effectors that work together with Rabs to define the fate of cargos and lipid membranes on a particular cellular compartment. The presence of active Rabs on the compartment determines whether effectors can be recruited for subsequent activation of downstream signaling pathways. However, Rab localization to a compartment may also depend on the presence of effectors as it has been shown for the Rab7 effector RILPCitation25: recognition of the HVD by RILP is critical for Rab7 localization on late endosomal/lysosomal compartments. Rab9 HVD is also important for its late endosome localization regulated by its effector TIP47.Citation90 In addition, effectors and Rabs may either belong to the same membrane (interaction in cis) or to 2 different compartments that they help to tether (interaction in trans).Citation91,92 Effector binding to a Rab often goes beyond just its specific recruitment to a compartment, as illustrated below by several examples. Trafficking and exchange between compartments can require several Rabs along a particular pathway, resulting in another level of complexity. Key regulators have the ability to bind and control the activity of different Rabs simultaneously and in a coordinated manner. Crosstalks between different Rab GTPases are frequently promoted by effectors that possess or associate with GEF or GAP domains that can activate or inactivate another Rab. These effectors can effectively enrich or deplete a membrane compartment with other Rabs and play key roles in establishing the spatiotemporal regulation of vesicle trafficking.Citation93 A selection of recent structural studies illustrates how functions can be triggered by Rab/effectors recognition on membranes.
Activation of effector functions upon recruitment onto Rab compartments
Some Rab GTPases can turn on the enzymatic activity of their effectors while recruiting them onto the membrane. The clustering of effector enzymes by Rab binding can locally enhance their activity. Prior to Rab recruitment, some effectors may be stabilized in an auto-inhibited state via intramolecular interactions, therefore precluding their activity. Interaction with specific Rabs can switch ON these effectors by destabilizing the inhibitory intramolecular interactions. This allows spatiotemporal control of enzymes and molecular motors, preventing uncontrolled activity before recruitment. We provide below few examples of functionality onset for some Rab/effectors.
Effector recruitment can lead to the onset of specific enzymatic activities on membrane
An example is OCRL1, which can interact with distinct Rabs for its recruitment to Golgi and endosomal compartments as well as to the lamellipodia upon growth factor stimulation.Citation78,94 Its lipid phosphatase activity is directly stimulated upon Rab5 and Rab6 binding in vitro,Citation78 suggesting that Rabs may activate OCRL in vivo upon its recruitment. Rab5 and its effector APPL1 (that also directly binds to the OCRL1 Rho-GAP-domain) recruit OCRL1 and cooperate in phosphoinositide remodeling of phagocytic membranes.Citation95 Precise spatial and temporal activation of Rab35 by a combined action of its GEF DENND1A and its GAP EPI64 is also a switch for OCRL recruitment to the endosome just after clathrin-coated vesicle (CCV) scission from the plasma membrane.Citation80 This timely regulated hydrolysis of PI(4,5)P2 by OCRL1 is essential after CCV formation since it promotes uncoating and cargo sorting. The structure of OCRL1 bound to Rab8Citation77 can be used to propose a model of the active form of the enzyme bound to the membrane, indicating the central position of the RBD and the importance of the Rab complex to recruit and activate the enzyme. This structure also helps to understand the structural consequences of identified mutations of OCRL1 that lead to the oculocerebrorenal syndrome of Lowe (OCRL), a multi-organ disorder characterized by congenital cataracts, mental retardation and renal Fanconi syndrome.Citation94 Altogether, the promiscuity of OCRL1 for different Rabs triggers rapid onset of enzyme activity to precisely control lipid composition on distinct cellular compartments.
Another example, Mical1, is a regulated redox enzyme and an effector of Rab35. In the cytosol, Mical1 adopts a catalytically incompetent, auto-inhibited conformation whereby the C-terminal region (including the RBD) interacts with its upstream domainsCitation96,97 (). The association of its RBD with Rab35 enhances the oxidation-mediated depolymerisation of F-actin in vitro, suggesting a model of Mical1 conformational activation by RabCitation98 (). Mical1 recruitment and activation by Rab35 to the inter-cellular bridge is critical for cytokinesis since successful abscission requires clearance of F-actin at the abscission site. This then allows recruitment of ESCRT-III to drive the final constriction to complete the separation of daughter cells.Citation98 Several Mical family members interact with a subset of Rabs from Rab1- and Rab8- functional subgroups, suggesting a similar activation mechanism upon Rab/effector interaction.
Figure 9. Effector conformational activation. (A) A schematic model of Mical1 activation upon Rab35 binding. Mical1s C-terminal domain, including the RBD, engages in auto-inhibitory interactions with its FAD-CH-LIM domains. Rab35 binding to the C-terminal domain promotes Mical1 enzymatic activity presumably by destabilizing the auto-inhibited state. (B) Dimeric EEA1 associates with early endosomes by binding to PI(3)P with its C-terminal FYVE domain. Its extended coiled-coil allows the N-terminal C2H2Zn2+ RBD to bind to Rab5-GTP, thereby capturing another early endosome. This interaction induces entropic collapse of its coiled-coil region, bringing 2 membranes together before endosomal fusion. (C) Rab11 cooperates with Spir in MyosinV activation and membrane recruitment. Spir and MyoV proteins adopt a back-folded, auto-inhibited conformation in the cytoplasm. Spir's globular tail binding motif (GTBM) binds to the inhibited MyoV and contributes to the release of MyoV auto-inhibition and facilitates MyoV-GTD interaction with Rab11 on vesicles. Together, they stabilize MyoV in an activated, extended conformation on the membrane. Spir's FYVE domain binds to the membrane and the WH2-KIND domains are available for interaction with Formin1 and F-actin nucleation.
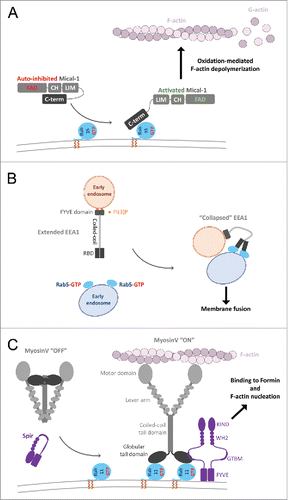
Finally, the L. pneumophila VipD phospholipase A1 activity is only triggered upon binding to endosomal Rab5 or Rab22.Citation99 Rab5 binding to VipD RBDCitation60 allosterically repositions an active site obstructing loop. This opens the phospholipase active site entranceCitation60 () and switches on its activity for PI(3)P depletion,Citation60 progressively leading to the loss of endosomal markers such as EEA1. Alteration of the composition of this compartment renders it incompetent for fusion with Legionella-containing vacuoles.Citation60,99
Effector functionality resulting from a critical conformational change upon Rab binding
EEA1, a specific Rab5 effector, mediates membrane tethering to promote endosomal fusion. EEA1 is a homodimer containing an extended coiled-coil with an N-terminal Rab5 specific RBD (C2H2 zinc finger) and a C-terminal PI(3)P binding FYVE domain, allowing tethering of PI(3)P- and Rab5-endosomes.Citation63 Interestingly, binding to Rab5-GTP induces an extensive allosteric conformational change in EEA1 ().Citation100 It has been suggested that separation of the C2H2 zinc finger domains within the EEA1 dimer may occur upon Rab5 binding, resulting in perturbation of the quaternary organization of its proximal coiled-coil.Citation92 As a result, EEA1 shifts from an extended conformation to a flexible and collapsed one, thus generating a crucial entropic force that pulls the captured Rab5 vesicle toward the PI(3)P membrane and initiating docking and fusion.Citation100
Motor recruitment and onset of motility
Vesicle movement and tethering require specific recruitment of molecular motors. Activation of motor activity upon Rab recruitment has been most studied in the case of MyosinV. Molecular motors such as dimeric MyosinV adopt an auto-inhibited stateCitation101 in which the motor domains interact with the C-terminal globular tail domains (GTD), thus forming a cytosolic pool of inactive motors (). This auto-inhibition has been shown to be critical for MyosinV function in vivo.Citation102 Rab11 binding to the MyosinV-GTD () or the presence of μM level of Ca2+ activates the motor in vitro by destabilizing the auto-inhibited state of MyosinV.Citation103 However, in the cell, endogenous Rab11 is not sufficient to recruit the auto-inhibited motor to the membranes.Citation104 Simultaneous binding of the actin regulator Spir and Rab11 to 2 distinct sites of the GTD is necessary to trigger the recruitment of MyosinV to vesicles ().Citation71 Thus, both MyosinV and Spir are co-recruited to the membrane by Rab11; and the coordination between MyosinVb and Spir activities is important for the long-range transport of Rab11 vesicles toward the cell cortex at metaphase and for the nucleus positioning in prophase in course of meiotic maturation of mouse oocytes.Citation105,106
In addition, MyosinVa is activated by direct interaction with the Rab27 effector Melanophilin.Citation107 This ternary interaction links the motor to melanosome for actin-based motility.Citation108 A model of the MyosinV auto-inhibited state suggests that Rab11 and Spir binding is not compatible with the myosin auto-inhibitory interactions, and thus stabilizes the active form of the motor.Citation71 Interestingly, Spir, Melanophilin and another Rab27 effector Granuphilin share the same binding site on MyosinV-GTD,Citation70,71,109 suggesting a common mechanism of MyosinV activation by these partners.
How Rab-recruitment triggers activity in auto-inhibited effectors is often unclear. Several different activation scenarios are possible in a cell. Rab binding may activate and stabilize the effector in its active conformation on the membrane, triggering the onset of activity. Alternatively, Rab may recruit an effector to a membrane in its auto-inhibited state and simply allow the effector to be transported to its designated cellular compartment where it will be activated by other means (calcium, lipids, post-translational modifications, other partner binding etc.). If the RBD is masked by the auto-inhibition, initial pre-activation by other signals might be necessary before Rab can recruit and maintain the activated effector on the membrane. Structures of the auto-inhibited effectors and further mechanistic studies are required to shed light on the mechanisms of Rab recruitment and effector activation, and how they may coordinate with each other.
The functional networks of Rabs and Rab-interacting proteins
Dual effector binding on a single Rab molecule
The ability for a Rab molecule to simultaneously bind to 2 effectors has been demonstrated for Rab11
Recent studies on Rab11 in complex with Rabin8 or PI4KIIIβ (phosphatidylinositol 4-kinase) have identified a specific second effector binding site on Rab11 adjacent to the Switch-Interswitch canonical effector binding surfaceCitation31,32 ( and ). This peculiar binding site can be recognized by the structurally diverse RBDs from Rabin8 and PI4KIIIβ. The 2 effector binding sites on Rab11 are sufficiently distant to allow simultaneous binding of 2 effectors,Citation31,32 thus promoting cooperation between effectors.
Figure 10. Rab dual-effector binding mode. (A) Non-canonical second effector binding site on Rab11a. Structures of PI4KIIIβ/Rab11a (4D0L, left) and Rabin8-RBD/Rab11a (4UJ5, right) complexes and the superimposition of their respective Rab11a (center) are shown. Residues changing solvent accessible area upon binding to the effectors are shown in black lines (middle). (B) PI4KIIIβ/Rab11a/Rab11FIP3-RBD complex structure (4D0M). Two Rab11a molecules bind to the Rab11FIP3-RBD dimer using the canonical effector-binding surface; and each binds to a PI4KIIIβ using the second binding sites. (C) A model of the Rab11-Rabin8-Rab8 cascade. Rabin8-RBD/Rab11a/Rab11FIP3-RBD complex structure (4UJ3, right) showing Rabin8-RBD dimer binds 2 Rab11a molecules using the second effector binding sites, while Rab11FIP3 interacts with Rab11a via the canonical site. Few direct contacts are observed between Rab11FIP3 and one of the Rabin8 RBDs. Rab11/Rab11FIP3 recruits Rabin8 by binding to its C-terminal dimeric RBD. Subsequently, the Rabin8 GEF domain can activate Rab8 (4LHX, left) and facilitate its membrane recruitment.
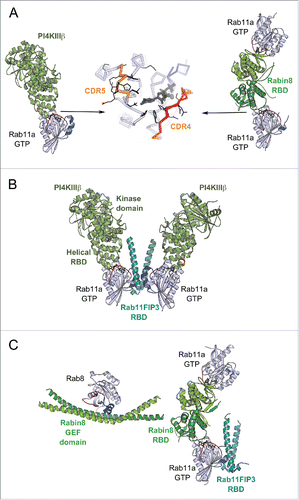
The structure of the ternary PI4KIIIβ-RBD/Rab11/Rab11FIP3-RBD complex () demonstrates how PI4KIIIβ can coordinate Rab11 and its effector Rab11FIP3 on PI(4)P-enriched membranesCitation32 besides its function in the synthesis of PI(4)P at Golgi and TGN. PI4KIIIβ can be recruited to the membrane either by direct interaction with Golgi resident proteins such as ACBD3 (acyl-CoA binding domain containing 3)Citation110 or in an Arf1-dependent manner.Citation111 Golgi localized PI4KIIIβ is required for Rab11 recruitment to the membraneCitation111 as well as for the consecutive recruitment of its down-stream effectors to this compartment, including Rab11FIP3.Citation112 Thus, this second effector binding site might be implicated in Rab11 membrane targeting. Furthermore, the interplay between Rab11 and the 2 effectors is essential for cytokinesis. PI4KIIIβ is required for localization of secretory organelles containing both PI(4)P and Rab11 at the cleavage sitesCitation112 and Rab11FIP3 also contributes to targeting of the Rab11 vesicles to the cleavage furrow to complete cytokinesis.Citation113 The PI(4)P-enriched membranes define proper localization of another Rab11 effector, Golgi phosphoprotein 3 (GOLPH3), that is required for cleavage furrow ingression.Citation114 In breast cancer cells, overexpressed PI4KIIIβ cooperates with Rab11a to activate Akt, a key central regulator for cell survival, proliferation, and growth.Citation115 Thus, the ability of Rab11 to simultaneously bind 2 effectors using 2 distinct binding sites allows the GTPase to coordinate the proteins and their respective functions in cytokinesis.
The Rabin8-RBD/Rab11/Rab11FIP3-RBD structure () indicates how multiple weak interactions (between Rabin8-RBD and Rab11 second effector binding site, and between Rabin8-RBD and Rab11FIP3-RBD) collaborate to create the complex,Citation31 allowing Rabin8 recruitment to Rab11-Rab11FIP3-positive membranes. Together with the structure of Rab8/Rabin8-GEF-domain complex, the 2 structures illustrate an architecture of the Rab11-Rabin8-Rab8 functional cascade machinery ()Citation116,117 that is essential for vesicle delivery to the ciliary base during ciliogenesis.Citation118 An assembly of the ciliary targeting complex is initiated by Arf4 and its effector/GAP ASAP1, which participate in cargo (rhodopsin) sorting at Golgi membranes and subsequently recruit Rab11FIP3 and Rab11 to the membrane.Citation119 The Rab11FIP3/Rab11 complex then forms a binding platform for Rabin8. The recruitment of Rabin8, the Rab8-specific GEF, initiates the Rab11-Rabin8-Rab8 signaling cascadeCitation116,117 that allows spatial Rab8 activation and conversion of the membrane to a Rab8-positive compartment. This enables the fusion of Rab8-positive vesicles at the ciliary base.Citation116,120 Rab11FIP3, on the other hand, stimulates ASAP1 GAP activity and the subsequent Arf4 removal from the membrane.Citation119 It also can recruit the dynein-dynactin complex for transport via binding to the dynein light chain DLIC-2.Citation121 By simultaneously binding to the 2 multi-functional effectors, Rab11 plays a central role in the crosstalk between Arf4, Rab11 and Rab8 GTPases for the specific maturation of the compartment that is pivotal for endosomal trafficking during primary ciliogenesis and epithelial polarization.
Positive feedback (or feed-forward) loop
The endosomal Rab5 accomplishes self-activation using a positive feedback loop mechanismCitation57 whereby the Rab mediates the recruitment of its own GEF. Rab5 effector Rabaptin5 forms a complex with the Rab5 exchange factor Rabex5,Citation2 allowing their co-recruitment on Rab5-membrane and thereby increasing the concentration of the active Rab5 on the endosomal membrane.
The crystal structure of the minimal Rab5/Rabex5/Rabaptin5 ternary complex reveals the interplay between the Rab5 effector and GEFCitation122 and how the GEF is activated toward Rab547 (). Rabex5s GEF activity is inhibited by intramolecular interactions between its catalytic helical bundle (HB)-Vps9 domain tandem and an auto-inhibitory C-terminal helical region.Citation47 In the Rab5/Rabex5/Rabaptin5 complex, the Rabex5 auto-inhibitory helix is instead engaged in an interaction with the dimeric Rabaptin5 extended coiled-coil, releasing Vps9 domain to bind and activate Rab5122 ( upper part). Thus, upon recruitment of Rabaptin5 by Rab5, Rabex5 is co-recruited to allow fast enrichment of activated Rab5 on endosomal membranes ( lower part). Such a feedback loop is likely important for Rabs such as Rab5 whose intrinsic GTPase activity is higher than others. Considering that the half-life of Rab5 intrinsic GTP hydrolysis at 37°C is only 6 min,Citation123 a time-span shorter than the Rab5-mediated endosome fusion that takes about 10 min,Citation123 additional regulations such as the positive feedback loop mechanism are needed to sustain Rab5 activity on the membrane long enough to complete the process it conveys.
Figure 11. A model of the positive feedback loop mechanism. Crystal structure of minimal Rabaptin5/Rabex5/Rab5 complex (4Q9U, upper part) shows that the extended Rabaptin5 central coiled-coil domain binds to Rabex5s auto-inhibitory helix, whereas Rabex5 helix-bundle-VPS9 GEF unit interacts with the nucleotide free Rab5a. The structure of Rabaptin5-RBD/Rab5-GTP (1TU3, lower part) represents the complex's membrane-binding unit.
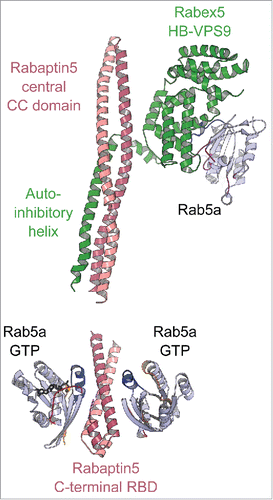
Interestingly, recent studies have demonstrated that the Rabaptin5/Rabex5 complex is in fact recruited first to Rab4 positive endosomes by the Rab4-specific RBD in Rabaptin5 and the Rabex5 ubiquitin-binding domain (recognizing ubiquitylated cargo). Thus, the Rab5 activation is initiated in a feed-forward manner in which Rab4 promotes activation of Rab5 on early endosomes.Citation124
Effectors with 2 (or multiple) Rab binding sites
The Rab effectors that possess adjacent RBDs with distinct specificities for 2 Rab molecules are of special interest since they provide ways to couple the actions of these GTPases and also enable crosstalk between the membrane compartments they are associated with.
Rabenosyn5 is a bifunctional effector of Rab5 and Rab4, connecting entry and recycling sites on early endosomes.Citation125,126 The effector is required for early endosome fusion, either homotypically or with clathrin coated vesicles.Citation127 After the uptake of transferrin receptors from the plasma membrane, the vesicles are selectively delivered to a specific endosome subpopulation containing Rabenosyn5 (likely via Rab5) and subsequently recycled toward the plasma membrane (likely via Rab4). Rabenosyn5 dual effector property may thus be critical to determining the fate of transferrin receptors internalized by clathrin-mediated endocytosis.Citation125–127 More broadly, Rabenosyn5, Rab5 and Rab4 promote endo/exocytic cycles of critical cargos (membrane-type 1 matrix metalloprotease and β3 integrin) that are required for invadosome formation.Citation128 Structures of Rabenosyn5 adjacent Rab4- and Rab5-RBDs have provided insights into the specificity of the 2 structurally similar RBDs.Citation62 However, the machinery that facilitates endosome tethering, sorting and fusion is complex and involves multiple components such as Rabaptin5, Rabex5, hVPS45 as well as EEA1, hVPS34 and Syntaxin13.Citation56,126 Further structural insights are still needed to understand how these multi-Rab effectors are spatially organized to regulate fusion and sorting of early endosomes.
Mical-family proteins provide another example of a Rab effector with 2 Rab binding sites. The RBD in this case is a monomeric flat 3-helix domain with 2 opposite promiscuous Rab binding sites on the same domain, one of higher affinity than the other.Citation76 Mical-family RBDs interact with a subset of phylogenetically related endosomal Rabs (Rab1, Rab8, Rab10, Rab13, Rab15, and Rab35).Citation76,98 The architecture of the bivalent Mical-RBD/Rab complexes () demonstrates how these effectors can bind and coordinate 2 different Rabs, linking the different functional processes the Rabs regulate or linking Rabs associated with different membranes and contributing to membrane tethering. In addition, Rab35 binding to Mical-l1 promotes the recruitment of downstream Rabs such as Rab8, Rab13, and Rab36 and the Rab36-specific effector JIP4 to recycling endosomes during neurite outgrowth.Citation129 Thus, Mical proteins can concentrate multiple Rabs at the same compartment via their bivalent and promiscuous RBD domains.
MyosinVa has been shown to directly bind to several Rabs on different domains of its C-terminal tail (Rab6 and Rab14 via the central coiled-coil; Rab8 and Rab10 via the alternatively spliced exonD region; and Rab3, Rab11 and Rab25 via the GTD).Citation104 Whether crosstalk can be facilitated via these multiple binding sites is presently unknown. MyosinVa is mainly found on Rab11 and Rab10 intracellular membranes, although it is also required for maintaining a peripheral distribution of Rab14-positive endosomes.Citation104 Moreover, melanophilin can indirectly link MyosinVa to Rab27 compartmentsCitation130 to regulate melanosome transportCitation108; mutations in any one of the complex components are associated with Griscelli disease.Citation131 In turn, Rab11FIP2 links MyosinVb to Rab11 recycling endosomes.Citation132 MyosinVb also mediates apical trafficking in epithelial cells.Citation133,134 In addition, microvilli establishment requires the interaction between Rab8a and MyosinVb, while the interaction between Rab11a and MyosinVb mediates apical recycling.Citation135 Importantly, mutations in MyosinVb cause microvillus inclusion disease, a severe form of congenital diarrhea linked to deficits in apical absorption, loss of microvilli, aberrant junctions, and losses in transcellular ion transport pathways.Citation135–137 More investigations are required to clarify how the motor can participate in different pathways and possibly accomplish different tasks depending on its associated Rabs.
Pathogenic bacteria hijacking Rabs and their partners
Dynamic exchange between cellular compartments is essential for life of eukaryotes and its processes can be hijacked by pathogens upon invasion. Intracellular bacterial pathogens successfully evade degradation by inhibiting phagosome maturation in the host cell and transforming it into a pathogen-containing vacuole. Such pathogens convey virulence factors into the host cell cytoplasm, which manipulate Rab and Arf functions to protect the newly formed pathogen-containing vacuoles from destruction.Citation9
Structural studies of these virulence factors have provided insights into the specific strategies the pathogen uses to compete with the cellular partners of Rab1. For example, Legionella pneumophila takes control of the trafficking of the endoplasmic reticulum (ER)-derived Rab1 positive vesicles and masks Legionella-containing vacuoles (LCV) into ER-like compartments that resist lysosomal fusion.Citation9 The bacteria uses a potent Rab1-GEF (DrrA),Citation49,138,139 which can also AMPylate Rab1,Citation29 and a high-affinity effector (LidA)Citation75 to successfully compete with the binding of regulatory proteins and antagonize Rab1 removal from LCVs.Citation140 Modification of the lipid composition of early endosomes by the bacterial phospholipase (VipD) also contributes to protecting the phagosomes from degradation.
DrrA, itself targeted to the LCV through interaction of its C-terminal domain with PI(4)P,Citation49,139 efficiently recruits and activates Rab1 via its GEF domain.Citation49,138,139 The N-terminal nucleotidyltransferase domain of DrrA then AMPylates the Rab1b Switch2 tyrosine (part of the hydrophobic triad).Citation29 The structure of the AMPylated-Rab1Citation29 () shows how the covalently attached bulky AMP moiety changes the partner binding surface. The modification blocks binding of GDI and abolishes nucleotide hydrolysis stimulation by Rab1GAPs (human TBC1D20 or LepB, a Legionella secreted protein with GAP activity).Citation29 Interestingly, the DrrA GEF activity is only moderately affected by the AMPylation. Combined GEF and AMPylation activities of DrrA amplify the recruitment and activation of Rab1 on the LCV and blocks Rab1 retrieval from its membranes.Citation141 Selective recycling of un-ampylated Rab1 by GAPs and GDI leave only modified active Rab1 on the LCV.Citation29
Figure 12. Bacterial Rab regulators. (A) Structure of active Rab1b modified by DrrA (3NKV). GTP analog and Mg2+ are shown in dark gray. The Tyr of Rab1b hydrophobic triad that is AMPylated (yellow) by DrrA is in the central part of the canonical partner binding site. The Rab1 Ser phosphocholination site in the Switch2 is also shown (cyan). (B) Legionella effector LidA bound to Rab8a (3TNF). Rab8a effector binding site (right) includes the canonical partner interaction surface (top) and expands to the adjacent surface (bottom). Rab8a residues changing solvent accessible area upon interaction with LidA are shown in black lines. (C) Legionella effector VipD bound to Rab5c (4KYI). Binding of Rab5c to VipD's helical RBD allosterically induces conformational changes in the phospholipase domain, resulting in opening of the catalytic site and activation of the enzymatic activity.
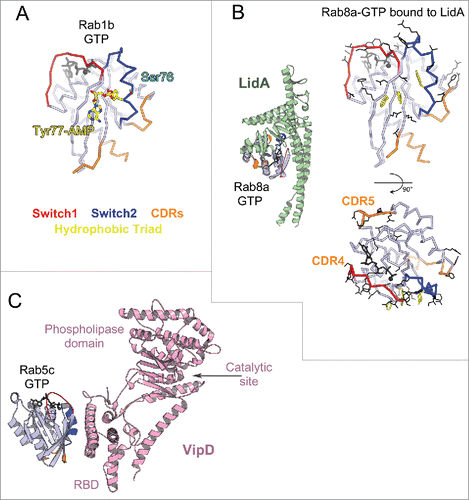
The Legionella LidA effector interacts with Rab1 on the LCV and contributes to preventing Rab1 removal from the endosomal membrane.Citation140 LidA binds several Rabs in vitro (Rab1, Rab6 and Rab8) in both their GTP- and GDP-bound states with very high affinities and can bind AMPylated-Rabs.Citation75 The basis for this high affinity was revealed by the structures of Rab8/LidA-RBD and Rab1/LidA-RBD: while a central coiled-coil of LidA binds Rab via the canonical effector binding site, a big concave binding surface is formed by several other subdomains that embrace the Rab, extending the interaction site to CDR4 and CDR5 as well as helix3 ().Citation75,142 Although the AMPylated Switch2 Tyr directly interacts with LidA, the local distortion at this site might be compensated by the extensive interactions within the unusually big interface of the proteins.
Legionella uses another mechanism for evading degradation. The AnkX and Lem3 proteins perform reversible phosphocholination of a serine/threonine residue in the Switch2 region of Rab1 adjacent to the triad tyrosineCitation143,144 (). These phosphocholinated Rabs cannot undergo GTP/GDP exchange via GEF, neither can they bind to GDI. Their deactivation by GAPs and interactions with Rab-effector proteins (such as LidA and Mical3) are only modestly inhibited. As a result, the phosphocholinated Rabs accumulate in membranes even in the GDP-bound form.Citation145
Another Legionella effector protein, VipD, targets Rab5 to early endosomal membranes and alters their lipid and protein composition, thereby protecting the pathogen from endosomal fusion.Citation60,99 VipD efficiently competes with host Rab5 effectors via its high affinity RBD for Rab560 (), ensuring effective hijacking of the host Rab and redirecting its function. Rab5 binding to C-terminal VipD-RBD allosterically induces the opening of the substrate binding pocket, activating the phospholipase for PI(3)P depletion.Citation60
The wealth of structural information about the interactions between bacterial effectors and host cell Rabs should aid in the fight against pathogens such as Legionella. This could be pursued by screening for specific drugs that revert the efficiency of this machinery, possibly by targeting Rab binding to DrrA.
Understanding complexity: Perspectives on the critical missing information
Rab proteins are essential switches that orchestrate the constant flow of membranes throughout the cell and provide identity to different compartments. A wealth of data has provided important clues on how key effectors regulate Rab switches with specificity. Ongoing efforts to further identify specific regulatory partnersCitation146 and effectorsCitation147 are essential, as well as coupled structural and cell biologic investigations to dissect the role of Rabs in vivo. One obvious gap in the field is the lack of understanding of specific Rab-membrane recruitment mechanisms. In addition, the importance of post-translational modifications in modulating binding of partners and thus the spatial and temporal regulation of Rab functions throughout the cell cycle need to be carefully investigated. The current challenges also include identifying how these different Rab effector proteins cooperate and act in a coordinated manner to accomplish complex membrane trafficking tasks. The extensive and diverse functions resulting from Rab interactions and how they control timely events on lipid membranes are only beginning to be understood.
How Rabs and their partners regulate the cytoskeleton near the membrane has been greatly underappreciated. Recent discoveries showed that activation of a motor can be linked to the nucleation of its actin track around the vesicleCitation71 and that Rab binding controls an enzyme that promotes actin disassembly.Citation98 These findings open up new directions for investigations into the crosstalks between Rabs and cytoskeleton regulators. Recent advances in cryo-electron microscopy and integrative structural biologyCitation148 also open new perspectives for the structural investigation of Rab regulated functional networks involving large multi-protein complexes. Future structural studies of these multicomponent systems combined with modern genome engineering and state-of-the-art high resolution imaging will provide a mechanistic view of the cooperative functions of Rab-effectors, and hold the promise of revealing the innermost workings of the multi-functional Rab family and their interacting partners.
Disclosure of potential conflicts of interest
No potential conflicts of interest were disclosed.
Supplemental_materials.zip
Download Zip (99.1 KB)Acknowledgments
We greatly acknowledge Meg Titus for the manuscript editing and useful suggestions, Arnaud Echard and Bruno Goud for careful reading and helpful comments.
We regret that we could not cite more references and apologize in advance to scientists in the field.
Funding
O.P. and A.H. were supported by grants from INCa (2014–1-PL BIO-04-ICR-1) and ANR-13-BSV8–0019–01. H.H. has been awarded a doctoral fellowship from the PSL University. I.Y. was supported by Marie Curie International Incoming Fellowship (PIIF-GA-2012–330975). A.H., O.P., H.H and I.Y. are a part of Labex CelTisPhyBio 11-LBX-0038, which is a part of the Initiative d'Excellence at PSL Research University (ANR-10-IDEX-0001–02 PSL).
References
- Touchot N, Chardin P, Tavitian A. Four additional members of the ras gene superfamily isolated by an oligonucleotide strategy: Molecular cloning of YPT-related cDNAs from a rat brain library. Proc Natl Acad Sci USA 1987; 84:8210-4 PMID:3317403; https://doi.org/10.1073/pnas.84.23.8210
- Stenmark H. Rab GTPases as coordinators of vesicle traffic. Nat Rev Mol Cell Biol 2009; 10:513-25 PMID:19603039; https://doi.org/10.1038/nrm2728
- Hutagalung AH, Novick PJ. Role of Rab GTPases in membrane traffic and cell physiology. Physiol Rev 2011; 91:119-49 PMID:21248164; https://doi.org/10.1152/physrev.00059.2009
- Diekmann Y, Seixas E, Gouw M, Tavares-Cadete F, Seabra MC, Pereira-Leal JB. Thousands of Rab GTPases for the Cell Biologist. PLoS Comput Biol 2011; 7:e1002217-20; PMID:22022256; https://doi.org/10.1371/journal.pcbi.1002217
- Pereira-Leal JB, Seabra MC. Evolution of the Rab family of small GTP-binding proteins. J Mol Biol 2001; 313:889-901 PMID:11697911; https://doi.org/10.1006/jmbi.2001.5072
- Klöpper TH, Kienle N, Fasshauer D, Munro S. Untangling the evolution of Rab G proteins: implications of a comprehensive genomic analysis. BMC Biol 2012; 10:71; PMID:22873208; https://doi.org/10.1186/1741-7007-10-71
- Vetter IR. The Structure of the G Domain of the Ras Superfamily. In: Ras Superfamily Small G Proteins: Biology and Mechanisms 1. Vienna: Springer Vienna; 2014. pages 25–50
- Zhen Y, Stenmark H. Cellular functions of Rab GTPases at a glance. J Cell Sci 2015; 128:3171-6 PMID:26272922; https://doi.org/10.1242/jcs.166074
- Stein M-P, Müller MP, Wandinger-Ness A. Bacterial Pathogens Commandeer Rab GTPases to Establish Intracellular Niches. Traffic 2012; 13:1565-88 PMID:22901006; https://doi.org/10.1111/tra.12000
- Wittinghofer A, Vetter IR. Structure-function relationships of the G domain, a canonical switch motif. Annu Rev Biochem 2011; 80:943-71 PMID:21675921; https://doi.org/10.1146/annurev-biochem-062708-134043
- Vetter IR, Wittinghofer A. The guanine nucleotide-binding switch in three dimensions. Science 2001; 294:1299-304 PMID:11701921; https://doi.org/10.1126/science.1062023
- Milburn MV, Tong L, deVos AM, Brünger A, Yamaizumi Z, Nishimura S, Kim SH. Molecular switch for signal transduction: structural differences between active and inactive forms of protooncogenic ras proteins. Science 1990; 247:939-45 PMID:2406906; https://doi.org/10.1126/science.2406906
- Sultana A, Jin Y, Dregger C, Franklin E, Weisman LS, Khan AR. The activation cycle of Rab GTPase Ypt32 reveals structural determinants of effector recruitment and GDI binding. FEBS Lett 2011; 585:3520-7 PMID:22024479; https://doi.org/10.1016/j.febslet.2011.10.013
- Lee SH, Baek K, Dominguez R. Large nucleotide-dependent conformational change in Rab28. FEBS Lett 2008; 582:4107-11 PMID:19026641; https://doi.org/10.1016/j.febslet.2008.11.008
- Bergbrede T, Pylypenko O, Rak A, Alexandrov K. Structure of the extremely slow GTPase Rab6A in the GTP bound form at 1.8A resolution. J Struct Biol 2005; 152:235-8 PMID:16332443; https://doi.org/10.1016/j.jsb.2005.10.001
- Grigoriev I, Splinter D, Keijzer N, Wulf PS, Demmers J, Ohtsuka T, Modesti M, Maly IV, Grosveld F, Hoogenraad CC, et al. Rab6 regulates transport and targeting of exocytotic carriers. Dev Cell [Internet] 2007; 13:305–14. Available from: http://www.ncbi.nlm.nih.gov/entrez/query.fcgi?db=pubmed&cmd=Retrieve&dopt=AbstractPlus&list_uids=17681140; PMID:17681140; https://doi.org/10.1016/j.devcel.2007.06.010
- Duclos S, Diez R, Garin J, Papadopoulou B, Descoteaux A, Stenmark H, Desjardins M. Rab5 regulates the kiss and run fusion between phagosomes and endosomes and the acquisition of phagosome leishmanicidal properties in RAW 264.7 macrophages. J Cell Sci 2000; 113(Pt 19):3531–41. PMID:10984443
- Rybin V, Ullrich O, Rubino M, Alexandrov K, Simon I, Seabra MC, Goody R, Zerial M. GTPase activity of Rab5 acts as a timer for endocytic membrane fusion. Nature 1996; 383:266-9 PMID:8805704; https://doi.org/10.1038/383266a0
- Bergbrede T, Chuky N, Schoebel S, Blankenfeldt W, Geyer M, Fuchs E, Goody RS, Barr F, Alexandrov K. Biophysical analysis of the interaction of Rab6a GTPase with its effector domains. J Biol Chem 2009; 284:2628-35 PMID:19019823; https://doi.org/10.1074/jbc.M806003200
- Pfeffer S, Aivazian D. Targeting Rab GTPases to distinct membrane compartments. Nat Rev Mol Cell Biol 2004; 5:886-96 PMID:15520808; https://doi.org/10.1038/nrm1500
- Wu Y-W, Oesterlin LK, Tan K-T, Waldmann H, Alexandrov K, Goody RS. Membrane targeting mechanism of Rab GTPases elucidated by semisynthetic protein probes. Nat Chem Biol 2010; 6:534-40 PMID:20512138; https://doi.org/10.1038/nchembio.386
- Gerondopoulos A, Langemeyer L, Liang J-R, Linford A, Barr FA. BLOC-3 mutated in Hermansky-Pudlak syndrome is a Rab32/38 guanine nucleotide exchange factor. Curr Biol 2012; 22:2135-9 PMID:23084991; https://doi.org/10.1016/j.cub.2012.09.020
- Blümer J, Rey J, Dehmelt L, Mazel T, Wu Y-W, Bastiaens P, Goody RS, Itzen A. RabGEFs are a major determinant for specific Rab membrane targeting. J Cell Biol 2013; 200:287-300 PMID:23382462; https://doi.org/10.1083/jcb.201209113
- Ali BR, Wasmeier C, Lamoreux L, Strom M, Seabra MC. Multiple regions contribute to membrane targeting of Rab GTPases. J Cell Sci 2004; 117:6401-12 PMID:15561774; https://doi.org/10.1242/jcs.01542
- Li F, Yi L, Zhao L, Itzen A, Goody RS, Wu Y-W. The role of the hypervariable C-terminal domain in Rab GTPases membrane targeting. Proc Natl Acad Sci USA 2014; 111:2572-7 PMID:24550285; https://doi.org/10.1073/pnas.1313655111
- Pereira-Leal JB, Seabra MC. The mammalian Rab family of small GTPases: definition of family and subfamily sequence motifs suggests a mechanism for functional specificity in the Ras superfamily. J Mol Biol 2000; 301:1077-87 PMID:10966806; https://doi.org/10.1006/jmbi.2000.4010
- Merithew E, Hatherly S, Dumas JJ, Lawe DC, Heller-Harrison R, Lambright DG. Structural plasticity of an invariant hydrophobic triad in the switch regions of Rab GTPases is a determinant of effector recognition. J Biol Chem 2001; 276:13982-8 PMID:11278565; https://doi.org/10.1074/jbc.M009771200
- Ostermeier C, Brunger AT. Structural basis of Rab effector specificity: crystal structure of the small G protein Rab3A complexed with the effector domain of rabphilin-3A. Cell 1999; 96:363-74 PMID:10025402; https://doi.org/10.1016/S0092-8674(00)80549-8
- Müller MP, Peters H, Blümer J, Blankenfeldt W, Goody RS, Itzen A. The Legionella effector protein DrrA AMPylates the membrane traffic regulator Rab1b. Science 2010; 329:946-9 PMID:20651120; https://doi.org/10.1126/science.1192276
- Guo Z, Hou X, Goody RS, Itzen A. Intermediates in the guanine nucleotide exchange reaction of Rab8 protein catalyzed by guanine nucleotide exchange factors Rabin8 and GRAB. J Biol Chem 2013; 288:32466-74 PMID:24072714; https://doi.org/10.1074/jbc.M113.498329
- Vetter M, Stehle R, Basquin C, Lorentzen E. Structure of Rab11-FIP3-Rabin8 reveals simultaneous binding of FIP3 and Rabin8 effectors to Rab11. Nat Struct Mol Biol 2015; 22:695-702 PMID:26258637; https://doi.org/10.1038/nsmb.3065
- Burke JE, Inglis AJ, Perisic O, Masson GR, McLaughlin SH, Rutaganira F, Shokat KM, Williams RL. Structures of PI4KIIIβ complexes show simultaneous recruitment of Rab11 and its effectors. Science 2014; 344:1035-8 PMID:24876499; https://doi.org/10.1126/science.1253397
- Schwartz SL, Cao C, Pylypenko O, Rak A, Wandinger-Ness A. Rab GTPases at a glance. J Cell Sci 2007; 120:3905-10 PMID:17989088; https://doi.org/10.1242/jcs.015909
- Leung KF, Baron R, Ali BR, Magee AI, Seabra MC. Rab GTPases containing a CAAX motif are processed post-geranylgeranylation by proteolysis and methylation. J Biol Chem 2007; 282:1487-97 PMID:17114793; https://doi.org/10.1074/jbc.M605557200
- Andres DA, Seabra MC, Brown MS, Armstrong SA, Smeland TE, Cremers FP, Goldstein JL. cDNA cloning of component A of Rab geranylgeranyl transferase and demonstration of its role as a Rab escort protein. Cell 1993; 73:1091-9 PMID:8513495; https://doi.org/10.1016/0092-8674(93)90639-8
- Guo Z, Wu Y-W, Das D, Delon C, Cramer J, Yu S, Thuns S, Lupilova N, Waldmann H, Brunsveld L, et al. Structures of RabGGTase-substrate/product complexes provide insights into the evolution of protein prenylation. EMBO J 2008; 27:2444-56 PMID:18756270; https://doi.org/10.1038/emboj.2008.164
- Rak A, Pylypenko O, Niculae A, Pyatkov K, Goody RS, Alexandrov K. Structure of the Rab7:REP-1 complex: insights into the mechanism of Rab prenylation and choroideremia disease. Cell 2004; 117:749-60 PMID:15186776; https://doi.org/10.1016/j.cell.2004.05.017
- Pylypenko O, Rak A, Reents R, Niculae A, Sidorovitch V, Cioaca MD, Bessolitsyna E, Thomä NH, Waldmann H, Schlichting I, et al. Structure of Rab escort protein-1 in complex with Rab geranylgeranyltransferase. Mol Cell 2003; 11:483-94 PMID:12620235; https://doi.org/10.1016/S1097-2765(03)00044-3
- Wu Y-W, Goody RS, Abagyan R, Alexandrov K. Structure of the disordered C terminus of Rab7 GTPase induced by binding to the Rab geranylgeranyl transferase catalytic complex reveals the mechanism of Rab prenylation. J Biol Chem 2009; 284:13185-92 PMID:19240028; https://doi.org/10.1074/jbc.M900579200
- Köhnke M, Delon C, Hastie ML, Nguyen UTT, Wu Y-W, Waldmann H, Goody RS, Gorman JJ, Alexandrov K. Rab GTPase Prenylation Hierarchy and Its Potential Role in Choroideremia Disease. PLoS ONE 2013; 8:e81758-11; PMID:24358126; https://doi.org/10.1371/journal.pone.0081758
- Chavas LMG, Torii S, Kamikubo H, Kawasaki M, Ihara K, Kato R, Kataoka M, Izumi T, Wakatsuki S. Structure of the small GTPase Rab27b shows an unexpected swapped dimer. Acta Crystallogr D Biol Crystallogr 2007; 63:769-79 PMID:17582168; https://doi.org/10.1107/S0907444907019725
- Larijani B, Hume AN, Tarafder AK, Seabra MC. Multiple factors contribute to inefficient prenylation of Rab27a in Rab prenylation diseases. J Biol Chem 2003; 278:46798-804 PMID:12941939; https://doi.org/10.1074/jbc.M307799200
- Pylypenko O, Rak A, Durek T, Kushnir S, Dursina BE, Thomae NH, Constantinescu AT, Brunsveld L, Watzke A, Waldmann H, et al. Structure of doubly prenylated Ypt1:GDI complex and the mechanism of GDI-mediated Rab recycling. EMBO J 2006; 25:13-23 PMID:16395334; https://doi.org/10.1038/sj.emboj.7600921
- Alory C, Balch WE. Organization of the Rab-GDI/CHM superfamily: the functional basis for choroideremia disease. Traffic 2001; 2:532-43 PMID:11489211; https://doi.org/10.1034/j.1600-0854.2001.20803.x
- Wu Y-W, Tan K-T, Waldmann H, Goody RS, Alexandrov K. Interaction analysis of prenylated Rab GTPase with Rab escort protein and GDP dissociation inhibitor explains the need for both regulators. Proc Natl Acad Sci USA 2007; 104:12294-9 PMID:17640890; https://doi.org/10.1073/pnas.0701817104
- Müller MP, Goody RS. Molecular control of Rab activity by GEFs, GAPs and GDI. Small GTPases 2017; 13:1-17; PMID:28055292; https://doi.org/10.1080/21541248.2016.1276999
- Delprato A, Lambright DG. Structural basis for Rab GTPase activation by VPS9 domain exchange factors. Nat Struct Mol Biol 2007; 14:406-12 PMID:17450153; https://doi.org/10.1038/nsmb1232
- Wu X, Bradley MJ, Cai Y, Kümmel D, La Cruz De EM, Barr FA, Reinisch KM. Insights regarding guanine nucleotide exchange from the structure of a DENN-domain protein complexed with its Rab GTPase substrate. Proc Natl Acad Sci USA 2011; 108:18672-7 PMID:22065758; https://doi.org/10.1073/pnas.1110415108
- Schoebel S, Oesterlin LK, Blankenfeldt W, Goody RS, Itzen A. RabGDI displacement by DrrA from Legionella is a consequence of its guanine nucleotide exchange activity. Mol Cell 2009; 36:1060-72 PMID:20064470; https://doi.org/10.1016/j.molcel.2009.11.014
- Itzen A, Pylypenko O, Goody RS, Alexandrov K, Rak A. Nucleotide exchange via local protein unfolding–structure of Rab8 in complex with MSS4. EMBO J 2006; 25:1445-55 PMID:16541104; https://doi.org/10.1038/sj.emboj.7601044
- Dong G, Medkova M, Novick P, Reinisch KM. A catalytic coiled coil: structural insights into the activation of the Rab GTPase Sec 4p by Sec 2p. Mol Cell 2007; 25:455-62 PMID:17289591; https://doi.org/10.1016/j.molcel.2007.01.013
- Kiontke S, Langemeyer L, Kuhlee A, Schuback S, Raunser S, Ungermann C, Kümmel D. Architecture and mechanism of the late endosomal Rab7-like Ypt7 guanine nucleotide exchange factor complex Mon1-Ccz1. Nat Commun 2017; 8:14034; PMID:28051187; https://doi.org/10.1038/ncomms14034
- Cai Y, Chin HF, Lazarova D, Menon S, Fu C, Cai H, Sclafani A, Rodgers DW, La Cruz De EM, Ferro-Novick S, et al. The structural basis for activation of the Rab Ypt1p by the TRAPP membrane-tethering complexes. Cell 2008; 133:1202-13; PMID:18585354; https://doi.org/10.1016/j.cell.2008.04.049
- Langemeyer L, Nunes Bastos R, Cai Y, Itzen A, Reinisch KM, Barr FA. Diversity and plasticity in Rab GTPase nucleotide release mechanism has consequences for Rab activation and inactivation. Elife 2014; 3:e01623; PMID:24520163; https://doi.org/10.7554/eLife.01623
- Chin HF, Cai Y, Menon S, Ferro-Novick S, Reinisch KM, La Cruz De EM. Kinetic analysis of the guanine nucleotide exchange activity of TRAPP, a multimeric Ypt1p exchange factor. J Mol Biol 2009; 389:275-88 PMID:19361519; https://doi.org/10.1016/j.jmb.2009.03.068
- Wandinger-Ness A, Zerial M. Rab proteins and the compartmentalization of the endosomal system. Cold Spring Harb Perspect Biol [Internet] 2014; 6:a022616–6. Available from: http://cshperspectives.cshlp.org/lookup/doi/10.1101/cshperspect.a022616; PMID:25341920; https://doi.org/10.1101/cshperspect.a022616
- Grosshans BL, Ortiz D, Novick P. Rabs and their effectors: achieving specificity in membrane traffic. Proc Natl Acad Sci USA 2006; 103:11821-7 PMID:16882731; https://doi.org/10.1073/pnas.0601617103
- Khan AR, Ménétrey J. Structural biology of Arf and Rab GTPases' effector recruitment and specificity. Structure 2013; 21:1284-97 PMID:23931141; https://doi.org/10.1016/j.str.2013.06.016
- Mott HR, Owen D. Structures of Ras superfamily effector complexes: What have we learnt in two decades? Crit Rev Biochem Mol Biol 2015; 50:85-133 PMID:25830673; https://doi.org/10.3109/10409238.2014.999191
- Lucas M, Gaspar AH, Pallara C, Rojas AL, Fernández-Recio J, Machner MP, Hierro A. Structural basis for the recruitment and activation of the Legionella phospholipase VipD by the host GTPase Rab5. Proc Natl Acad Sci USA 2014; 111:E3514-23; PMID:25114243; https://doi.org/10.1073/pnas.1405391111
- Zhu G, Zhai P, Liu J, Terzyan S, Li G, Zhang XC. Structural basis of Rab5-Rabaptin5 interaction in endocytosis. Nat Struct Mol Biol 2004; 11:975-83 PMID:15378032; https://doi.org/10.1038/nsmb832
- Eathiraj S, Pan X, Ritacco C, Lambright DG. Structural basis of family-wide Rab GTPase recognition by rabenosyn-5. Nature 2005; 436:415-9 PMID:16034420; https://doi.org/10.1038/nature03798
- Mishra A, Eathiraj S, Corvera S, Lambright DG. Structural basis for Rab GTPase recognition and endosome tethering by the C2H2 zinc finger of Early Endosomal Autoantigen 1 (EEA1). Proc Natl Acad Sci USA 2010; 107:10866-71 PMID:20534488; https://doi.org/10.1073/pnas.1000843107
- Dumas JJ, Zhu Z, Connolly JL, Lambright DG. Structural basis of activation and GTP hydrolysis in Rab proteins. Structure [Internet] 1999; 7:413–23. Available from: http://www.ncbi.nlm.nih.gov/entrez/query.fcgi?db=pubmed&cmd=Retrieve&dopt=AbstractPlus&list_uids=10196122; PMID:10196122; https://doi.org/10.1016/S0969-2126(99)80054-9
- Chavas LMG, Ihara K, Kawasaki M, Torii S, Uejima T, Kato R, Izumi T, Wakatsuki S. Elucidation of Rab27 recruitment by its effectors: structure of Rab27a bound to Exophilin4/Slp2-a. Structure 2008; 16:1468-77 PMID:18940603; https://doi.org/10.1016/j.str.2008.07.015
- Kukimoto-Niino M, Sakamoto A, Kanno E, Hanawa-Suetsugu K, Terada T, Shirouzu M, Fukuda M, Yokoyama S. Structural basis for the exclusive specificity of Slac2-a/melanophilin for the Rab27 GTPases. Structure 2008; 16:1478-90 PMID:18940604; https://doi.org/10.1016/j.str.2008.07.014
- Shiba T, Koga H, Shin H-W, Kawasaki M, Kato R, Nakayama K, Wakatsuki S. Structural basis for Rab11-dependent membrane recruitment of a family of Rab11-interacting protein 3 (FIP3)/Arfophilin-1. Proc Natl Acad Sci USA 2006; 103:15416-21 PMID:17030804; https://doi.org/10.1073/pnas.0605357103
- Eathiraj S, Mishra A, Prekeris R, Lambright DG. Structural basis for Rab11-mediated recruitment of FIP3 to recycling endosomes. J Mol Biol 2006; 364:121-35 PMID:17007872; https://doi.org/10.1016/j.jmb.2006.08.064
- Jagoe WN, Lindsay AJ, Read RJ, McCoy AJ, McCaffrey MW, Khan AR. Crystal structure of rab11 in complex with rab11 family interacting protein 2. Structure 2006; 14:1273-83 PMID:16905101; https://doi.org/10.1016/j.str.2006.06.010
- Pylypenko O, Attanda W, Gauquelin C, Lahmani M, Coulibaly D, Baron B, Hoos S, Titus MA, England P, Houdusse AM. Structural basis of myosin V Rab GTPase-dependent cargo recognition. Proc Natl Acad Sci USA 2013; 110:20443-8 PMID:24248336; https://doi.org/10.1073/pnas.1314329110
- Pylypenko O, Welz T, Tittel J, Kollmar M, Chardon F, Malherbe G, Weiß S, Michel CIL, Samol-Wolf A, Grasskamp AT, et al. Coordinated recruitment of Spir actin nucleators and myosin V motors to Rab11 vesicle membranes. Elife [Internet] 2016; 5:213. Available from: http://elifesciences.org/lookup/doi/10.7554/eLife.17523; https://doi.org/10.7554/eLife.17523
- Recacha R, Boulet A, Jollivet F, Monier S, Houdusse AM, Goud B, Khan AR. Structural basis for recruitment of Rab6-interacting protein 1 to Golgi via a RUN domain. Structure 2009; 17:21-30 PMID:19141279; https://doi.org/10.1016/j.str.2008.10.014
- Burguete AS, Fenn TD, Brunger AT, Pfeffer SR. Rab and Arl GTPase family members cooperate in the localization of the golgin GCC185. Cell 2008; 132:286-98 PMID:18243103; https://doi.org/10.1016/j.cell.2007.11.048
- Wu M, Wang T, Loh E, Hong W, Song H. Structural basis for recruitment of RILP by small GTPase Rab7. EMBO J 2005; 24:1491-501 PMID:15933719; https://doi.org/10.1038/sj.emboj.7600643
- Schoebel S, Cichy AL, Goody RS, Itzen A. Protein LidA from Legionella is a Rab GTPase supereffector. Proc Natl Acad Sci USA 2011; 108:17945-50 PMID:22011575; https://doi.org/10.1073/pnas.1113133108
- Rai A, Oprisko A, Campos J, Fu Y, Friese T, Itzen A, Goody RS, Gazdag E-M, Müller MP. bMERB domains are bivalent Rab8 family effectors evolved by gene duplication. Elife 2016; 5:213; https://doi.org/10.7554/eLife.18675
- Hou X, Hagemann N, Schoebel S, Blankenfeldt W, Goody RS, Erdmann KS, Itzen A. A structural basis for Lowe syndrome caused by mutations in the Rab-binding domain of OCRL1. EMBO J 2011; 30:1659-70 PMID:21378754; https://doi.org/10.1038/emboj.2011.60
- Hyvola N, Diao A, McKenzie E, Skippen A, Cockcroft S, Lowe M. Membrane targeting and activation of the Lowe syndrome protein OCRL1 by rab GTPases. EMBO J 2006; 25:3750-61 PMID:16902405; https://doi.org/10.1038/sj.emboj.7601274
- Dambournet D, Machicoane M, Chesneau L, Sachse M, Rocancourt M, Marjou El A, Formstecher E, Salomon R, Goud B, Echard A. Rab35 GTPase and OCRL phosphatase remodel lipids and F-actin for successful cytokinesis. Nat Cell Biol 2011; 13:981-8 PMID:21706022; https://doi.org/10.1038/ncb2279
- Cauvin C, Rosendale M, Gupta-Rossi N, Rocancourt M, Larraufie P, Salomon R, Perrais D, Echard A. Rab35 GTPase triggers switch-like recruitment of the lowe syndrome lipid phosphatase OCRL on newborn endosomes. Curr Biol 2016; 26:120-8 PMID:26725203; https://doi.org/10.1016/j.cub.2015.11.040
- Hagemann N, Hou X, Goody RS, Itzen A, Erdmann KS. Crystal structure of the Rab binding domain of OCRL1 in complex with Rab8 and functional implications of the OCRL1/Rab8 module for Lowe syndrome. Small GTPases 2012; 3:107-10 PMID:22790198; https://doi.org/10.4161/sgtp.19380
- Barr F, Lambright DG. Rab GEFs and GAPs. Curr Opin Cell Biol 2010; 22:461-70 PMID:20466531; https://doi.org/10.1016/j.ceb.2010.04.007
- Frasa MAM, Koessmeier KT, Ahmadian MR, Braga VMM. Illuminating the functional and structural repertoire of human TBC/RABGAPs. Nat Rev Mol Cell Biol 2012; 13:67-73 PMID:22251903; https://doi.org/10.1038/nrm3364
- Pan X, Eathiraj S, Munson M, Lambright DG. TBC-domain GAPs for Rab GTPases accelerate GTP hydrolysis by a dual-finger mechanism. Nature 2006; 442:303-6 PMID:16855591; https://doi.org/10.1038/nature04847
- Dong N, Zhu Y, Lu Q, Hu L, Zheng Y, Shao F. Structurally distinct bacterial TBC-like GAPs Link Arf GTPase to Rab1 inactivation to counteract host defenses. Cell 2012; 150:1029-41 PMID:22939626; https://doi.org/10.1016/j.cell.2012.06.050
- Gavriljuk K, Gazdag E-M, Itzen A, Kötting C, Goody RS, Gerwert K. Catalytic mechanism of a mammalian Rab·RabGAP complex in atomic detail. Proc Natl Acad Sci USA 2012; 109:21348-53 PMID:23236136; https://doi.org/10.1073/pnas.1214431110
- Mishra AK, Del Campo CM, Collins RE, Roy CR, Lambright DG. The Legionella pneumophila GTPase Activating Protein LepB Accelerates Rab1 Deactivation by a Non-canonical Hydrolytic Mechanism. J Biol Chem 2013; 288:24000-11 PMID:23821544; https://doi.org/10.1074/jbc.M113.470625
- Mihai Gazdag E, Streller A, Haneburger I, Hilbi H, Vetter IR, Goody RS, Itzen A. Mechanism of Rab1b deactivation by the Legionella pneumophila GAP LepB. EMBO Rep 2013; 14:199-205 PMID:23288104; https://doi.org/10.1038/embor.2012.211
- Yu Q, Hu L, Yao Q, Zhu Y, Dong N, Wang D-C, Shao F. Structural analyses of Legionella LepB reveal a new GAP fold that catalytically mimics eukaryotic RasGAP. Nature Publishing Group 2013; 23:775–87
- Aivazian D, Serrano RL, Pfeffer S. TIP47 is a key effector for Rab9 localization. J Cell Biol 2006; 173:917-26 PMID:16769818; https://doi.org/10.1083/jcb.200510010
- Klinkert K, Rocancourt M, Houdusse A, Echard A. Rab35 GTPase couples cell division with initiation of epithelial apico-basal polarity and lumen opening. Nat Commun [Internet] 2016; 7:11166. Available from: http://eutils.ncbi.nlm.nih.gov/entrez/eutils/elink.fcgi?dbfrom=pubmed&id=27040773&retmode=ref&cmd=prlinks; PMID:27040773; https://doi.org/10.1038/ncomms11166
- Das S, Lambright DG. Membrane Trafficking: An Endosome tether meets a rab and collapses. Curr Biol 2016; 26:R927-9; PMID:27780062; https://doi.org/10.1016/j.cub.2016.08.056
- Barr FA. Review series: Rab GTPases and membrane identity: Causal or inconsequential? J Cell Biol 2013; 202:191-9 PMID:23878272; https://doi.org/10.1083/jcb.201306010
- Mehta ZB, Pietka G, Lowe M. The cellular and physiological functions of the Lowe syndrome protein OCRL1. Traffic 2014; 15:471-87 PMID:24499450; https://doi.org/10.1111/tra.12160
- Bohdanowicz M, Balkin DM, De Camilli P, Grinstein S. Recruitment of OCRL and Inpp5B to phagosomes by Rab5 and APPL1 depletes phosphoinositides and attenuates Akt signaling. Mol Biol Cell 2012; 23:176-87 PMID:22072788; https://doi.org/10.1091/mbc.E11-06-0489
- Giridharan SSP, Rohn JL, Naslavsky N, Caplan S. Differential regulation of actin microfilaments by human MICAL proteins. J Cell Sci 2012; 125:614-24 PMID:22331357; https://doi.org/10.1242/jcs.089367
- Vitali T, Maffioli E, Tedeschi G, Vanoni MA. Properties and catalytic activities of MICAL1, the flavoenzyme involved in cytoskeleton dynamics, and modulation by its CH, LIM and C-terminal domains. Arch Biochem Biophy 2016; 593:24-37 PMID:26845023; https://doi.org/10.1016/j.abb.2016.01.016
- Frémont S, Hammich H, Bai J, Wioland H, Klinkert K, Rocancourt M, Kikuti C, Stroebel D, Romet-Lemonne G, Pylypenko O, et al. Oxidation of F-actin controls the terminal steps of cytokinesis. Nat Commun 2017; 8:14528; PMID:28230050; https://doi.org/10.1038/ncomms14528
- Gaspar AH, Machner MP. VipD is a Rab5-activated phospholipase A1 that protects Legionella pneumophila from endosomal fusion. Proc Natl Acad Sci USA 2014; 111:4560-5 PMID:24616501; https://doi.org/10.1073/pnas.1316376111
- Murray DH, Jahnel M, Lauer J, Avellaneda MJ, Brouilly N, Cezanne A, Morales-Navarrete H, Perini ED, Ferguson C, Lupas AN, et al. An endosomal tether undergoes an entropic collapse to bring vesicles together. Nature 2016; 537:107-11 PMID:27556945; https://doi.org/10.1038/nature19326
- Thirumurugan K, Sakamoto T, Hammer JA, Sellers JR, Knight PJ. The cargo-binding domain regulates structure and activity of myosin 5. Nature 2006; 442:212-5 PMID:16838021; https://doi.org/10.1038/nature04865
- Donovan KW, Bretscher A. Head-to-tail regulation is critical for the in vivo function of myosin V. J Cell Biol 2015; 160:811
- Ji H-H, Zhang H-M, Shen M, Yao L-L, Li X-D. The motor function of Drosophila melanogaster myosin-5 is activated by calcium and cargo-binding protein dRab11. Biochem J 2015; 469:135-44 PMID:25940004; https://doi.org/10.1042/BJ20141330
- Lindsay AJ, Jollivet F, Horgan CP, Khan AR, Raposo G, McCaffrey MW, Goud B. Identification and characterization of multiple novel Rab-myosin Va interactions. Mol Biol Cell 2013; 24:3420-34 PMID:24006491; https://doi.org/10.1091/mbc.E13-05-0236
- Schuh M. An actin-dependent mechanism for long-range vesicle transport. Nat Cell Biol 2011; 13:1431-6 PMID:21983562; https://doi.org/10.1038/ncb2353
- Almonacid M, Ahmed WW, Bussonnier M, Mailly P, Betz T, Voituriez R, Gov NS, Verlhac M-H. Active diffusion positions the nucleus in mouse oocytes. Nat Cell Biol 2015; 17:470-9 PMID:25774831; https://doi.org/10.1038/ncb3131
- Yao L-L, Cao Q-J, Zhang H-M, Zhang J, Cao Y, Li X-D. Melanophilin stimulates myosin-5a motor function by allosterically inhibiting the interaction between the head and tail of myosin-5a. Sci Rep 2015; 5:10874; PMID:26039755; https://doi.org/10.1038/srep10874
- Strom M, Hume AN, Tarafder AK, Barkagianni E, Seabra MC. A family of Rab27-binding proteins. Melanophilin links Rab27a and myosin Va function in melanosome transport. J Biol Chem 2002; 277:25423-30 PMID:11980908; https://doi.org/10.1074/jbc.M202574200
- Wei Z, Liu X, Yu C, Zhang M. Structural basis of cargo recognitions for class V myosins. Proc Natl Acad Sci USA [Internet] 2013; 110:11314–9. Available from: http://www.pnas.org/cgi/doi/10.1073/pnas.1306768110; PMID:23798443; https://doi.org/10.1073/pnas.1306768110
- Klima M, Tóth DJ, Hexnerova R, Baumlova A, Chalupska D, Tykvart J, Rezabkova L, Sengupta N, Man P, Dubankova A, et al. Structural insights and in vitro reconstitution of membrane targeting and activation of human PI4KB by the ACBD3 protein. Sci Rep 2016;:1–11
- de Graaf P, Zwart WT, van Dijken RAJ, Deneka M, Schulz TKF, Geijsen N, Coffer PJ, Gadella BM, Verkleij AJ, van der Sluijs P, et al. Phosphatidylinositol 4-kinasebeta is critical for functional association of rab11 with the Golgi complex. Mol Biol Cell 2004; 15:2038-47 PMID:14767056; https://doi.org/10.1091/mbc.E03-12-0862
- Polevoy G, Wei H-C, Wong R, Szentpetery Z, Kim YJ, Goldbach P, Steinbach SK, Balla T, Brill JA. Dual roles for the DrosophilaPI 4-kinase Four wheel drive in localizing Rab11 during cytokinesis. J Cell Biol 2009; 187:847-58 PMID:19995935; https://doi.org/10.1083/jcb.200908107
- Wilson GM, Fielding AB, Simon GC, Yu X, Andrews PD, Hames RS, Frey AM, Peden AA, Gould GW, Prekeris R. The FIP3-Rab11 protein complex regulates recycling endosome targeting to the cleavage furrow during late cytokinesis. Mol Biol Cell 2005; 16:849-60 PMID:15601896; https://doi.org/10.1091/mbc.E04-10-0927
- Sechi S, Colotti G, Belloni G, Mattei V, Frappaolo A, Raffa GD, Fuller MT, Giansanti MG. GOLPH3 Is Essential for Contractile Ring Formation and Rab11 Localization to the Cleavage Site during Cytokinesis in Drosophila melanogaster. PLoS Genet 2014; 10:e1004305; PMID:24786584; https://doi.org/10.1371/journal.pgen.1004305
- Morrow AA, Alipour MA, Bridges D, Yao Z, Saltiel AR, Lee JM. The lipid kinase PI4KIIIβ is highly expressed in breast tumors and activates Akt in cooperation with Rab11a. Mol Cancer Res 2014; 12:1492-508 PMID:24962317; https://doi.org/10.1158/1541-7786.MCR-13-0604
- Knödler A, Feng S, Zhang J, Zhang X, Das A, Peränen J, Guo W. Coordination of Rab8 and Rab11 in primary ciliogenesis. Proc Natl Acad Sci USA 2010; 107:6346-51 PMID:20308558; https://doi.org/10.1073/pnas.1002401107
- Wang J, Deretic D. The Arf and Rab11 effector FIP3 acts synergistically with ASAP1 to direct Rabin8 in ciliary receptor targeting. J Cell Sci 2015; 128:1375-85 PMID:25673879; https://doi.org/10.1242/jcs.162925
- Sung C-H, Leroux MR. The roles of evolutionarily conserved functional modules in cilia-related trafficking. Nat Cell Biol 2013; 15:1387-97 PMID:24296415; https://doi.org/10.1038/ncb2888
- Wang J, Deretic D. Molecular complexes that direct rhodopsin transport to primary cilia. Prog Retin Eye Res 2014; 38:1-19 PMID:24135424; https://doi.org/10.1016/j.preteyeres.2013.08.004
- Bryant DM, Datta A, Rodriguez-Fraticelli AE, Peränen J, Martín-Belmonte F, Mostov KE. A molecular network for de novo generation of the apical surface and lumen. Nat Cell Biol 2010; 12:1035-45 PMID:20890297; https://doi.org/10.1038/ncb2106
- Horgan CP, Hanscom SR, Jolly RS, Futter CE, McCaffrey MW. Rab11-FIP3 links the Rab11 GTPase and cytoplasmic dynein to mediate transport to the endosomal-recycling compartment. J Cell Sci 2010; 123:181-91 PMID:20026645; https://doi.org/10.1242/jcs.052670
- Zhang Z, Zhang T, Wang S, Gong Z, Tang C, Chen J, Ding J. Molecular mechanism for Rabex-5 GEF activation by Rabaptin-5. Elife 2014; 3:e02687; PMID:24957337; https://doi.org/10.7554/eLife.02687
- Simon I, Zerial M, Goody RS. Kinetics of interaction of Rab5 and Rab7 with nucleotides and magnesium ions. J Biol Chem 1996; 271:20470-8 PMID:8702787; https://doi.org/10.1074/jbc.271.34.20470
- Ka lin S, Hirschmann DT, Buser DP, Spiess M. Rabaptin5 is recruited to endosomes by Rab4 and Rabex5 to regulate endosome maturation. J Cell Sci 2015; 128:4126-37 PMID:26430212; https://doi.org/10.1242/jcs.174664
- de Renzis S, Sönnichsen B, Zerial M. Divalent Rab effectors regulate the sub-compartmental organization and sorting of early endosomes. Nat Cell Biol 2002; 4:124-33 PMID:11788822; https://doi.org/10.1038/ncb744
- Deneka M, van der Sluijs P. “Rab”ing up endosomal membrane transport. Nat Cell Biol 2002; 4:E33-5; PMID:11835054; https://doi.org/10.1038/ncb0202-e33
- Navaroli DM, Bellvé KD, Standley C, Lifshitz LM, Cardia J, Lambright D, Leonard D, Fogarty KE, Corvera S. Rabenosyn-5 defines the fate of the transferrin receptor following clathrin-mediated endocytosis. Proc Natl Acad Sci USA 2012; 109:E471-80; PMID:22308388; https://doi.org/10.1073/pnas.1115495109
- Frittoli E, Palamidessi A, Marighetti P, Confalonieri S, Bianchi F, Malinverno C, Mazzarol G, Viale G, Martin-Padura I, Garré M, et al. A RAB5/RAB4 recycling circuitry induces a proteolytic invasive program and promotes tumor dissemination. J Cell Biol 2014; 206:307-28 PMID:25049275; https://doi.org/10.1083/jcb.201403127
- Kobayashi H, Etoh K, Ohbayashi N, Fukuda M. Rab35 promotes the recruitment of Rab8, Rab13 and Rab36 to recycling endosomes through MICAL-L1 during neurite outgrowth. Biology Open 2014; 3:803-14 PMID:25086062; https://doi.org/10.1242/bio.20148771
- Nagashima K, Torii S, Yi Z, Igarashi M, Okamoto K, Takeuchi T, Izumi T. Melanophilin directly links Rab27a and myosin Va through its distinct coiled-coil regions. FEBS Lett 2002; 517:233-8 PMID:12062444; https://doi.org/10.1016/S0014-5793(02)02634-0
- Van Gele M, Dynoodt P, Lambert J. Griscelli syndrome: a model system to study vesicular trafficking. Pigment Cell Melanoma Res 2009; 22:268-82 PMID:19243575; https://doi.org/10.1111/j.1755-148X.2009.00558.x
- Schafer JC, Baetz NW, Lapierre LA, McRae RE, Roland JT, Goldenring JR. Rab11-FIP2 interaction with MYO5B regulates movement of Rab11a-containing recycling vesicles. Traffic 2014; 15:292-308 PMID:24372966; https://doi.org/10.1111/tra.12146
- Weisz OA, Rodriguez-Boulan E. Apical trafficking in epithelial cells: signals, clusters and motors. 2009; 122:4253–66. Available from: http://jcs.biologists.org/cgi/doi/10.1242/jcs.032615
- Vogel GF, Klee KMC, Janecke AR, Müller T, Hess MW, Huber LA. Cargo-selective apical exocytosis in epithelial cells is conducted by Myo5B, Slp4a, Vamp7, and Syntaxin 3. J Cell Biol [Internet] 2015; 211:587–604. Available from: http://eutils.ncbi.nlm.nih.gov/entrez/eutils/elink.fcgi?dbfrom=pubmed&id=26553929&retmode=ref&cmd=prlinks; PMID:26553929; https://doi.org/10.1083/jcb.201506112
- Knowles BC, Roland JT, Krishnan M, Tyska MJ, Lapierre LA, Dickman PS, Goldenring JR, Shub MD. Myosin Vb uncoupling from RAB8A and RAB11A elicits microvillus inclusion disease. J Clin Invest 2014; 124:2947-62 PMID:24892806; https://doi.org/10.1172/JCI71651
- Thoeni CE, Vogel GF, Tancevski I, Geley S, Lechner S, Pfaller K, Hess MW, Müller T, Janecke AR, Avitzur Y, et al. Microvillus inclusion disease: Loss of myosin Vb disrupts intracellular traffic and cell polarity. Traffic 2013; 15:22-42 PMID:24138727; https://doi.org/10.1111/tra.12131
- Schneeberger K, Vogel GF, Teunissen H, van Ommen DD, Begthel H, Bouazzaoui El L, van Vugt AHM, Beekman JM, Klumperman J, Müller T, et al. An inducible mouse model for microvillus inclusion disease reveals a role for myosin Vb in apical and basolateral trafficking. Proc Natl Acad Sci USA 2015; 112:12408-13 PMID:26392529; https://doi.org/10.1073/pnas.1516672112
- Suh H-Y, Lee D-W, Lee K-H, Ku B, Choi S-J, Woo J-S, Kim Y-G, Oh B-H. Structural insights into the dual nucleotide exchange and GDI displacement activity of SidM/DrrA. EMBO J 2010; 29:496-504 PMID:19942850; https://doi.org/10.1038/emboj.2009.347
- Zhu Y, Hu L, Zhou Y, Yao Q, Liu L, Shao F. Structural mechanism of host Rab1 activation by the bifunctional Legionella type IV effector SidM/DrrA. Proc Natl Acad Sci USA 2010; 107:4699-704 PMID:20176951; https://doi.org/10.1073/pnas.0914231107
- Neunuebel MR, Mohammadi S, Jarnik M, Machner MP. Legionella pneumophila lida affects nucleotide binding and activity of the host GTPase Rab1. J Bacteriol 2012; 194:1389-400 PMID:22228731; https://doi.org/10.1128/JB.06306-11
- Hardiman CA, Roy CR. AMPylation is critical for Rab1 localization to vacuoles containing Legionella pneumophila. MBio 2014; 5:e01035-13; PMID:24520063; https://doi.org/10.1128/mBio.01035-13
- Cheng W, Yin K, Lu D, Li B, Zhu D, Chen Y, Zhang H, Xu S, Chai J, Gu L. Structural insights into a unique Legionella pneumophila effector LidA recognizing both GDP and GTP bound Rab1 in their active state. PLoS Pathog 2012; 8:e1002528; PMID:22416225; https://doi.org/10.1371/journal.ppat.1002528
- Mukherjee S, Liu X, Arasaki K, Mcdonough J, Galán JE, Roy CR. Modulation of Rab GTPase function by a protein phosphocholine transferase. Nature 2011; 477:103-6 PMID:21822290; https://doi.org/10.1038/nature10335
- Tan Y, Arnold RJ, Luo Z-Q. Legionella pneumophila regulates the small GTPase Rab1 activity by reversible phosphorylcholination. Proc Natl Acad Sci USA [Internet] 2011; 108:21212–7. Available from: http://www.ncbi.nlm.nih.gov/entrez/query.fcgi?db=pubmed&cmd=Retrieve&dopt=AbstractPlus&list_uids=22158903; PMID:22158903; https://doi.org/10.1073/pnas.1114023109
- Goody PR, Heller K, Oesterlin LK, Müller MP, Itzen A, Goody RS. Reversible phosphocholination of Rab proteins by Legionella pneumophila effector proteins. EMBO J 2012; 31:1774-84 PMID:22307087; https://doi.org/10.1038/emboj.2012.16
- Koch D, Rai A, Ali I, Bleimling N, Friese T, Brockmeyer A, Janning P, Goud B, Itzen A, Müller MP, et al. A pull-down procedure for the identification of unknown GEFs for small GTPases. Small GTPases 2016; 7:93-106 PMID:26918858; https://doi.org/10.1080/21541248.2016.1156803
- Gillingham AK, Sinka R, Torres IL, Lilley KS, Munro S. Toward a comprehensive map of the effectors of rab GTPases. Dev Cell 2014; 31:358-73 PMID:25453831; https://doi.org/10.1016/j.devcel.2014.10.007
- Lürick A, Gao J, Kuhlee A, Yavavli E, Langemeyer L, Perz A, Raunser S, Ungermann C. Multivalent Rab interactions determine tether-mediated membrane fusion. Mol Biol Cell 2016; 28(2):322-332; PMID:27852901; https://doi.org/10.1091/mbc.E16-11-0764
- Stroupe C, Brunger AT. Crystal structures of a Rab protein in its inactive and active conformations. J Mol Biol 2000; 304:585-98 PMID:11099382; https://doi.org/10.1006/jmbi.2000.4236
- Ashkenazy H, Abadi S, Martz E, Chay O, Mayrose I, Pupko T, Ben-Tal N. ConSurf 2016: An improved methodology to estimate and visualize evolutionary conservation in macromolecules. Nucleic Acids Res 2016; 44:W344-50; PMID:27166375; https://doi.org/10.1093/nar/gkw408