Abstract
This review explores the use of microalgae for nutrient removal in municipal wastewater treatment, considering recent improvements in the understanding of removal mechanisms and developments of both suspended and non-suspended systems. Nutrient removal is associated to both direct and indirect uptake, with the former associated to the biomass concentration and growth environment (reactor). Importantly, direct uptake is influenced by the Nitrogen:Phosphorus content in both the cells and the surrounding wastewater, with opposite trends observed for N and P. Comparison of suspended and non-suspended systems revealed that whilst all were capable of achieving high levels of nutrient removal, only non-suspended immobilized systems could do so with reduced hydraulic retention times of less than 1 day. As microalgae are photosynthetic organisms, the metabolic processes associated with nutrient assimilation are driven by light. Optimization of light delivery remains a key area of development with examples of improved mixing in suspended systems and the use of pulsating lights to enhance light utilization and reduce costs. Recent data provide increased confidence in the use of microalgae for nutrient removal in municipal wastewater treatment, enabling effluent discharges below 1 mg L−1 to be met whilst generating added value in terms of bioproducts for energy production or nutrient recovery. Ultimately, the review suggests that future research should focus on non-suspended systems and the determination of the added value potential. In so doing, it is predicted that microalgae systems will be significant in the delivery of the circular economy.
Introduction
The potential for remediation of inorganic nitrogen and phosphorus from wastewater by microalgae is well documented [Citation1–9] and considered an environmental approach to nutrient polishing.[Citation10,Citation11] In addition to enabling low nutrient discharges, a number of added benefits have been described: (a) sequestering of CO2 from the atmosphere during photosynthesis [Citation12]; (b) oxygenating the treated effluent [Citation13]; (c) unlike alternative biological treatment processes, a compulsory inorganic carbon source is unnecessary to optimize treatment [Citation8,Citation13] and (d) removal of trace organic micropollutants. Furthermore, following treatment, the algal biomass can be processed for the production of low-value products within human and animal nutrition, cosmetics and biofuels, including biomethane through anaerobic digestion of the residual biomass.[Citation14,Citation15]
Microalgae are ubiquitous to wastewater environments [Citation16] albeit at dilute concentrations, confirming the nutrient characteristics of such environments are suitable for growth [Citation17], with microalgae demonstrating the ability to remediate effluents at concentrations commonly encountered post-secondary treatment.[Citation18] Microalgae are therefore considered as prospective candidates for tertiary wastewater treatment.[Citation19–21]
The desired features for a microalgal solution for tertiary treatment include performance reaching the required level of remediation within a practical hydraulic retention time (HRT). The longest HRTs typically encountered in tertiary treatment are related to constructed wetlands extending up to 1 day.[Citation22] Current operation of microalgae treatment is most commonly achieved in high rate algal ponds (HRAP) with HRTs of 4–10 days. Accordingly, uptake is predominately reported in locations where land availability is not restrictive (e.g. USA [Citation23]) but represents a research challenge to broaden uptake.
The combination of microalgae being able to meet low nutrient discharges (e.g. sub 1.0 mg L−1 total phosphorus) and the generation of added value components (e.g. biomethane feedstock material) have resulted in refreshed consideration of the need and benefits of microalgae over traditional nutrient removal options (e.g. chemical dosing for phosphorus), which offer no added value. Illustration of this is seen in regard to phosphorus, where a range of new technologies being implemented to meet sub 1.0 mg L−1 discharges are all based on chemical dosing and clarification (e.g. BluePro, CoMag), resulting in an increase in coagulant use and residual sludge production. Additional issues arise at sites that previously did not incorporate chemical dosing such as small rural works. In such cases, the chemical-dosing-based options generate additional challenges due to the need for better infrastructure around transport (roads) and health and safety, including supply of potable water safety showers and chemical storage facilities.[Citation24] Accordingly, there is a need for microalgae treatment options that offer a real alternative to the chemical-dosing-based technologies beyond sites where use of HRAP is appropriate.[Citation25,Citation26] This has seen a growth in research around application to more nutrient-limited environments such as tertiary treatment [Citation19] coupled with new insights and trials of alternative technologies.[Citation27,Citation28] This review aims to appraise the new insights and technologies to consider the impact on the future potential for microalgae systems for tertiary treatment.
Overview of microalgal nutrient remediation mechanisms
Direct (biological) remediation of nitrogen and phosphate and microalgal species’ nitrogen:phosphorus composition
Nutrient remediation with microalgae occurs through one of two pathways (). Direct remediation is the most commonly discussed mechanism of remediation and is achieved through interconnected biochemical pathways for the uptake of the target nutrients into the biomass for storage,[Citation29,Citation30] or assimilation into nucleic acids and proteins for biomass growth [Citation23] ().
Figure 1. Schematic diagram of a microalgal cell summarising the biochemical pathways of nitrogen and phosphorus remediation, including indirect mechanisms (highlighted within a dashed box). © Represents co-transportation.
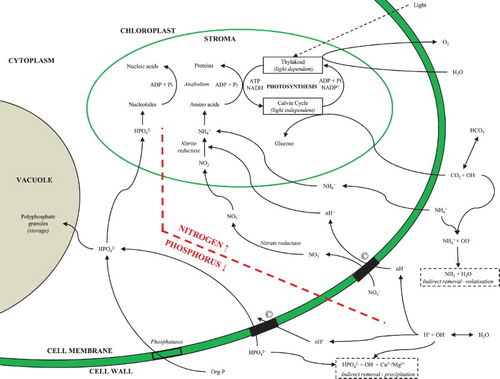
Inorganic nitrogen – that is, nitrite , nitrate
and ammonium
– are translocated across the cell membrane [Citation23] in the preference of
.[Citation31] These oxidized nitrogen species are subsequently reduced to
and assimilated into amino acids for the formation of proteins (), with
uptake preferred due to the reduced energy requirement necessary for reduction and assimilation.[Citation23] Accordingly, microalgae can be utilized for total nitrogen (TN) removal (nitrification and denitrification), with
assimilation observed following the near-complete exhaustion of
[Citation32] from the source wastewater.
Phosphate, in the preferred form of and
, is transported across the cell membrane via energized transport [Citation13,Citation23] and assimilated into nucleotides following phosphorylation for the synthesis of ribosomal RNA [Citation33] (). A nitrogen source is therefore required for the synthesis of proteins to enable the assimilation of phosphorus, with a limitation of either nutrient resulting in a low cell-protein content and reduced biomass growth.[Citation33] Furthermore, in high-phosphate environments, microalgae can consume excess phosphate through a luxury uptake pathway [Citation29,Citation34] for storage as an acid-insoluble polyphosphate granule [Citation29] () for future use in times when the external phosphate concentration may become limiting.
Nutrient uptake by microalgae depends on the associated concentration in the microalgae biomass such that phosphorus removal is consistently lower than nitrogen () due to a greater microalgal nitrogen biomass content.[Citation42] Freshwater microalgae biomass nitrogen:phosphorus (N:P) molar concentrations range between 8:1 and 45:1, indicating the importance of species selection when optimizing treatment.[Citation43] In addition, microalgae are known to adjust the N and P concentration in their biomass in relation to the levels in the surrounding medium.[Citation33,Citation44] For example, when cultured in mediums of varying N:P, internal N:P contents of 8.5–32 and 4.1–32 have been reported for the freshwater species Chlorella vulgaris and Scenedesmus obliquus.[Citation33,Citation45,Citation46]
Table 1. Ammonium and phosphorus removal by microalgal cultures for varying waste streams.
The remediation of nitrogen and phosphate is generally correlated to an increase in both the biomass volume [Citation17] and the internal nutrient content within the biomass.[Citation33,Citation47] Biomass productivity (growth) decreases with an increasing external N:P concentration as illustrated in the case of C. vulgaris, with a maximum growth rate of 2.97 g L−1 d−1 at N:P (mg mg−1) >10 demonstrated through a transition from N to P limitation.[Citation44] In relation to phosphorus, an inverse relationship between a species internal content and specific uptake rate is reported.[Citation30,Citation44] This results in reduced nutrient removal as illustrated with a culture of C. vulgaris, where phosphorus remediation decreased from >80% with an increasing phosphorus cellular content when treating wastewater with an N:P up to 20 mg mg−1 down to only 20% when treating a wastewater with a nutrient ratio of >50 N:P.[Citation44] Similarly, Ruiz-Martínez [Citation30] found an increased phosphate uptake rate of ∼3.3 mgPO4-P gTSS−1 h−1 in comparison to ∼0.7 mgPO4-P gTSS−1 h−1 for Scenedesmus sp. with internal P concentrations of ∼0.6% and 1% (w/w), respectively. An internal phosphate concentration of <1% characterizes growth within phosphorus-limited environments,[Citation48] whereas >1% is indicative of microalga luxuriously consuming phosphate for growth and storage for future use in conditions of limitation.[Citation29] Observations from these studies suggest that an increased specific uptake rate for phosphorus is a result of either a limiting external concentration or an initially reduced internal content, with both conditions representing times of stress when phosphorus assimilation is necessary for the continued metabolic processes and growth of a culture. The opposite has been demonstrated for nitrogen assimilation, with a cellular content of ∼0.02 mgN mgVSS−1 for C. vulgaris when remediating an influent profiled by an N:P (mg mg−1) of 30 in comparison to 0.2 mgN mgVSS−1 at an N:P of 80 [Citation44] corresponding with phosphorus limitation. Similarly, the nitrogen removal efficiency of Scenedesmus sp. has been reported to decrease significantly when the N:P ratio exceeds 12:1.[Citation17] Whilst no significant link has been observed between P removal and carbon concentrations, below a C:N of 10, nitrate uptake has been observed to be reduced in the case of Chlorella sp. [Citation50].
The ability of a range of microalgae to remove nutrients from wastewater and synthetic wastewater has been analysed extensively within laboratory trials. The majority of research to date has been conducted on unicellular chlorophyceae,[Citation49] in particular on the members from the Chlorella and Scenedesmus families (). Species from these families are largely used due to their dominance in freshwater environments,[Citation50] the ease with which they are cultured and reproduce [Citation20,Citation36] and their ability to efficiently remove nutrients.[Citation50] Recent comparisons of a range of algae have largely confirmed the suitability of Scenedesmus sp. for use in tertiary treatment applications.[Citation20]
However, unicellular algae are difficult to harvest, resulting in recent research into non-planktonic algae, especially filamentous species such as Oedogonium sp. and Tribonema sp.[Citation51,Citation52] For instance, Liu and Vyverman [Citation53] reported that for the filamentous algae trialled, Cladophora sp. was most efficient under low N:P ratio wastewater whilst Pseudanabaena sp. was better for removing nitrogen from high N:P ratio wastewater. In comparison to research on these identified species, fewer studies exist on other chlorophyceae as well as other taxa such as cyanobacteria and diatoms. The potential for nutrient removal by other chlorophyceae species in addition to diatoms and cyanobacteria are not as widely investigated, but are known to populate wastewater treatment works [Citation54,Citation55] and demonstrate beneficial characteristics. Cyanobacteria, for example, contain accessory pigments and an enhanced concentration of chlorophyll in comparison to chlorophyceae, enabling a more efficient use of available light.[Citation56] In addition, species isolated from colder climates, for example, Phormidium bohneri, have shown acceptable growth and nutrient removal rates at cooler temperatures, demonstrating removal rates between 2.4 and 19.9 mg L−1 d−1 for and 1.6 and 13.8 mg L−1 d−1 for total phosphorus (TP) within secondary effluent.[Citation40] In comparison, optimum nitrogen removal of 77.5 mg L−1 d−1 was observed for S. obliquus at 31°C with no treatment predicted below 8.8°C based on fitting the observed data to the cordinal temperature model with inflexion.[Citation30]
Mono-culture growth of a species is achieved commercially by operating at favourable loading rates, retention times and environmental parameters [Citation56] (e.g. for the growth of Chlorella, Spirulina and Dunaliella [Citation57]); or by the selective recycling of species.[Citation58] However, species control within a wastewater environment is challenging as microalgae are opportunistic [Citation59], and attempts to control the community have failed due to contamination from native algal species.[Citation60]
Indirect nitrogen (volitisation) and phosphate (precipitation) remediation
A by-product of direct remediation and growth is the alkalization of the localized environment through: (1) production of hydroxyl radicals (OH−) during photosynthetic consumption of inorganic carbon such as bicarbonate [Citation42,Citation36,Citation61] and (2) a net uptake of protons (H+) from the dissociation of H2O for co-transportation of
and
through the microalgal cell membrane [Citation61,Citation62] (). The modification of the physiochemical environment through pH [Citation42] facilitates the indirect removal method. In the case of
, at pH values greater than 7, there is an equilibrium shift within the kinetic equilibria for
and ammonia (NH3) towards the production of NH3 (gas) [Citation39], which is subsequently volatized and stripped from the solution. The mechanism of indirect removal of ammonium has been shown to contribute greatly to total NH4-N remediation, with removal percentages of 38–100% reported for the cyanobacteria P. bohneri [Citation63] and 53–82% for S. obliquus under varying temperatures and mixing regimes.[Citation39]
Unlike ammonium, phosphate cannot exist in a gaseous state and precipitates with metal ions (e.g. Ca, Mg and Fe) within the effluent , at elevated pH and high dissolved oxygen concentrations [Citation23,Citation29], with a removal efficiency of 16–63% reported for Monoraphidium species upon treating sterile-filtered wastewater.[Citation61] The indirect removal mechanisms are not specifically monitored in the operation of microalgal bioreactors in the vast majority of cases. However, increases from an influent pH ranging between 7 and 9.3 to a final effluent pH between 8.5 and 11.1 are documented during the operation of those bioreactors were the pH is uncontrolled (Supplementary information). Once pH increases beyond 10.5, phosphate precipitation decreases due to a switch towards calcium carbonate formation as a result of the relative change in precipitation kinetics between calcium and phosphate or carbonate.[Citation64]
Microalgal bioreactor configurations for wastewater nutrient remediation
The assimilation of nutrients for growth is facilitated by the process of photosynthesis, which is driven by the supply of inorganic carbon, light and temperature (). Inorganic carbon, however, is often regarded as non-limiting [Citation56,Citation63] within wastewater effluent and expressed indirectly as the chemical oxygen demand.[Citation56] It is the external factors of light and temperature, as opposed to the concentration of target nutrients, which have the greatest influence on growth and productivity [Citation63] and are considered the key design features in the operation of a microalgal bioreactor with studies focusing on light [Citation65–67]; temperature [Citation63,Citation68] in addition to species selection [Citation20,Citation53,Citation69] to optimize performance.
Various bioreactor designs are available to enhance growth and facilitate biomass removal following treatment, which include suspended and non-suspended systems [Citation11,Citation70], with sub-categories of either open to the environment or enclosed ().
Suspended cultures enable the microscopic algal cells to move freely within a body of water in dilute concentrations [Citation28] and are most commonly used in microalgal wastewater treatment.[Citation11] The biomass concentrations of suspended systems are reasonably low (<2 g L−1) () but can exceed 4.5 g L−1 in intensified systems when treating industrial sources.[Citation80] If not efficiently removed (harvested) post-treatment, the biomass has been reported to contribute to an increase in suspended solids content and 60–90% of the effluent biological oxygen demand.[Citation81]
Table 2. Summary of microalgal bioreactor designs, operating parameters and performance.
Harvesting is challenging and expensive and described as the defining factor of overall affordability [Citation28] representing 20–30% of the production cost.[Citation53] The microalgal cell surface is negatively charged,[Citation82] with cells repulsed from one another and maintained in suspension. Harvesting typically involves dosing a positively charged metal coagulant to neutralize the surface charge, allowing the cells to aggregate together, creating flocs.[Citation82] Subsequent removal of the flocs is through filtration, sedimentation, centrifugation or dissolved air flotation (DAF),[Citation83] with DAF taking advantage of the natural tendency of algae to float by raising the biomass to the surface, where it is skimmed off and recovered. Harvesting thorough centrifugation and DAF represent significant chemical and energy costs [Citation84,Citation15,Citation85] and require additional assets within the process flow-sheet representing further capital and operational expenditure.
Recommendations to overcome the challenges associated with harvesting suspended cultures include the use of species known to self-flocculate, thereby aiding in removal by sedimentation or flotation,[Citation5] or alternatively through the selection and growth of a non-suspended/filamentous culture.[Citation53] Filamentous species naturally attach together in addition to other particles (i.e. suspended material and other biological entities), forming a biofilm layer on a surface interface.[Citation55] This interface can be the surface of the reactor (i.e. floor, walls and baffles), or intentionally submerged substrates (e.g. polyurethane and polystyrene foam [Citation78,Citation86]) can be used to increase the available attachment surface area. Harvesting is achieved through physically scrapping the attachment surface to remove the biofilm, thereby eliminating the costs associated with harvesting suspended biomass.[Citation87] Non-suspended systems can be further categorized into matrix-immobilization with microalgal biomass encapsulated in a hydrophilic polymer, whilst reducing the challenges associated with harvesting increases issues related to cellular access to CO2, nutrients and photons.[Citation88] Such systems are considerably less established than the suspended systems and questions remain around their suitability and affordability for municipal wastewater treatment.
The suspended and non-suspended bioreactors are then further categorized into open and closed systems. Open systems rely on the external environmental conditions to facilitate growth, characterized by the use of solar irradiance with sunlight hours and intensity affecting biomass productivity. Open systems are further influenced by external conditions, including temperature, rainfall (instigating culture dilution) and contamination by opportunistic species resulting in variability in annual performance. Alternatively, closed systems contain the biomass within the reactor, thereby minimising the opportunity for contamination [Citation89] and supporting the culture of a mono-community through separation of the biomass from a potentially growth inhibiting environment.[Citation90] An enclosed system offers greater control of the parameters to optimize growth [Citation70,Citation89] (e.g. irradiance, temperature, evapotranspiration, O2, CO2 and pH) and encourages increased specific growth, but requires greater infrastructure and operational costs, limiting their scalability.
The choice of bioreactor is evaluated upon performance at an economically accepted cost,[Citation57] with examples including (but not limited to) HRAP, photobioreactors (PBRs), attached microalgal biofilms and matrix-immobilization ().
High rate algal pond
A HRAP is a raceway-configured open pond mixed via a paddle wheel to circulate the algal culture and prevent settlement.[Citation71,Citation91] Sunlight is the primary method of irradiation and as such, culture depths of 20–60 cm are typical [Citation57,Citation71,Citation72] to enable optimal light penetration and maximize growth. A HRAP supports a symbiotic community of microalgae and bacteria for the assimilation of nutrients and organic matter [Citation58], supporting dilute microalgal biomass concentrations of up to 1 g(DW) L−1.[Citation11] Operational retention times of 4–10 days [Citation72] are required to enable sufficient contact time with the biomass to achieve the required level of remediation (), resulting in large footprints.[Citation27] Of the bioreactors available, HRAPs have received the most attention [Citation11] and can be found operational at full scale with a demonstration plant located in New Zealand with individual pond footprints of 1.25 ha.[Citation92,Citation93] Further larger demonstration plants will be constructed in California, New Mexico, Hawaii and Florida with the primary focus on biomass production for biofuels.[Citation23]
Photobioreactor
A PBR is an example of a closed, suspended system and are available in various configurations, including horizontal or vertical tubular photobioreactor (TPBR) or flat panel reactors.[Citation57,Citation89,Citation94,Citation95] A PBR encloses the culture in a series of narrow tubes (e.g. <4 cm diameter [Citation27]) or panels illuminated by sunlight and/or artificial sources.[Citation89] Enclosing the culture enables a greater control of growth conditions (i.e. light, CO2, O2 and pH [Citation11]) and permits the growth of a target species through optimized growth parameters, facilitating an increased biomass concentration in comparison to a HRAP of up to 2.0 g L−1 () when cultured/remediating domestic wastewater effluents. The culture is circulated through the reactor by pumping and degassing/bubbling processes (additionally releasing excess O2 produced through photosynthesis). As a consequence of the sophistication of control, PBRs are expensive to install and operate [Citation27] and are typically only employed in wastewater treatment as a plentiful source of low-cost culture medium for growth of a species for the return of a high-value product [Citation27,Citation96] to cover the cost of operation. High biomass production PBRs of up to 4000 L in capacity are operational and utilized for the cultivation of an inoculum species for HRAPs with the ultimate goal of biofuel production.[Citation23]
Microalgal biofilms
Two types of microalgal biofilm processes exist; these include the use of an inclined floway (aka algal turf scrubber) with biofilm attachment to a surface [Citation75,Citation97] or submersion of a substrate to support biofilm growth and development [Citation76,Citation98,Citation99] with practical examples including rotating algal biofilm reactors (RABR) [Citation77] (). Biomass communities are heterogenous and multi-layered [Citation55,Citation100] and change seasonally [Citation91] with reported biomass productivity of up to 60.9 g m−2 d−1 [Citation75] and demonstrating enhanced metabolic activity.[Citation101] Floway systems have been operated at full scale in Florida and California [Citation59,Citation102] with footprints up to 1012 m2 treating a flow of 109–1336 m3 d−1.
Matrix-immobilisation
Matrix-immobilization is a variant of the attachment theme of reactors through the entrapment of living microalgae cells within a natural or artificial resin.[Citation103] These resins are hydrophilic in nature with small pores to enable the diffusion of wastewater to the entrapped microalgal cells.[Citation104] Immobilization enables intensification of a biomass concentration greater than a suspended bioreactor with concentrations up to 3.3 g L−1 reported (). The resin used to immobilize the microalgal biomass can provide additional remediation with the natural resin alginate found to contribute approximately 5% remediation efficiency of ammonium from a synthetic wastewater [Citation37] through a chemical bond between the ammonium ions and the carboxyl groups of the resin.[Citation37,Citation105] This bond not only removes the ammonium from the source water but concentrates the nutrient for assimilation by the entrapped microalgae cells.[Citation37] Further benefits of immobilising a microalgal culture includes the creation of a barrier around the selected species which prevents penetration by other organisms, which could inhibit productivity or outcompete the selected species [Citation88,Citation106]; and following treatment, the biomass can be harvested through the low-costing option of gravity settlement, eliminating chemical and energy costs associated with suspended systems. However, reduced space for mobility within the matrix leads to high shear stresses, with the matrix imposing additional hindrance to photon accessibility being an area of concern along with the cost of the polymeric matrix when considered at full scale.[Citation91,Citation28] Of the microalgal reactors available, the immobilization technology for nutrient remediation is within its infancy with the majority of research and knowledge gained to date through lab-scale activities with bioreactors of up to 5 L in volume [Citation105] (, see Table 6 at online supplemental data at 10.1080/21622515.2015.1105308).
Influence of operational parameters and bioreactor design on remediation performance
Influent nutrient concentration and treatment period
The final effluent concentration and treatment period are key criteria when assessing the performance of microalgal bioreactors for wastewater nutrient remediation. European regulations within the Urban Wastewater Treatment Directive require a final effluent concentration prior to discharge of 15 or 10 mg L−1 TN and 2 or 1 mg L−1 TP for works treating a population equivalence of either 10–100 k or >100 k, respectively,[Citation107] with further site specific reductions in phosphorus to ∼0.1 mg L−1 proposed with the onset of the water framework directive in 2015. Furthermore, the inclusion of an additional asset within a flow sheet to satisfy the required discharge concentrations must complement upstream processes to enable a constant output and flow. Microalgal bioreactors have been analysed for the treatment of a variety of wastewater streams, including primary and secondary domestic effluent, dairy manure wastewater, agricultural run-off and centrate (Supplementary information) with remediation data available for a wide range of influent concentration from 3.3 to 309 mg L−1 for ammonium and 0.04 to 770 mg L−1 for phosphorus ().
Figure 3. Influent concentration versus effluent concentration for (a) ammonium and (b) phosphate; and treatment period versus remediation efficiency for (c) ammonium and (d) phosphate for all bioreactors. HRAP (▪), biofilms (◊), matrix-immobilization (▴), PBR (○) and parity line (- -).
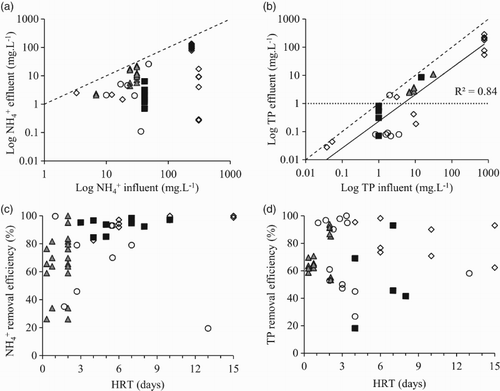
When comparing microalgal bioreactor options for ammonium remediation, the influent and effluent concentrations show no clear relationship ((a)) with effluent concentrations ranging from 0.11 to 140.9 mg L−1. Those showing enhanced remediation performance include a TPBR with a 99.7% removal efficiency and effluent concentration of 0.11 mg L−1,[Citation73] in addition to a polystyrene submersion system achieving 99.9% removal and an effluent concentration of 0.3 mg L−1 [Citation98] ((a)). These systems were either inoculated or naturally dominated by species of chlorophyceae well known for their nutrient remediation abilities, namely Chlorella sp. (TPBR) and Scenedesmus sp. (biofilm). Both systems demonstrated high biomass yields and productivities with a specific growth rate of 0.39 d−1 for Scenedesmus sp. and an increase in biomass concentration from 0.4 to 2 g L−1 (approximate maximum biomass concentration reported for a TPBR configuration [Citation11]) and Chlorella sp. with a biomass concentration of 30–35 g(dry mass) m−2 equivalent to a productivity of 2–4 g m−2 d−1.[Citation98]
Those bioreactors which did not perform as well belonged to the biofilm category of reactors with an algal turf scrubber demonstrating a 24.2% removal and effluent concentration of 2.5 mg L−1 [Citation97] and a PBR containing rough surfaces to facilitate biofilm attachment with a removal efficiency of 45.8% and an effluent concentration of 26.0 mg L−1.[Citation108] Although the algal turf scrubber reported an extremely high yearly biomass productivity of 35 g m−2 d−1, the biofilm contained a significant proportion of bacterial matter, particulates and cyanobacteria with the chlorophyceae Chlorella sp. and Scenedesmus sp. reported as only ‘present’ or ‘few’.[Citation97] Findings from these studies demonstrate the importance of species selection in addition to biomass concentration for the enhanced remediation of ammonium. Performance could therefore be improved by an increased biomass concentration or longer contact time with the available biomass to facilitate remediation through the direct mechanisms.
Whereas for phosphorus, a reasonable log–log relationship (r2 = 0.84) is observed for all bioreactors ((b)) with a lower influent concentration resulting in lower effluent concentration despite vast differences in operating parameters, including biomass concentration, treatment time and irradiance. This relationship suggests that unlike ammonium, mechanisms other than direct remediation are primarily responsible for the remediation of phosphate with treated effluent concentrations of <1 mg L−1 possible at influent concentrations <10 mg L−1 ((b)) providing there is an adequate supply of nitrogen.[Citation33]
In terms of treatment period, performance data for NH4-N removal exhibit a more defined relationship with performance efficiency and treatment period, in comparison to those for PO4-P ((c),(d)). The enclosed and more-intensive reactors remediate >80% NH4-N within less than 2 days ((c)) with HRTs >3 days required by HRAPs through the necessary increased contact period with the reduced biomass concentration. The biofilm solutions are characterized by a high biomass concentration but require a HRT >10 days for a > 90% NH4-N removal[Citation98]; however, this is a necessary design feature as increasing the flow velocity (hence reducing the HRT) creates increased shear stress, reducing biofilm colonization [Citation109] with impacts on remediation performance and increased suspended solids within the treated effluent.
In comparison, PBRs and matrix-immobilization can achieve >99% NH4-N remediation efficiency within HRT <2 days through an increased biomass concentration and protection from biomass washout with increased flows. For example, a matrix-immobilized system with an algal concentration of 106 cells bead−1 and 11.7 beads mL−1 was shown to remediate 100% NH4-N within 24 h,[Citation105] in addition to a TPBR with a 2 g L−1 biomass concentrations achieving a 99.7% NH4-N remediation.[Citation73] The remediation of ammonium is a function of biomass concentration and contact time, with those bioreactors with a dilute biomass concentration requiring a greater treatment period in comparison to bioreactors with high biomass concentrations, with biofilms an exception through operational limits.
A relationship between HRT and TP removal efficiency is not as clear as NH4-N ((d)) further supporting previous assumptions that an alternative mechanism other than direct remediation significantly contributes to the removal of phosphate. Phosphorus removal efficiencies are generally <69% for HRAPs with removal of approximately 40% seen in the majority of studies (see Table 3 at online supplemental data at 10.1080/21622515.2015.1105308). The cases where >90% removal are observed involve the modification of parameters to enhance the performance of a HRAP for phosphorus removal. For example, a 99% removal efficiency and a residual of 0.07 mg L−1 (see Table 3 at online supplemental data at doi:10.1080/21622515.2015.1105308) are achieved through the addition of lime (CaO) to promote autoflocculation and the precipitation of phosphorus (equivalent to the indirect mechanism),[Citation42] with a consensus that the role of indirect removal plays a more significant role than that of direct in HRAPs systems.[Citation71,Citation110]
Light, temperature and biomass productivity
As microalgae are photosynthetic organisms, metabolic processes associated with nutrient assimilation through growth are driven by light, [Citation90,Citation111] with light described as a key parameter of microalgal reactors.[Citation89,Citation90,Citation111] The required light intensity for optimal growth is species specific with an example range of 150–400 µmol m−2 s−1 reported for Scenedesmus sp.[Citation112,Citation113] Light intensities below a species threshold range are associated with a reduction in biomass productivity [Citation112] and can be generated through light limitation as a result of high-density microalgal cultures creating self-shading [Citation114] and/or light attenuation and reduction with an increasing transmittance pathway.[Citation114] Intensities beyond a species’ preferred range results in oxidative damage through photoinhibition associated with a reduction in biomass productivity with the dissipation of the excess photons into heat.[Citation115] To illustrate, Di Termini et al. [Citation73] observed a specific growth rate of 0.39 d−1 and a remediation efficiency of >98% for NH4-N and PO4-P for an autochthonous culture of Scenedesmus sp. when grown within an indoor TPBR with a constant light intensity of 200 µmol m−2 s−1 in comparison to a reduced growth rate of 0.02 d−1 and <80% NH4-N and PO4-P removal efficiency within an outdoor TPBR with a variable light intensity reaching a daylight maximum of 1300 µmol m−2 s−1.
As a consequence of biomass concentration, incident light intensity and culture depth (hence light transmittance depth), multiple ‘light zones’ are simultaneously evident within microalgal bioreactors.[Citation116] These zones can be described as light inhibited, saturated, limited and no light with the zones determined by the increasing depth from the culture surface, with a changing profile as a consequence of the incident light intensity and biomass concentration [Citation117] estimated through the Beer–Lambert law.[Citation90,Citation118] The challenge of microalgal bioreactors is to maintain the culture within the light saturating zone to enable optimal productivity and the associated direct remediation of the target nutrients. This can be achieved through (1) reducing the light transmittance pathway (short depths),[Citation27] (2) increasing culture circulation through mixing to ensure the microalgal cells move within the saturation zone [Citation93] and (3) maximising the surface to volume ratio [Citation11,Citation111,Citation114] to ensure sufficient light reaches the culture surface.
Outdoor systems are typically exposed to variable levels of light intensities through seasonal (and daily) changes in the available solar radiation. Intensities during the summer months of >1200 µmol m−2 s−1 in comparison to 170–685 µmol m−2 s−1 are reported in the winter months (see Table 3 at online supplemental data at doi:10.1080/21622515.2015.1105308). However, only 50% of the radiation provided by sunlight is available to the microalgae for use as photosynthetically active radiation (PAR) (400–700 nm) [Citation119] with open systems demonstrating poor photosynthetic efficiency in the conversion of solar energy into chemical energy of approximately 1.5%.[Citation120] The variabilities in light intensities are reflected in fluctuating biomass productivities with ranges between 4.4 and 11.5 g m−2 d−1 observed in a 5 ha demonstration HRAP plant with removal efficiencies for NH4-N and dissolved reactive phosphorus (DRP) between approximately 40–80% and 10–50%, respectively, mirroring the pattern in seasonal biomass productivity.[Citation92]
Artificially lit reactors are employed to overcome the variability in biomass productivities and the associated treatment profiles, with generally lower intensities in the range of ∼200–400 µmol m−2 s−1 reported for commercial PBRs.[Citation115] Exposure to lower intensities are possible through design optimization (e.g. surface to volume ratio) to enable effective use of the provided light with increased photosynthetic efficiencies of 3–5% for PBRs [Citation120] and/or the selection of a light source with a specific wavelength within the PAR to enable a more efficient use of the provided light, particularly as light is supplied at an operational cost. For instance, within PAR the microalgal chlorophyll molecules absorb light more efficiently within the blue (∼400 nm) and red (∼600–700 nm) region of the spectrum, with exposure to these wavelengths improving the photosynthetic efficiency and enhancing biochemical processes aligned to nitrogen and phosphorus remediation. For example, growth under a blue light regime is associated with increased phosphorus remediation through the activation of protein synthesis,[Citation121] demonstrated by a culture of Scenedesmus sp. with a 45% increase in removal rate under a blue light regime of 1.8 mg L−1 d−1 in comparison to 1 mg L−1 d−1 when grown under white light (400–700 nm).[Citation65] Furthermore, red light is known to enhance microalgal growth rate, with a 38% increase in the specific growth rate of a culture of Spirulina platensis in comparison to growth of the same species within white light.[Citation122]
The use of constant artificial light can represent a significant proportion of the total operational costs. Strategies are employed to improve the efficiency of artificial light which can be reflected within these costs and include (as discussed) the selection of an appropriate intensity to minimize wasted photons and the application of a light source with a suitable wavelength (i.e. LEDs) to eliminate energy use on unutilized wavelengths.[Citation123] However, the antenna structure of the microalgal light-harvesting complex is unable to absorb all the photons provided under constant light [Citation124], offering a further option of cost reduction and increased photosynthetic efficiency through reduced photoperiods and flashing/pulsating light regimes. For instance, under a flashing light regime of 37 kHz, the cell concentration of a culture of C. vulgaris was 20% greater than that of the same species grown under a constant light regime.[Citation124]
Overall, artificial lighting offers a variety of options for increasing biomass productivity and associated remediation of nutrients through lighting regimes, which cannot be benefitted from within open systems. Advances made within LED industry resulting in increased bulb life, associated energy savings and predicted reduction in unit cost over time [Citation125] make the use of artificial lighting a more attractive option for intensifying the remediation performance of microalgal reactors.
Microalgae exhibit a similar relationship to temperature as light, profiled by an increase in biomass productivity (and associated nutrient remediation) with increasing temperature [Citation126] until reaching a critical temperature, beyond which has a negative effect on growth. For instance, Martinez et al. [Citation68] documented an increase in specific growth rate of 0.69 d−1–1.10 d−1 for Scenedesmus sp. grown within secondary wastewater effluent with an increasing temperature from 20°C to 30°C coupled with >90% remediation of nitrogen and phosphorus. At 35°C, the specific growth rate decreased to 0.46 d−1 with a remediation efficiency of 79% and 54% for nitrogen and phosphorus, respectively. A temperature range between 15 and 30°C [Citation70,Citation126] are believed optimal for microalgal bioreactors, with maximum critical temperatures being species-specific, providing the external nutrient concentration and light provision are not limiting.[Citation126]
Maintenance of a constant temperature is challenging within open reactors [Citation126] with seasonal variations from 7.2°C to 25°C documented (see Table 3 at online supplemental data at doi:10.1080/21622515.2015.1105308) and extreme lows of 5°C and highs >30°C reported for HRAPs.[Citation42] Application of open systems are therefore favoured within locations with suitable annual climates to facilitate biomass productivity and achieve the required level of remediation throughout the year; for example, a floway periphyton scrubber located in the Florida Everglades with an daily mean air temperature of 19°C corresponding to a water temperature ranging from 18.1°C to 27.2°C.[Citation59] Furthermore, microalgae within low-temperature environments are more susceptible to photoinhibition, which can constrain the use of open reactors in countries with a cold climate,[Citation70] particularly with winter light intensities of up to 600 µmol m−2 s−1 reported.[Citation71] Temperature maintenance in closed reactors (and systems located indoors) is easier to control with documented temperatures of 20°C–30°C (see Tables 4–6 at online supplemental data at 10.1080/21622515.2015.1105308), corresponding to the range of temperatures associated with enhanced growth and nutrient remediation and more suited to locations with a cooler annual climate.
Conclusions and key remaining challenges
Algal treatment of wastewater, realized through a combination of direct uptake and indirect removal associated with elevated pH, provides a potential alternative to traditional tertiary treatment options for nutrient removal. Recent advancement in the understanding of both the mechanisms by which algae remediate nutrients in wastewater and specifically non-suspended algae treatment systems attests to the suggestions outlined by Hoffmann [Citation91]. Accordingly, the ability of algal-based wastewater treatment to meet future challenges can be viewed with greater confidence. The most pressing illustration of which is associated with compliance to emerging sub 1 mg L−1 phosphorus discharge standards. Further, developments in non-suspended systems have significantly reduced the required HRT of such systems to mirror existing passive tertiary treatment technologies. Consequently, consideration of the use of microalgal treatment can more reliably extend to sites where previously the lack of availability of sufficient inexpensive land was seen as a barrier to uptake of HRAP.
Future research will likely focus on the remaining challenges that require resolution before widespread use of algae can be realized. The costs associated with either harvesting of suspended systems, irradiance of closed systems and/or the chemicals associated with either harvesting and matrix- immobilization require better understanding and optimization to truly established the relative merit of microalgal systems compared to alternative tertiary treatment systems. As part of that better refinement is required with respect to the understanding of the added value algae systems can offer (in terms of associated bioenergy production, biofuels and bioproducts). This will become increasingly important in positioning microalgae treatment options as part of the delivery of the circular economy, which is expected to increasingly shape future investment consideration. Furthermore, the associated removal of hazardous chemicals and the ability for total nutrient removal need exploring in detail so that they can be properly valued. In addition, technical challenges remain associated with intensification and seasonal stability (HRAP), scalability and light utilization (PBRs and non-suspended) and selection of better strains/mixtures to match the target wastewater and maximize biomass growth and by-product yields (all systems). Whilst development and increased uptake of all reactors types should be expected, it is perhaps in relation to non-suspended systems that the greatest advancements can be anticipated. Increasing demonstration of non-suspended systems will better enable appropriate comparison to be made with HRAP and PBRs and practical optimization achieved. Ultimately this will enable the potential for such systems to be considered in places where HRAPs are either not practical or desirable such as small wastewater treatment works with limited land availability.
Supplemental data
Supplemental data for this article can be accessed at doi:10.1080/21622515.2015.1105308.
Supplemental_Data.zip
Download Zip (81.2 KB)Disclosure statement
No potential conflict of interest was reported by the authors.
ORCID
Rachel Whitton http://orcid.org/0000-0001-9378-0859
Francesco Ometto http://orcid.org/0000-0002-6989-6197
Marc Pidou http://orcid.org/0000-0003-3031-9584
Peter Jarvis http://orcid.org/0000-0003-2740-6740
Raffaella Villa http://orcid.org/0000-0001-6646-894X
Bruce Jefferson http://orcid.org/0000-0003-4320-628X
Additional information
Funding
References
- Oswald WJ, Gotaas HB. Photosynthesis in sewage treatment. Trans Am Soc Civ Eng. 1957;122:73–105.
- Bogan R, Albertson O, Pluntze J. Use of algae in removing phosphorus from sewage. J Sanit Eng Div ASCE. 1960;86(5):1–20.
- Gates W, Borchardt J. Nitrogen and phosphorus extraction from domestic wastewater treatment plant effluents by controlled algal culture. J Water Pollut Control Fed. 1964;36:443–462.
- Doran MD, Boyle WC. Phosphorus removal by activated algae. Water Res. 1979;13(8):805–812. doi: 10.1016/0043-1354(79)90246-X
- Hashimoto S, Furukawa K. Nutrient removal from secondary effluent by filamentous algae. J Ferment Bioeng. 1989;67(1):62–69. doi: 10.1016/0922-338X(89)90088-3
- Davis LS, Hoffmann JP, Cook PW. Production and nutrient accumulation by periphyton in a wastewater treatment facility. J Phycol. 1990;26(4):617–623. doi: 10.1111/j.0022-3646.1990.00617.x
- Jiménez-Pérez MV, Sánchez-Castillo P, Romera O, Fernández-Moreno D, Pérez-Martínez C. Growth and nutrient removal in free and immobilized planktonic green algae isolated from pig manure. Enzyme Microb Technol. 2004;34(5):392–398. doi: 10.1016/j.enzmictec.2003.07.010
- Boelee NC, Temmink H, Janssen M, Buisman CJN, Wijffels RH. Nitrogen and phosphorus removal from municipal wastewater effluent using microalgal biofilms. Water Res. 2011;45(18):5925–5933. doi: 10.1016/j.watres.2011.08.044
- Abinandan S, Shanthakumar S. Challenges and opportunities in application of microalgae (Chlorophyta) for wastewater treatment: a review. Renew Sust Energ Rev. 2015;52:123–132. doi: 10.1016/j.rser.2015.07.086
- Shi J, Podola B, Melkonian M. Removal of nitrogen and phosphorus from wastewater using microalgae immobilized on twin layers: an experimental study. J Appl Phycol. 2007;19(5):417–423. doi: 10.1007/s10811-006-9148-1
- Christenson L, Sims R. Production and harvesting of microalgae for wastewater treatment, biofuels, and bioproducts. Biotechnol Adv. 2011;29(6):686–702. doi: 10.1016/j.biotechadv.2011.05.015
- Oswald WJ, Golueke CG. Biological transformation of solar energy. Adv Appl Microbiol Adv Appl Microbiol. 1960;2:223–262. doi: 10.1016/S0065-2164(08)70127-8
- Silva NFP, Gonçalves AL, Moreira FC, et al. Towards sustainable microalgal biomass production by phycoremediation of a synthetic wastewater: a kinetic study. Algal Res. 2015;11:350–358. doi: 10.1016/j.algal.2015.07.014
- Spolaore P, Joannis-Cassan C, Duran E, Isambert A. Commercial applications of microalgae. J Biosci Bioeng. 2006;101(2):87–96. doi: 10.1263/jbb.101.87
- Ometto F, Pozza C, Whitton R, et al. The impacts of replacing air bubbles with microspheres for the clarification of algae from low cell-density culture. Water Res. 2014;53:168–179. doi: 10.1016/j.watres.2014.01.012
- Davis LS, Hoffmann JP, Cook PW. Seasonal succession of algal periphyton from a wastewater treatment facility. J Phycol. 1990b;26(4):611–617. doi: 10.1111/j.0022-3646.1990.00611.x
- Xin L, Hong-ying H, Ke G, Ying-xue S. Effects of different nitrogen and phosphorus concentrations on the growth, nutrient uptake, and lipid accumulation of a freshwater microalga Scenedesmus sp. Bioresour Technol. 2010;101(14):5494–5500. doi: 10.1016/j.biortech.2010.02.016
- Judd S, van den Broeke LJ, Shurair M, Kuti Y, Znad H. Algal remediation of CO2 and nutrient discharges: a review. Water Res. 2015;87:356–366. doi: 10.1016/j.watres.2015.08.021
- Sukačová K, Trtílek M, Rataj T. Phosphorus removal using a microalgal biofilm in a new biofilm photobioreactor for tertiary wastewater treatment. Water Res. 2015;71:55–63. doi: 10.1016/j.watres.2014.12.049
- Gómez-Serrano C, Morales-Amaral M, Acién F, Escudero R, Fernández-Sevilla J, Molina-Grima E. Utilization of secondary-treated wastewater for the production of freshwater microalgae. Appl Microbiol Biotechnol. 2015;99(16):6931–6944. doi: 10.1007/s00253-015-6694-y
- Selvaratnam T, Pegallapati A, Montelya F, et al. Feasibility of algal systems for sustainable wastewater treatment. Renew Energ. 2015;82:71–76. doi: 10.1016/j.renene.2014.07.061
- Butterworth E, Dotro G, Jones M, et al. Effect of artificial aeration on tertiary nitrification in a full-scale subsurface horizontal flow constructed wetland. Ecol Eng. 2013;54:236–244. doi: 10.1016/j.ecoleng.2013.01.034
- Cai T, Park SY, Li Y. Nutrient recovery from wastewater streams by microalgae: status and prospects. Renew Sust Energ Rev. 2013;19:360–369. doi: 10.1016/j.rser.2012.11.030
- Germain-Cripps E. Low energy, low maintenance phosphorus removal using constructed wetlands. 4th Conference on low energy wastewater treatment systems; 2015 Sep 24; Cranfield; 2015.
- Vale P. Assessing technologies to meet very low phosphorus limits. 4th Conference on low energy wastewater treatment systems; 2015 Sep 24; Cranfield; 2015.
- Jefferson B. Making algae reactors feasible for wastewater treatment in the UK. 4th Conference on low energy wastewater treatment systems; 2015 Sep 24; Cranfield; 2015.
- Gupta PL, Lee S, Choi H. A mini review: photobioreactors for large scale algal cultivation. World J Microbiol Biotechnol. 2015;31(9):1409–1417. doi: 10.1007/s11274-015-1892-4
- Zeng X, Guo X, Su G, et al. Bioprocess considerations for microalgal-based wastewater treatment and biomass production. Renew Sust Energ Rev. 2015;42:1385–1392. doi: 10.1016/j.rser.2014.11.033
- Powell N, Shilton AN, Pratt S, Chisti Y. Factors influencing luxury uptake of phosphorus by microalgae in waste stabilization ponds. Environ Sci Technol. 2008;42(16):5958–5962. doi: 10.1021/es703118s
- Ruiz-Martínez A, Serralta J, Romero I, Seco A, Ferrer J. Effect of intracellular P content on phosphate removal in Scenedesmus sp. Experimental study and kinetic expression. Bioresour Technol. 2015;175:325–332. doi: 10.1016/j.biortech.2014.10.081
- Lau PS, Tam NFY, Wong YS. Effect of algal density on nutrient removal from primary settled wastewater. Environ Pollut. 1995;89(1):59–66. doi: 10.1016/0269-7491(94)00044-E
- Maestrini SY, Robert J, Leftley JW, Collos Y. Ammonium thresholds for simultaneous uptake of ammonium and nitrate by oyster-pond algae. J Exp Mar Biol Ecol. 1986;102(1):75–98. doi: 10.1016/0022-0981(86)90127-9
- Beuckels A, Smolders E, Muylaert K. Nitrogen availability influences phosphorus removal in microalgae-based wastewater treatment. Water Res. 2015;77:98–106. doi: 10.1016/j.watres.2015.03.018
- Eixler S, Karsten U, Selig U. Phosphorus storage in Chlorella vulgaris (Trebouxiophyceae, Chlorophyta) cells and its dependence on phosphate supply. Phycologia. 2006;45(1):53–60. doi: 10.2216/04-79.1
- Sawayama S, Minowa T, Dote Y, Yokoyama S. Growth of the hydrocarbon-rich microalga Botryococcus braunii in secondarily treated sewage. Appl Microbiol Biotechnol. 1992; l. 38(1):135–138.
- Kim J, Lingaraju BP, Rheaume R, Lee J, Siddiqui KF. Removal of ammonia from wastewater effluent by Chlorella Vulgaris. Tsinghua Sci Technol. 2010;5(4):391–396. doi: 10.1016/S1007-0214(10)70078-X
- Ruiz-Marin A, Mendoza-Espinosa LG, Stephenson T. Growth and nutrient removal in free and immobilized green algae in batch and semi-continuous cultures treating real wastewater. Bioresour Technol. 2010;101(1):58–64. doi: 10.1016/j.biortech.2009.02.076
- González LE, Cañizares RO, Baena S. Efficiency of ammonia and phosphorus removal from a colombian agroindustrial wastewater by the microalgae Chlorella vulgaris and Scenedesmus dimorphus. Bioresour Technol. 1997;60(3):259–262. doi: 10.1016/S0960-8524(97)00029-1
- Martínez ME, Sánchez S, Jiménez JM, El Yousfi F, Muñoz L. Nitrogen and phosphorus removal from urban wastewater by the microalga Scenedesmus obliquus. Bioresour Technol. 2000;73(3):263–272. doi: 10.1016/S0960-8524(99)00121-2
- Laliberté G, Lessard P, de la Noüe J, Sylvestre S. Effect of phosphorus addition on nutrient removal from wastewater with the cyanobacterium Phormidium bohneri. Bioresour Technol. 1997;59(2–3):227–233. doi: 10.1016/S0960-8524(96)00144-7
- Thimijan RW, Heins RD. Photometric, radiometric, and quantum light units of measure: a review of procedures for interconversion. HortScience. 1983;18(6):818–822.
- Nurdogan Y, Oswald WJ. Enhanced nutrient removal in high-rate ponds. Water Sci Technol. 1995;31(12):33–43. doi: 10.1016/0273-1223(95)00490-E
- Hecky RE, Campbell P, Hendzel LL. The stoichiometry of carbon, nitrogen, and phosphorus in particulate matter of lakes and oceans. Limnol Oceanogr. 1993;38(4):709–724. doi: 10.4319/lo.1993.38.4.0709
- Choi HJ, Lee SM. Effect of the N/P ratio on biomass productivity and nutrient removal from municipal wastewater. Bioprocess Eng. 2015;38(4):761–766.
- Rhee G. Phosphate uptake under nitrate limitation by Scenedesmus sp. and its ecological implications. J Phycol. 1974;10(4):470–475.
- Oh-Hama T, Miyachi S. Chlorella. In: Borowitzka MA, Borowitzka LJ, editor. Microalgal biotechnology. Cambridge: Cambridge University Press; 1988. p. 3–26.
- Portielje R, Lijklema L. Kinetics of luxury uptake of phosphate by algae-dominated benthic communities. Hydrobiologia. 1994;275(1):349–358. doi: 10.1007/BF00026725
- Hessen DO, Færøvig PJ, Andersen T. Light, nutrients, and P:C ratios in algae: grazer performance related to food quality and quantity. Ecology. 2002;83(7):1886–1898. doi: 10.1890/0012-9658(2002)083[1886:LNAPCR]2.0.CO;2
- Wu H, Gau S, Li M, Chen Y, Sun C. Effects of Chlorella sp. on nutrient treatment in cultures with different carbon to nitrogen ratios. Wat Sci Tech. 2015;71(11):1597–1603. doi: 10.2166/wst.2015.123
- Chevalier P, De la Noue J. Efficiency of immobilized hyperconcentrated algae for ammonium and orthophosphate removal from wastewaters. Biotechnol Lett. 1985;7(6):395–400. doi: 10.1007/BF01166210
- Tang EPY, Vincent WF, Proulx D, Lessard P, De la Noüe J. Polar cyanobacteria versus green algae for tertiary waste-water treatment in cool climates. J Appl Phycol. 1997;9(4):371–381. doi: 10.1023/A:1007987127526
- Roberts DA, de Nys R, Paul NA. The effect of CO2 on algal growth in industrial waste water for bioenergy and bioremediation applications. PLoS ONE. 2013;8(11):e81631. doi:10.1371/journal.pone.0081631.
- Wang H, Gao L, Chen L, Guo F, Liu T. Integration process of biodiesel production from filamentous oleaginous microalgae Tribonema minus. Bioresour Technol. 2013;142:39–44. doi: 10.1016/j.biortech.2013.05.058
- Liu J, Vyverman W. Differences in nutrient uptake capacity of the benthic filamentous algae Cladophora sp., Klebsormidium sp. and Pseudanabaena sp. under varying N/P conditions. Bioresour Technol. 2015;179:234–242. doi: 10.1016/j.biortech.2014.12.028
- Schumacher G, Blume T, Sekoulov I. Bacteria reduction and nutrient removal in small wastewater treatment plants by an algal biofilm. Water Sci Technol. 2003;47:195–202.
- Congestri R, Di Pippo F, De Philippis R, Buttino I, Paradossi G, Albertano P. Seasonal succession of phototrophic biofilms in an Italian wastewater treatment plant: biovolume, spatial structure and exopolysaccharides. Aquat Microb Ecol. 2006;45(3):301–312. doi: 10.3354/ame045301
- Cromar NJ, Fallowfield HJ. Effect of nutrient loading and retention time on performance of high rate algal ponds. J Appl Phycol. 1997;9(4):301–309. doi: 10.1023/A:1007917610508
- Borowitzka MA. Commercial production of microalgae: ponds, tanks, tubes and fermenters. J Biotechnol. 1999;70(1–3):313–321. doi: 10.1016/S0168-1656(99)00083-8
- Park JBK, Craggs RJ. Wastewater treatment and algal production in high rate algal ponds with carbon dioxide addition. Water Sci Technol. 2010;61:633–639. doi: 10.2166/wst.2010.951
- Adey W, Luckett C, Jensen K. Phosphorus removal from natural waters using controlled algal production. Restor Ecol. 1993;1(1):29–39. doi: 10.1111/j.1526-100X.1993.tb00006.x
- Park JBK, Craggs RJ, Shilton AN. Wastewater treatment high rate algal ponds for biofuel production. Bioresour Technol. 2011;102(1):35–42. doi: 10.1016/j.biortech.2010.06.158
- Larsdotter K, La Cour Jansen J, Dalhammar G. Biologically mediated phosphorus precipitation in wastewater treatment with microalgae. Environ Technol. 2007;28(9):953–960. doi: 10.1080/09593332808618855
- Ullrich WR. Uptake and reduction of nitrate: algae and fungi. In: Läuchli A, Bieleski RL, editor. Inorganic plant nutrition. 15th ed. Berlin: Springer Verlag; 1983; p. 376–397.
- Talbot P, de la Noüe J. Tertiary treatment of wastewater with Phormidium bohneri (Schmidle) under various light and temperature conditions. Water Res. 1993;27(1):153–159. doi: 10.1016/0043-1354(93)90206-W
- Montastruc L, Azzaro-Pantel C, Biscans B, Cabassud M, Domenech S. A thermochemical approach for calcium phosphate precipitation modeling in a pellet reactor. Chem Eng J. 2003;94(1):41–50. doi: 10.1016/S1385-8947(03)00044-5
- Kim T, Lee Y, Han S, Hwang S. The effects of wavelength and wavelength mixing ratios on microalgae growth and nitrogen, phosphorus removal using Scenedesmus sp. for wastewater treatment. Bioresour Technol. 2013;130:75–80. doi: 10.1016/j.biortech.2012.11.134
- Lee CS, Lee S, Ko S, Oh H, Ahn C. Effects of photoperiod on nutrient removal, biomass production, and algal-bacterial population dynamics in lab-scale photobioreactors treating municipal wastewater. Water Res. 2015;68:680–691. doi: 10.1016/j.watres.2014.10.029
- Meseck SL, Alix JH, Wikfors GH. Photoperiod and light intensity effects on growth and utilization of nutrients by the aquaculture feed microalga, Tetraselmis chui (PLY429). Aquaculture. 2005;246(1):393–404. doi: 10.1016/j.aquaculture.2005.02.034
- Martinez M, Jimenez J, El Yousfi F. Influence of phosphorus concentration and temperature on growth and phosphorus uptake by the microalga Scenedesmus obliquus. Bioresour Technol. 1999;67(3):233–240. doi: 10.1016/S0960-8524(98)00120-5
- Mennaa FZ, Arbib Z, Perales JA. Urban wastewater treatment by seven species of microalgae and an algal bloom: biomass production, N and P removal kinetics and harvestability. Water Res. 2015;83:42–51. doi: 10.1016/j.watres.2015.06.007
- Larsdotter K. Wastewater treatment with microalgae-a literature review. Vatten. 2006;62(1):31–38.
- García J, Mujeriego R, Hernández-Mariné M. High rate algal pond operating strategies for urban wastewater nitrogen removal. J Appl Phycol. 2000;12(3–5):331–339. doi: 10.1023/A:1008146421368
- Picot B, Bahlaoui A, Moersidik S, Baleux B, Bontoux J. Comparison of the purifying efficiency of high rate algal pond with stabilization pond. Water Sci Technol. 1992;25(12):197–206.
- Di Termini I, Prassone A, Cattaneo C, Rovatti M. On the nitrogen and phosphorus removal in algal photobioreactors. Ecol Eng. 2011;37(6):976–980. doi: 10.1016/j.ecoleng.2011.01.006
- Ruiz J, Álvarez-Díaz PD, Arbib Z, Garrido-Pérez C, Barragán J, Perales JA. Performance of a flat panel reactor in the continuous culture of microalgae in urban wastewater: prediction from a batch experiment. Bioresour Technol. 2013;127(0):456–463. doi: 10.1016/j.biortech.2012.09.103
- Craggs RJ, Adey WH, Jenson KR, St. John MS, Green FB, Oswald WJ. Phosphorus removal from wastewater using an algal turf scrubber. Water Sci Technol. 1996;33:191–198. doi: 10.1016/0273-1223(96)00354-X
- Wei Q, Hu Z, Li G, Xiao B, Sun H, Tao M. Removing nitrogen and phosphorus from simulated wastewater using algal biofilm technique. Front Environ Sci Eng. 2008;2(4):446–451. doi: 10.1007/s11783-008-0064-2
- Christenson LB, Sims RC. Rotating algal biofilm reactor and spool harvester for wastewater treatment with biofuels by-products. Biotechnol Bioeng. 2012;109(7):1674–1684. doi: 10.1002/bit.24451
- Travieso L, Benitez F, Weiland P, Sánchez E, Dupeyrón R, Dominguez AR. Experiments on immobilization of microalgae for nutrient removal in wastewater treatments. Bioresour Technol. 1996;55(3):181–186. doi: 10.1016/0960-8524(95)00196-4
- Filippino KC, Mulholland MR, Bott CB. Phycoremediation strategies for rapid tertiary nutrient removal in a waste stream. Algal Res. 2015;11:125–133. doi: 10.1016/j.algal.2015.06.011
- Van Wagenen J, Pape ML, Angelidaki I. Characterization of nutrient removal and microalgal biomass production on an industrial waste-stream by application of the deceleration-stat technique. Water Res. 2015;75:301–311. doi: 10.1016/j.watres.2015.02.022
- Laliberté G, Proulx D, De Pauw N, De La Noüe J. Algal technology in wastewater treatment. Adv Limnol. 1994;42:283–302.
- Henderson RK, Parsons SA, Jefferson B. Successful removal of algae through the control of zeta potential. Sep Sci Technol. 2008a;43(7):1653–1666. doi: 10.1080/01496390801973771
- Henderson R, Parsons SA, Jefferson B. The impact of algal properties and pre-oxidation on solid–liquid separation of algae. Water Res. 2008b;42(8–9):1827–1845. doi: 10.1016/j.watres.2007.11.039
- Li Y, Horsman M, Wu N, Lan CQ, Dubois-Calero N. Biofuels from microalgae. Biotechnol Prog. 2008;24(4):815–820.
- Jarvis P, Buckingham P, Holden B, Jefferson B. Low energy ballasted flotation. Water Res. 2009;43(14):3427–3434. doi: 10.1016/j.watres.2009.05.003
- Ledwoch K, Gu S, Singh OA. Non-enclosure methods for non-suspended microalgae cultivation: literature review and research needs. Renew Sustain Energ Rev. 2015;42:1418–1427. doi: 10.1016/j.rser.2014.11.029
- Gross M, Jarboe D, Wen Z. Biofilm-based algal cultivation systems. Appl Microbiol Biotechnol. 2015;99(14):5781–5789. doi: 10.1007/s00253-015-6736-5
- Moreno-Garrido I. Microalgae immobilization: current techniques and uses. Bioresour Technol. 2008;99(10):3949–3964. doi: 10.1016/j.biortech.2007.05.040
- Ugwu CU, Aoyagi H, Uchiyama H. Photobioreactors for mass cultivation of algae. Bioresour Technol. 2008;99(10):4021–4028. doi: 10.1016/j.biortech.2007.01.046
- Grima EM, Fernández FA, Camacho FG, Chisti Y. Photobioreactors: light regime, mass transfer, and scaleup. J Biotechnol. 1999;70(1):231–247. doi: 10.1016/S0168-1656(99)00078-4
- Hoffmann JP. Wastewater treatment with suspended and nonsuspended algae. J Phycol. 1998;34(5):757–763. doi: 10.1046/j.1529-8817.1998.340757.x
- Craggs R, Sutherland D, Campbell H. Hectare-scale demonstration of high rate algal ponds for enhanced wastewater treatment and biofuel production. J Appl Phycol. 2012;24(3):329–337. doi: 10.1007/s10811-012-9810-8
- Sutherland DL, Howard-Williams C, Turnbull MH, Broady PA, Craggs RJ. Seasonal variation in light utilisation, biomass production and nutrient removal by wastewater microalgae in a full-scale high-rate algal pond. J Appl Phycol. 2014;26(3):1317–1329. doi: 10.1007/s10811-013-0142-0
- Molina E, Fernández J, Acién FG, Chisti Y. Tubular photobioreactor design for algal cultures. J Biotechnol. 2001;92(2):113–131. doi: 10.1016/S0168-1656(01)00353-4
- Sierra E, Acién FG, Fernández JM, García JL, González C, Molina E. Characterization of a flat plate photobioreactor for the production of microalgae. Chem Eng J. 2008;138(1–3):136–147. doi: 10.1016/j.cej.2007.06.004
- Cantrell KB, Ducey T, Ro KS, Hunt PG. Livestock waste-to-bioenergy generation opportunities. Bioresour Technol. 2008;99(17):7941–7953. doi: 10.1016/j.biortech.2008.02.061
- Craggs RJ, Adey WH, Jessup BK, Oswald WJ. A controlled stream mesocosm for tertiary treatment of sewage. Ecol Eng. 1996b;6(1–3):149–169. doi: 10.1016/0925-8574(95)00056-9
- Johnson MB, Wen Z. Development of an attached microalgal growth system for biofuel production. Appl Microbiol Biotechnol. 2010;85(3):525–534. doi: 10.1007/s00253-009-2133-2
- Rectenwald LL, Drenner RW. Nutrient removal from wastewater effluent using an ecological water treatment system. Environ Sci Technol. 2000;34(3):522–526. doi: 10.1021/es9908422
- Kesaano M, Sims RC. Algal biofilm based technology for wastewater treatment. Algal Res. 2014:5:231–240. doi: 10.1016/j.algal.2014.02.003
- Cohen Y. Biofiltration – the treatment of fluids by microorganisms immobilized into the filter bedding material: a review. Bioresour Technol. 2001;77(3):257–274. doi: 10.1016/S0960-8524(00)00074-2
- Craggs RJ. Wastewater treatment by algal turf scrubbing. Water Sci Technol. 2001;44:427–433.
- Mallick N. Biotechnological potential of immobilized algae for wastewater N, P and metal removal: a review. Biometals. 2002;15(4):377–390. doi: 10.1023/A:1020238520948
- de-Bashan LE, Bashan Y. Immobilized microalgae for removing pollutants: review of practical aspects. Bioresour Technol. 2010;101(6):1611–1627. doi: 10.1016/j.biortech.2009.09.043
- Tam NFY, Wong YS. Effect of immobilized microalgal bead concentrations on wastewater nutrient removal. Environ Pollut. 2000;107(1):145–151. doi: 10.1016/S0269-7491(99)00118-9
- Covarrubias SA, De-Bashan LE, Moreno M, Bashan Y. Alginate beads provide a beneficial physical barrier against native microorganisms in wastewater treated with immobilized bacteria and microalgae. Appl Microbiol Biotechnol. 2012;93(6):2669–2680. doi: 10.1007/s00253-011-3585-8
- European Commission. Directive 91/271/EEC of the Council of 21 May 1991 concerning urban waste water treatment. OJ Eur Commun. 1991;135:40–52.
- Karapinar Kapdan I, Aslan S. Application of the Stover-Kincannon kinetic model to nitrogen removal by Chlorella vulgaris in a continuously operated immobilized photobioreactor system. J Chem Technol Biotechnol. 2008;83(7):998–1005. doi: 10.1002/jctb.1905
- Roeselers G, Loosdrecht MCMV, Muyzer G. Phototrophic biofilms and their potential applications. J Appl Phycol. 2008;20(3)227–235. doi: 10.1007/s10811-007-9223-2
- Mesplé F, Casellas C, Troussellier M, Bontoux J. Modelling orthophosphate evolution in a high rate algal pond. Ecol Model. 1996;89(1–3):13–21. doi: 10.1016/0304-3800(95)00115-8
- Janssen M, Tramper J, Mur LR, Wijffels RH. Enclosed outdoor photobioreactors: light regime, photosynthetic efficiency, scale-up, and future prospects. Biotechnol Bioeng. 2003;81(2):193–210. doi: 10.1002/bit.10468
- Gris B, Morosinotto T, Giacometti GM, Bertucco A, Sforza E. Cultivation of Scenedesmus obliquus in photobioreactors: effects of light intensities and light–dark cycles on growth, productivity, and biochemical composition. Appl Biochem Biotechnol. 2014;172;5:2377–2389. doi: 10.1007/s12010-013-0679-z
- Liu J, Yuan C, Hu G, Li F. Effects of light intensity on the growth and lipid accumulation of microalga Scenedesmus sp. 11-1 under nitrogen limitation. Appl Biochem Biotechnol. 2012;166(8):2127–2137. doi: 10.1007/s12010-012-9639-2
- Arbib Z, Ruiz J, Álvarez-Díaz P, Garrido-Pérez C, Barragan J, Perales JA. Long term outdoor operation of a tubular airlift pilot photobioreactor and a high rate algal pond as tertiary treatment of urban wastewater. Ecol Eng. 2013;52:143–153. doi: 10.1016/j.ecoleng.2012.12.089
- Gordon JM, Polle JE. Ultrahigh bioproductivity from algae. Appl Microbiol Biotechnol. 2007;76(5):969–975. doi: 10.1007/s00253-007-1102-x
- Ogbonna JC, Tanaka H. Light requirement and photosynthetic cell cultivation – development of processes for efficient light utilization in photobioreactors. J Appl Phycol. 2000;12(3–5):207–218. doi: 10.1023/A:1008194627239
- Kumar K, Mishra SK, Shrivastav A, Park MS, Yang J. Recent trends in the mass cultivation of algae in raceway ponds. Renew Sustain Energ Rev. 2015;51:875–885. doi: 10.1016/j.rser.2015.06.033
- Lee C. Calculation of light penetration depth in photobioreactors. Biotechnol Bioproc Eng. 1999;4(1):78–81. doi: 10.1007/BF02931920
- Walker DA. Biofuels, facts, fantasy, and feasibility. J Appl Phycol. 2009;21(5):509–517. doi: 10.1007/s10811-009-9446-5
- Norsker N, Barbosa MJ, Vermuë MH, Wijffels RH. Microalgal production – a close look at the economics. Biotechnol Adv. 2011;29(1):24–27. doi: 10.1016/j.biotechadv.2010.08.005
- Figueroa FL, Aguilera J, Niell FX. Red and blue light regulation of growth and photosynthetic metabolism in Porphyra umbilicalis (Bangiales, Rhodophyta). Eur J Phycol. 1995;30(1):11–18. doi: 10.1080/09670269500650761
- Wang C, Fu C, Liu Y. Effects of using light-emitting diodes on the cultivation of Spirulina platensis. Biochem Eng J. 2007;37(1):21–25. doi: 10.1016/j.bej.2007.03.004
- Yeh N, Chung J. High-brightness LEDs – energy efficient lighting sources and their potential in indoor plant cultivation. Renew Sustain Energ Rev. 2009;13(8):2175–2180.
- Park K, Lee C. Optimization of algal photobiorectors isong flashing lights. Biotechnol Bioproc Eng. 2000;5(3):186–190. doi: 10.1007/BF02936592
- Ibrahim MA, MacAdam J, Autin O, Jefferson B. Evaluating the impact of LED bulb development on the economic viability of ultraviolet technology for disinfection. Environ Technol. 2014;35(4):400–406. doi: 10.1080/09593330.2013.829858
- Singh SP, Singh P. Effect of temperature and light on the growth of algae species: a review. Renew Sustain Energ Rev. 2015;50:431–444. doi: 10.1016/j.rser.2015.05.024
- Min M, Wang L, Li Y, et al. Cultivating Chlorella sp. in a pilot-scale photobioreactor using centrate wastewater for microalgae biomass production and wastewater nutrient removal. Appl Biochem Biotechnol. 2011;165(1):123–137. doi: 10.1007/s12010-011-9238-7
- Guzzon A, Bohn A, Diociaiuti M, Albertano P. Cultured phototrophic biofilms for phosphorus removal in wastewater treatment. Water Res. 2008;42(16):4357–4367. doi: 10.1016/j.watres.2008.07.029
- Travieso L, Benitez F, Dupeiron R. Sewage treatment using immobilized microalgae. Bioresour Technol. 1992;40(2):183–187. doi: 10.1016/0960-8524(92)90207-E
- Mallick N, Rai LC. Removal of inorganic ions from wastewaters by immobilized microalgae. World J Microbiol Biotechnol. 1994;10(4):439–443. doi: 10.1007/BF00144469