ABSTRACT
Obesity and associated metabolic complications, including diabetes, cardiovascular and hepatic diseases, and certain types of cancers, create a major socioeconomic burden. Obesity is characterized by excessive expansion of white adipose tissue resulting from increased adipocyte size, and enhanced adipocyte precursor cells proliferation and differentiation into mature adipocytes, a process well-defined as adipogenesis. Efforts to develop therapeutically potent strategies to circumvent obesity are impacted by our limited understanding of molecular mechanisms regulating adipogenesis. In this review, we discuss recently discovered molecular mechanisms restraining adipogenesis. In this perspective, the discoveries of white adipose tissue endogenous adipogenesis-regulatory cells (Aregs) that negatively regulate adipocyte differentiation, platelet-derived growth factor receptor isoform α (PDGFRα) activation and downstream signaling that hinder adipocyte precursors differentiation, and a group of obesity-associated non-coding RNAs (ncRNAs) that regulate adipogenesis open up promising therapeutic avenues to prevent and/or treat obesity.
Introduction
Under physiological conditions, human body weight is governed by a balance between energy intake and energy expenditure. In this regard, excessive calorie input without proper adjustment of calorie used through daily activities or resulting from adverse hormonal metabolic milieu contributes to the development of obesity [Citation1]. In recent years, obesity has become an extremely prevalent phenomenon which is still poorly understood from an etiologic-mechanistic perspective. Indeed, worldwide in 2016, an estimated 1.9 billion of the adults, 18 years and older, were overweight and with over 650 million of these were obese [Citation2] and effective pharmacological approaches available to prevent the development of obesity are very few. Given that obesity is a major risk of life-threatening diseases such as cardiovascular diseases, type 2 diabetes, non-alcoholic fatty liver disease (NAFLD) and cancer [Citation2–Citation4], obesity is now amongst the serious health issues.
Figure 1. Molecular mechanisms to harness adipogenesis.
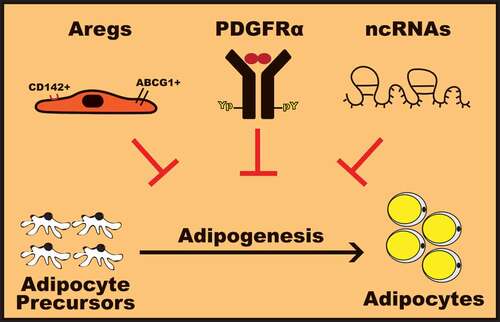
Obesity is characterized by an excessive accumulation of white adipose tissue (WAT). WAT, mainly composed of adipocytes that store lipid, plays an important role in the control of whole-body energy homeostasis [Citation1]. During normal development and abnormal expansion associated with obesity, WAT remodeling involves hypertrophy (increase in size) and adipocyte hyperplasia (increase in number) [Citation5,Citation6]. Adipocyte hyperplasia, defined as adipogenesis, is to a certain extent linked to the healthy expansion of WAT through proliferation and differentiation of adipocyte precursor cells (APCs) present in WAT stromal-vascular fraction (SVF) [Citation6]. In contrast, hypertrophic WAT expansion due to enlargement of existing adipocytes, along with macrophage infiltration and fibrosis, contributes to WAT dysfunction associated with insulin resistance and metabolic syndrome [Citation6,Citation7].
The process of adipogenesis is already well reviewed in various landmark publications [Citation8–Citation10]. Briefly, adipogenesis involves the regulation of a complex network of various transcription factors, which among others include the master regulators peroxisome proliferator-activated receptor gamma (PPARγ) and the family of CCAAT enhancer binding proteins (C/EBPs) [Citation8–Citation10]. Indeed, PPARγ along with C/EBPα function to control the transcription of various target genes that establish the adipocyte signature. However, through the years, additional players either potentiating or inhibiting differentiation of adipocyte precursor cells into adipocytes have been identified. In this review, we focus on the recent discoveries of novel molecular mechanisms limiting adipogenesis and their potential implications in preventing or treating obesity.
Adipogenesis-regulatory cells (Aregs)
Recently, Schwalie et al. uncovered a novel mechanism regulating de novo adipogenic capacity of WAT [Citation11]. Using a single-cell transcriptomics approach, this study revealed the existence of a distinct endogenous WAT SVF cell population displaying a low propensity to differentiate into adipocytes. Interestingly, this subpopulation of SVF cells, characterized by high expression of the cell surface proteins CD142 and the ATP-binding cassette sub-family G member 1 (ABCG1), negatively regulates ex vivo mouse and human APCs differentiation in a paracrine manner. Furthermore, the anti-adipogenic function of this SVF cell population is demonstrated in vivo by following high-fat diet-induced adipogenesis in mice implanted with matrigel embedded total or CD142−ABCG1− SVF cells. Interestingly, matrigel pads containing CD142−ABCG1− SVF cells displayed a significantly higher number of mature adipocytes than total SVF cells, further supporting that the CD142+ ABCG1+ cells prevent adipogenesis in vivo. Considering their functional similarity with the T regulatory cells (Tregs) in preventing autoimmunity [Citation12–Citation14], this subpopulation of SVF cells is defined as adipogenesis-regulatory cells (Aregs).
Interestingly, SVF from visceral WAT (vWAT) displays a significantly higher proportion of Aregs as compared to SVF isolated from subcutaneous WAT (scWAT) in humans [Citation11]. It is therefore proposed that the difference in the intrinsic potential of specific WAT depots toward adipogenesis could be attributed to variations in the number of resident Aregs. Following this hypothesis, the higher number of Aregs in visceral compared to subcutaneous fat would suggest that the visceral depot should be more resistant to hyperplasic expansion. However, lineage tracing studies using the AdipoChaser mice, a model tracking adipogenesis in vivo, demonstrated higher adipogenesis in epididymal WAT (eWAT) than scWAT upon high-fat diet or cold exposure challenges [Citation15]. The robust hyperplasic expansion of eWAT contradicts the presence of a significantly higher number of Aregs in visceral as compared to the subcutaneous fat depot in humans, weakening the hypothesis that the size of the Aregs population determines the rate of adipogenesis. On the other hand, we and others have reported that in mice, SVF from scWAT displays increased differentiation potential into adipocytes in vitro as compared to eWAT [Citation16–Citation19]. Similarly, human adipose stem cells (ASCs) isolated from scWAT have a higher adipogenic potential than vWAT ASCs [Citation16,Citation19], supporting that lower number of Aregs in subcutaneous fat depots may contribute to higher adipogenesis potential. Overall, the contradiction between the number of Aregs cells in visceral and subcutaneous fat depots and their respective adipogenic capacity could be attributed to yet unidentified pro- and anti-adipogenic factors between mice and humans. In addition, higher complexity between in vitro and in vivo adipogenesis could also lead to different outcomes, thus arising contradictory findings between the correlation of the number of Aregs in various WAT depots with their adipogenic capacity. Nevertheless, the recently discovered existence of the Aregs in various WAT depots potentially provides a novel avenue of investigation to design potential therapies to prevent obesity.
PDGFRα activation and signaling
Long-term overfeeding induces WAT APCs proliferation and differentiation into mature adipocytes, thus contributing to enhance hyperplasic expansion of WAT leading to obesity [Citation6]. Interestingly, while mature adipocytes lack the α isoform of the platelet-derived growth factor receptor tyrosine kinase (PDGFRα), WAT APCs express PDGFRα [Citation20] and increased number of PDGFRα-positive APCs contributes to the expansion of WAT upon high-fat diet [Citation21]. On the other hand, activation of PDGFRα signaling in APCs blocks differentiation into adipocytes in vitro and leads to WAT fibrosis in adult mice due to the conversion of APCs into the extracellular matrix (ECM)-producing fibroblasts rather than adipocytes [Citation22]. Therefore, activation of PDGFRα signaling dictates the balance between adipogenic and non-adipogenic precursor cell populations. Indeed, mice harboring PGDFRα-activating mutations display accumulation of fibroblasts-like stromal cell population associated with WAT fibrosis and reduced embryonic WAT depots [Citation23]. In this perspective, we recently reported that decreased adiposity in mice lacking the Src homology (SH) adaptor protein Nck1 correlates with ECM accumulation in WAT as well as impaired adipogenesis associated with enhanced PDGFRα activation and signaling [Citation18]. Therefore, targeting PDGFRα activation and signaling in APCs may be an interesting avenue to oppose increased adipocyte hyperplasia underlying excessive WAT expansion leading to obesity.
Non-coding RNAs (ncRNAs)
Evidence of ncRNAs was reported in the early 1980s with the identification of small nuclear RNAs involved in excision of introns. As a result, ncRNAs were considered to be exclusive building blocks of spliceosomes. However, in the early 2000s, the discovery of micro RNAs inducing translation inhibition advanced the field of ncRNAs [Citation24–Citation26]. Important progress in deep sequencing technology has led to the identification of additional members of ncRNA, especially the long non-coding RNAs that emerged as important regulators of cell- and tissue-specific post-transcriptional genes expression. Micro RNAs and long non-coding RNAs involvement in the regulation of adipogenesis and WAT biology is further discussed below.
Small non-coding micro RNAs (miRNAs)
Small ncRNA miRNAs, which are about 20–25 nucleotides, bind to specific target mRNAs to promote their degradation and/or prevent their translation [Citation27,Citation28]. MiRNAs are detected in all living organisms and actively participate in most normal biological processes, including development, differentiation, and metabolism, but their aberrant expression could result in the development of specific pathologies [Citation29,Citation30]. The mammalian genome is predicted to encode more than 3000 conserved miRNAs [Citation31], among them, several have been investigated in the context of obesity. In fact, an increasing number of genetic and epigenetic studies focusing on obesity revealed miRNAs as potent regulators of post-transcriptional expression of specific genes that are critical in the process of adipogenesis. In this regard, lists of miRNAs that enhance or inhibit adipocyte differentiation in murine preadipocytes or human APCs are reported in recent reviews [Citation32–Citation34]. Briefly, several miRNAs (miR-21, miR-29b, miR-144-3p, miR-148a, miR-210, miR-205-5p) enhance adipogenesis by interfering with the expression of molecular components involved in pathways that counteract adipogenesis (TGF-β, TNF-α, Wnt, corepressors of C/EBPα) [Citation35–Citation40]. Conversely, an important group of miRNAs that inhibits adipocyte differentiation (miR-27a and b, miR-31, miR-128-3p, miR-130a and b, miR-146a-5p, miR-155, miR-540) directly targets master regulators of adipogenic differentiation, such as C/EBPs and PPARγ [Citation41–Citation50]. In accord, these miRNAs are either downregulated during adipocyte differentiation or induced in conditions associated with inhibition of differentiation.
In contrast to the recognized role of miRNAs in regulating adipogenic differentiation, their significance in mature WAT biology still remains elusive. In this perspective, Koh et al. recently reported that in scWAT, lower levels of miR-30a correlate with insulin resistance in diet-induced obese mice and humans [Citation51]. In addition, overexpression of miR-30a in scWAT improves insulin sensitivity and energy expenditure, along with reducing ectopic fat deposition in the liver and inflammation within WAT depots in obese mice. Importantly, miR-30a exerted its anti-inflammatory action in WAT by directly targeting the 3ʹUTR of the transcription factor signal transducer and activator of transcription 1 (STAT1), resulting in lower endogenous STAT1 mRNA, protein expression and activity. Thus, miR-30a limits the STAT1-dependent signaling pathway mediating the pro-cytokines actions. In addition, miR-103 and miR-107 were recently shown to promote endoplasmic reticulum stress-mediated apoptosis in preadipocyte by directly suppressing the expression of Wnt3a, an activator of the canonical Wnt/β-catenin pathway [Citation52]. Therefore, these miRNAs might be involved in regulating the size of the preadipocyte population in WAT. Altogether, these recent studies clearly illustrate that in addition to perturbing signaling pathways interfering with adipogenesis, miRNAs also impact mature WAT homeostasis.
Worth mentioning, miRNAs are also detected in the plasma and could serve as important biomarkers for the diagnosis of specific diseases, although their role in the circulation is not fully understood. In this view, plasma miRNAs could serve as potential biomarkers for morbid obesity and related complications as shown by the profile of specific plasma miRNAs [Citation53]. Indeed, increased levels of miR-142-3p, miR-140-5p, miR-222 miR-143 and miR-130, and decreased concentrations of miR-532-5p, miR-423-5p, miR-520c-3p, miR-146a, and miR-15a were strongly associated with elevated circulating adipokines and leptin levels, body mass index as well as metabolic syndrome biomarkers in individuals with morbid obesity [Citation53]. In addition, dysregulated levels of circulating miRNAs in obesity are normalized upon acute weight loss [Citation54], further emphasizing that miRNAs could be useful clinical biomarkers to predict the development of obesity-related complications or to assess the efficiency of promising strategies inducing weight loss.
Long non-coding RNAs (lncRNAs)
A second class of ncRNAs, the lncRNAs, is arbitrarily defined based on their size larger than 200 nucleotides with no evidence for coding potential. LncRNAs regulate gene expression in cells and tissues as well as at various stages during development [Citation55,Citation56]. Like miRNAs, lncRNAs impact gene expression by binding to mRNA, however, they also bind to DNA to interfere with gene transcription. Furthermore, lncRNAs assist the formation of protein complexes involved in chromatin modification and titration of proteins away from their targets or their normal site of action. Finally, they could contribute to regulating gene expression through miRNA decoys. The biological function of most lncRNAs remains to be elucidated. Nevertheless, many lncRNAs are differentially expressed during adipogenesis and increasing evidence supports a significant role for lncRNAs in regulating this process [Citation57,Citation58].
The first lncRNA relevant to adipogenesis was reported in 2010 with the discovery that the non-coding RNA, steroid receptor RNA activator (SRA), behaves like a coactivator of PPARγ target genes expression by interacting with PPARγ [Citation59]. Since this discovery, additional lncRNAs were reported to be enriched in adipose tissue and strongly induced during adipogenesis [Citation57]. In accord, several studies identified PU.1 antisense, NEAT1, ADINR, ADNCR, H19, U90926, MIR31HG, and MEG3 [Citation60–Citation67] as lncRNAs that participate in multiple networks driving expression and function of genes regulating adipogenic differentiation. Furthermore, recent studies reported a role for lncRNAs ASMER and Plnc1 in adipocyte differentiation [Citation68,Citation69]. Indeed, these two lncRNAs were also upregulated in adipose tissue of obese mice and humans and involved in controlling the expression of genes in the adipogenic master transcriptional network, such as PPARγ.
Conclusion
Molecular mechanisms that prevent adipogenesis may serve as powerful targets for therapeutic interventions in obesity. In this review, we present recent advances in the field of adipogenesis by highlighting newly discovered mechanisms involved in limiting adipocyte differentiation (). Indeed, several recent studies support that differentiation of adipocyte precursor cells into adipocytes may be restricted by increasing the population of Aregs cells, promoting PDGFRα activation and signaling, and modulating expression of specific ncRNAs such as miRNAs and/or lncRNAs. In this perspective, further investigation is required to identify the anti-adipogenic factor released by Aregs cells and how it affects the differentiation of APCs. Developing cell-permeable molecules targeting intracellular negative regulators of PDGFRα signaling may also be of great interest. On the other hand, targeting APCs proliferation and differentiation by modulating the expression of specific ncRNAs, including miRNAs and lncRNAs involved in promoting or inhibiting adipogenesis may represent a powerful therapy to prevent or treat obesity. Using synthetic molecules or bioactive compounds that mimic or inhibit specific ncRNAs or modulating expression of specific ncRNAs through the powerful CRISPR-Cas9 gene editing approach may have a significant impact on obesity therapy. Considering these potential therapeutic avenues to prevent obesity, important challenges remain considering efficacy, toxicity, and specificity in targeting APCs. Nevertheless, it is of interest to consider that combination of multiple strategies targeting molecular mechanisms harnessing adipogenesis could emerge for beneficial personalized treatment of obesity and its related complications.
Author contribution
N.H. and L.L. contributed equally to this review.
Acknowledgments
Considering the abundant literature on adipogenesis, we apologize if some relevant papers were not cited here due to space limitation.
Disclosure statement
No potential conflict of interest was reported by the authors.
Additional information
Funding
References
- Rosen ED, Spiegelman BM. Adipocytes as regulators of energy balance and glucose homeostasis. Nature. 2006 Dec 14;444(7121):847–853. PubMed PMID: 17167472; PubMed Central PMCID: PMCPMC3212857.
- WHO. Obesity and overweight 2018; [ cited 2018 Feb 16]. Available from: https://www.who.int/news-room/fact-sheets/detail/obesity-and-overweight
- Farrell G. Insulin resistance, obesity, and liver cancer. Clin Gastroenterol Hepatol. 2014 Jan;12(1):117–119. PubMed PMID: 23954644.
- Segula D. Complications of obesity in adults: a short review of the literature. Malawi Med J. 2014 Mar;26(1):20–24. PubMed PMID: 24959321; PubMed Central PMCID: PMCPMC4062780.
- Haczeyni F, Bell-Anderson KS, Farrell GC. Causes and mechanisms of adipocyte enlargement and adipose expansion. Obes Rev. 2018 Mar;19(3):406–420. PubMed PMID: 29243339.
- Sun K, Kusminski CM, Scherer PE. Adipose tissue remodeling and obesity. J Clin Invest. 2011 Jun;121(6):2094–2101. PubMed PMID: 21633177; PubMed Central PMCID: PMCPMC3104761.
- Rosen ED, Spiegelman BM. What we talk about when we talk about fat. Cell. 2014 Jan 16;156(1–2):20–44. PubMed PMID: 24439368; PubMed Central PMCID: PMCPMC3934003.
- Lefterova MI, Lazar MA. New developments in adipogenesis. Trends Endocrinol Metab. 2009 Apr;20(3):107–114. PubMed PMID: 19269847.
- Rosen ED, MacDougald OA. Adipocyte differentiation from the inside out. Nat Rev Mol Cell Biol. 2006 Dec;7(12):885–896. PubMed PMID: 17139329.
- Tang QQ, Lane MD. Adipogenesis: from stem cell to adipocyte. Annu Rev Biochem. 2012;81:715–736. PubMed PMID: 22463691.
- Schwalie PC, Dong H, Zachara M, et al. A stromal cell population that inhibits adipogenesis in mammalian fat depots. Nature. 2018 Jul;559(7712):103–108. 10.1038/s41586-018-0226-8. PubMed PMID: 29925944.
- Buckner JH. Mechanisms of impaired regulation by CD4(+)CD25(+)FOXP3(+) regulatory T cells in human autoimmune diseases. Nat Rev Immunol. 2010 Dec;10(12):849–859. PubMed PMID: 21107346; PubMed Central PMCID: PMCPMC3046807.
- Cvetanovich GL, Hafler DA. Human regulatory T cells in autoimmune diseases. Curr Opin Immunol. 2010 Dec;22(6):753–760. PubMed PMID: 20869862; PubMed Central PMCID: PMCPMC2997859.
- Kasper IR, Apostolidis SA, Sharabi A, et al. Empowering regulatory T cells in autoimmunity. Trends Mol Med. 2016 Sep;22(9):784–797. PubMed PMID: 27461103; PubMed Central PMCID: PMCPMC5003773.
- Wang QA, Tao C, Gupta RK, et al. Tracking adipogenesis during white adipose tissue development, expansion and regeneration. Nat Med. 2013 Oct;19(10):1338–1344. PubMed PMID: 23995282; PubMed Central PMCID: PMCPMC4075943.
- Baglioni S, Cantini G, Poli G, et al. Functional differences in visceral and subcutaneous fat pads originate from differences in the adipose stem cell. PLoS One. 2012;7(5):e36569. PubMed PMID: 22574183; PubMed Central PMCID: PMCPMC3344924.
- Church CD, Berry R, Rodeheffer MS. Isolation and study of adipocyte precursors. Methods Enzymol. 2014;537:31–46. PubMed PMID: 24480340; PubMed Central PMCID: PMCPMC4276307.
- Haider N, Dusseault J, Larose L. Nck1 deficiency impairs adipogenesis by activation of PDGFRalpha in preadipocytes. iScience. 2018 Aug 31;6:22–37. PubMed PMID: 30240612; PubMed Central PMCID: PMCPMC6137712.
- Macotela Y, Emanuelli B, Mori MA, et al. Intrinsic differences in adipocyte precursor cells from different white fat depots. Diabetes. 2012 Jul;61(7):1691–1699. PubMed PMID: 22596050; PubMed Central PMCID: PMCPMC3379665.
- Miwa H, Era T. Tracing the destiny of mesenchymal stem cells from embryo to adult bone marrow and white adipose tissue via Pdgfralpha expression. Development. 2018 Jan 29;145(2):1-9. 10.1242/dev.155879. PubMed PMID: 29378823.
- Lee YH, Petkova AP, Mottillo EP, et al. In vivo identification of bipotential adipocyte progenitors recruited by beta3-adrenoceptor activation and high-fat feeding. Cell Metab. 2012 Apr 4;15(4):480–491. PubMed PMID: 22482730; PubMed Central PMCID: PMCPMC3322390.
- Iwayama T, Steele C, Yao L, et al. PDGFRalpha signaling drives adipose tissue fibrosis by targeting progenitor cell plasticity. Genes Dev. 2015 Jun 01;29(11):1106–1119. PubMed PMID: 26019175; PubMed Central PMCID: PMCPMC4470280.
- Sun C, Berry WL, Olson LE. PDGFRalpha controls the balance of stromal and adipogenic cells during adipose tissue organogenesis. Development. 2017 Jan 01 1;144:83–94. PubMed PMID: 28049691; PubMed Central PMCID: PMCPMC5278626.
- Lagos-Quintana M, Rauhut R, Lendeckel W, et al. Identification of novel genes coding for small expressed RNAs. Science. 2001 Oct 26;294(5543):853–858. PubMed PMID: 11679670.
- Lau NC, Lim LP, Weinstein EG, et al. An abundant class of tiny RNAs with probable regulatory roles in Caenorhabditis elegans. Science. 2001 Oct 26;294(5543):858–862. PubMed PMID: 11679671.
- Lee RC, Ambros V. An extensive class of small RNAs in Caenorhabditis elegans. Science. 2001 Oct 26;294(5543):862–864. PubMed PMID: 11679672.
- Chatterjee S, Grosshans H. Active turnover modulates mature microRNA activity in Caenorhabditis elegans. Nature. 2009 Sep 24;461(7263):546–549. PubMed PMID: 19734881.
- Djuranovic S, Nahvi A, Green R. miRNA-mediated gene silencing by translational repression followed by mRNA deadenylation and decay. Science. 2012 Apr 13;336(6078):237–240. PubMed PMID: 22499947; PubMed Central PMCID: PMCPMC3971879.
- Jiang Q, Wang Y, Hao Y, et al. miR2Disease: a manually curated database for microRNA deregulation in human disease. Nucleic Acids Res. 2009 Jan;37(Database issue):D98–104. PubMed PMID: 18927107; PubMed Central PMCID: PMCPMC2686559.
- Krichevsky AM, King KS, Donahue CP, et al. A microRNA array reveals extensive regulation of microRNAs during brain development. RNA. 2003 Oct;9(10):1274–1281. PubMed PMID: 13130141; PubMed Central PMCID: PMCPMC1370491.
- Londin E, Loher P, Telonis AG, et al. Analysis of 13 cell types reveals evidence for the expression of numerous novel primate- and tissue-specific microRNAs. Proc Natl Acad Sci U S A. 2015 Mar 10;112(10):E1106–15. PubMed PMID: 25713380; PubMed Central PMCID: PMCPMC4364231.
- Lorente-Cebrian S, Gonzalez-Muniesa P, Milagro FI, et al. MicroRNAs and other non-coding RNAs in adipose tissue and obesity: emerging roles as biomarkers and therapeutic targets. Clin Sci (Lond). 2019 Jan 15;133(1):23–40. PubMed PMID: 30606812.
- Sadie-Van Gijsen H. Adipocyte biology: it is time to upgrade to a new model. J Cell Physiol. 2018. DOI:10.1002/jcp.27266
- Son YH, Ka S, Kim AY, et al. Regulation of adipocyte differentiation via MicroRNAs. Endocrinol Metab (Seoul). 2014 Jun;29(2):122–135. PubMed PMID: 25031884; PubMed Central PMCID: PMCPMC4091493.
- Kim YJ, Hwang SJ, Bae YC, et al. MiR-21 regulates adipogenic differentiation through the modulation of TGF-beta signaling in mesenchymal stem cells derived from human adipose tissue. Stem Cells. 2009 Dec;27(12):3093–3102. PubMed PMID: 19816956.
- Shi C, Zhang M, Tong M, et al. miR-148a is associated with obesity and modulates adipocyte differentiation of mesenchymal stem cells through Wnt signaling. Sci Rep. 2015 May 22;5:9930. PubMed PMID: 26001136; PubMed Central PMCID: PMCPMC4441322.
- Zhang XM, Wang LH, Su DJ, et al. MicroRNA-29b promotes the adipogenic differentiation of human adipose tissue-derived stromal cells. Obesity (Silver Spring). 2016 May;24(5):1097–1105. PubMed PMID: 27030318.
- Qin LC, Niu Y, Chen W, et al. A deep investigation into the adipogenesis mechanism: profile of microRNAs regulating adipogenesis by modulating the canonical Wnt/β-catenin signaling pathway. BMC Genomics. 2010;11(320):1-10.
- He H, Chen K, Wang F, et al. miR-204-5p promotes the adipogenic differentiation of human adipose-derived mesenchymal stem cells by modulating DVL3 expression and suppressing Wnt/beta-catenin signaling. Int J Mol Med. 2015 Jun;35(6):1587–1595. PubMed PMID: 25847080; PubMed Central PMCID: PMCPMC4432921.
- Shen L, Li Q, Wang J, et al. miR-144-3p promotes adipogenesis through releasing C/EBPalpha from Klf3 and CtBP2. Front Genet. 2018;9:677. PubMed PMID: 30619490; PubMed Central PMCID: PMCPMC6305703.
- Chen L, Chen Y, Zhang S, et al. MiR-540 as a novel adipogenic inhibitor impairs adipogenesis via suppression of PPARγ. J Cell Biochem. 2015 Jun;116(6):969–976. PubMed PMID: 25560764.
- Karbiener M, Fischer C, Nowitsch S, et al. microRNA miR-27b impairs human adipocyte differentiation and targets PPARγ. Biochem Biophys Res Commun. 2009 Dec 11;390(2):247–251. PubMed PMID: 19800867.
- Kim SY, Kim AY, Lee HW, et al. miR-27a is a negative regulator of adipocyte differentiation via suppressing PPARγ expression. Biochem Biophys Res Commun. 2010 Feb 12;392(3):323–328. PubMed PMID: 20060380.
- Lee EK, Lee MJ, Abdelmohsen K, et al. miR-130 suppresses adipogenesis by inhibiting peroxisome proliferator-activated receptor gamma expression. Mol Cell Biol. 2011 Feb;31(4):626–638. PubMed PMID: 21135128; PubMed Central PMCID: PMCPMC3028659.
- Lin Q, Gao Z, Alarcon RM, et al. A role of miR-27 in the regulation of adipogenesis. FEBS J. 2009 Apr;276(8):2348–2358. PubMed PMID: 19348006; PubMed Central PMCID: PMCPMC5330386.
- Wu D, Xi QY, Cheng X, et al. miR-146a-5p inhibits TNF-alpha-induced adipogenesis via targeting insulin receptor in primary porcine adipocytes. J Lipid Res. 2016 Aug;57(8):1360–1372. PubMed PMID: 27324794; PubMed Central PMCID: PMCPMC4959853.
- Chen C, Deng Y, Hu X, et al. miR-128-3p regulates 3T3-L1 adipogenesis and lipolysis by targeting Pparg and Sertad2. J Physiol Biochem. 2018 Aug;74(3):381–393. 10.1007/s13105-018-0625-1. PubMed PMID: 29654510.
- Liu S, Yang Y, Wu J. TNFalpha-induced up-regulation of miR-155 inhibits adipogenesis by down-regulating early adipogenic transcription factors. Biochem Biophys Res Commun. 2011 Oct 28;414(3):618–624. PubMed PMID: 21986534.
- Sun F, Wang J, Pan Q, et al. Characterization of function and regulation of miR-24-1 and miR-31. Biochem Biophys Res Commun. 2009 Mar 13;380(3):660–665. PubMed PMID: 19285018.
- Tang YF, Zhang Y, Li XY, et al. Expression of miR-31, miR-125b-5p, and miR-326 in the adipogenic differentiation process of adipose-derived stem cells. OMICS. 2009 Aug;13(4):331–336. PubMed PMID: 19422302.
- Koh EH, Chernis N, Saha PK, et al. miR-30a remodels subcutaneous adipose tissue inflammation to improve insulin sensitivity in obesity. Diabetes. 2018 Jul 12;67:2541–2553. PubMed PMID: 30002134.
- Zhang Z, Wu S, Muhammad S, et al. miR-103/107 promote ER stress-mediated apoptosis via targeting the Wnt3a/beta-catenin/ATF6 pathway in preadipocytes. J Lipid Res. 2018 May;59(5):843–853. PubMed PMID: 29483204; PubMed Central PMCID: PMCPMC5928437.
- Al-Rawaf HA. Circulating microRNAs and adipokines as markers of metabolic syndrome in adolescents with obesity. Clin Nutr. 2018 Sep 25. DOI:10.1016/j.clnu.2018.09.024. PubMed PMID: 30309709.
- Manning P, Munasinghe PE, Bellae Papannarao J, et al. Acute weight loss restores dysregulated circulating microRNAs in obese individuals. J Clin Endocrinol Metab. 2018 Oct 31. DOI:10.1210/jc.2018-00684. PubMed PMID: 30383229.
- Derrien T, Johnson R, Bussotti G, et al. The GENCODE v7 catalog of human long noncoding RNAs: analysis of their gene structure, evolution, and expression. Genome Res. 2012 Sep;22(9):1775–1789. PubMed PMID: 22955988; PubMed Central PMCID: PMCPMC3431493.
- Washietl S, Kellis M, Garber M. Evolutionary dynamics and tissue specificity of human long noncoding RNAs in six mammals. Genome Res. 2014 Apr;24(4):616–628. PubMed PMID: 24429298; PubMed Central PMCID: PMCPMC3975061.
- Sun L, Goff LA, Trapnell C, et al. Long noncoding RNAs regulate adipogenesis. Proc Natl Acad Sci U S A. 2013 Feb 26;110(9):3387–3392. PubMed PMID: 23401553; PubMed Central PMCID: PMCPMC3587215.
- Yang Q, Wan Q, Zhang L, et al. Analysis of LncRNA expression in cell differentiation. RNA Biol. 2018 Mar 4;15(3):413–422. PubMed PMID: 29508657; PubMed Central PMCID: PMCPMC5927722.
- Xu B, Gerin I, Miao H, et al. Multiple roles for the non-coding RNA SRA in regulation of adipogenesis and insulin sensitivity. PLoS One. 2010 Dec 2;5(12):e14199. PubMed PMID: 21152033; PubMed Central PMCID: PMCPMC2996286.
- Chen J, Liu Y, Lu S, et al. The role and possible mechanism of lncRNA U90926 in modulating 3T3-L1 preadipocyte differentiation. Int J Obes (Lond). 2017 Feb;41(2):299–308. PubMed PMID: 27780975; PubMed Central PMCID: PMCPMC5309343.
- Cooper DR, Carter G, Li P, et al. Long non-coding RNA NEAT1 associates with SRp40 to temporally regulate PPARγ2 splicing during adipogenesis in 3T3-L1 cells. Genes (Basel). 2014 Nov 27;5(4):1050–1063. PubMed PMID: 25437750; PubMed Central PMCID: PMCPMC4276926.
- Huang Y, Jin C, Zheng Y, et al. Knockdown of lncRNA MIR31HG inhibits adipocyte differentiation of human adipose-derived stem cells via histone modification of FABP4. Sci Rep. 2017 Aug 14;7(1):8080. PubMed PMID: 28808264; PubMed Central PMCID: PMCPMC5556051.
- Huang Y, Zheng Y, Jin C, et al. Long non-coding RNA H19 inhibits adipocyte differentiation of bone marrow mesenchymal stem cells through epigenetic modulation of histone deacetylases. Sci Rep. 2016 Jun 28;6:28897. PubMed PMID: 27349231; PubMed Central PMCID: PMCPMC4924093.
- Li M, Sun X, Cai H, et al. Long non-coding RNA ADNCR suppresses adipogenic differentiation by targeting miR-204. Biochim Biophys Acta. 2016 Jul;1859(7):871–882. PubMed PMID: 27156885.
- Li Z, Jin C, Chen S, et al. Long non-coding RNA MEG3 inhibits adipogenesis and promotes osteogenesis of human adipose-derived mesenchymal stem cells via miR-140-5p. Mol Cell Biochem. 2017 Sep;433(1–2):51–60. PubMed PMID: 28382492.
- Pang WJ, Lin LG, Xiong Y, et al. Knockdown of PU.1 AS lncRNA inhibits adipogenesis through enhancing PU.1 mRNA translation. J Cell Biochem. 2013 Nov;114(11):2500–2512. PubMed PMID: 23749759.
- Xiao T, Liu L, Li H, et al. Long noncoding RNA ADINR regulates adipogenesis by transcriptionally activating C/EBPα. Stem Cell Rep. 2015 Nov 10;5(5):856–865. PubMed PMID: 26489893; PubMed Central PMCID: PMCPMC4649137.
- Gao H, Kerr A, Jiao H, et al. Long non-coding RNAs associated with metabolic traits in human white adipose tissue. EBioMedicine. 2018 Apr;30:248–260. PubMed PMID: 29580841; PubMed Central PMCID: PMCPMC5952343.
- Zhu E, Zhang J, Li Y, et al. Long noncoding RNA Plnc1 controls adipocyte differentiation by regulating peroxisome proliferator-activated receptor gamma. FASEB J. 2018 Oct2:fj201800739RRR. DOI:10.1096/fj.201800739RRR. PubMed PMID: 30277818.