Abstract
Adoptive transfer of allogeneic natural killer (NK) cells represents a promising treatment approach against cancer, including acute myeloid leukemia (AML). Previously, we reported a cytokine-based culture method for the generation of NK cell products with high cell number and purity. In this system, CD34+ hematopoietic progenitor cells (HPC) were expanded and differentiated into NK cells under stroma-free conditions in the presence of IL-15 and IL-2. We show that combining IL-15 with IL-12 drives the generation of more mature and highly functional NK cells. In particular, replacement of IL-2 by IL-12 enhanced the cytolytic activity and IFNγ production of HPC-NK cells toward cultured and primary AML cells in vitro, and improved antileukemic responses in NOD/SCID-IL2Rγnull (NSG) mice bearing human AML cells. Phenotypically, IL-12 increased the frequency of HPC-NK cells expressing NKG2A and killer immunoglobulin-like receptor (KIR), which were more responsive to target cell stimulation. In addition, NK15/12 cell products demonstrated superior maturation potential, resulting in >70% positivity for CD16 and/or KIR within 2 weeks after infusion into NSG mice. We predict that higher functionality and faster in vivo maturation will favor HPC-NK cell alloreactivity toward malignant cells in patients, making this cytokine combination an attractive strategy to generate clinical HPC-NK cell products for cancer adoptive immunotherapy.
Introduction
Adoptive transfer of natural killer (NK) cells is a novel relatively non-toxic and promising treatment approach against cancer, including acute myeloid leukemia (AML).Citation1 In the context of allogeneic hematopoietic stem cell transplantation (HSCT), it is well established that fast repopulating alloreactive NK cells mediate an efficient graft-versus-leukemia reaction and improve control of relapse without causing graft-versus-host disease.Citation2-4 Importantly, prolonged relapse-free survival has been demonstrated in allotransplanted AML patients in the setting of killer immunoglobulin-like receptor (KIR)-ligand mismatch between patients and allogeneic donors.Citation5-7 In this situation, AML cells of the patient lack an inhibitory HLA ligand for the allogeneic donor NK cells, resulting in the absence of inhibitory signals for NK cells and potent activation upon engagement of activating receptors. These clinical observations raised interest in exploiting NK cell adoptive immunotherapy as an adjuvant treatment approach against AML. In the non-transplant setting, adoptive transfer of allogeneic NK cells also demonstrated clinical activity in AML patients with poor prognosisCitation8,9 and in childhood AML with induction of long-term remission.Citation10 In these trials, NK cell infusions were given following fludarabine and cyclophosphamide conditioning, which resulted in a marked increase in endogenous IL-15 that favored in vivo survival and expansion of infused NK cells in the majority of treated patients.Citation8,9
Although infusions with peripheral blood derived-NK cells appears to be safe, NK cell enrichment from leukapheresis products yields relatively low NK cell numbers with contaminating alloreactive T cells.Citation11-14 To overcome these limitations, several methods have been explored to activate and expand NK cells before adoptive transfer.Citation15–20 Alternatively, NK cells can be generated from hematopoietic progenitor cells (HPCs).Citation21-25 Previously, we reported a good manufacturing practice (GMP)-compliant, cytokine-based culture protocol for the ex vivo generation of allogeneic NK cells from CD34+ HPCs isolated from cryopreserved umbilical cord blood (UCB) units, bone marrow (BM), or G-CSF-mobilized blood (see ref. 23 and unpublished data). Purified CD34+ progenitor cells are expanded in closed, large-scale bioreactors to achieve clinically relevant doses of NK cell products completely devoid of allogeneic T cells.Citation25 In addition, pre-clinical studies conducted in NOD/SCID-IL2Rγnull (NSG) mice demonstrated that these HPC-NK cells have BM homing capacity, display IL-15–driven in vivo expansion, and prolong survival of leukemia-bearing mice.Citation26
Currently, we generate HPC-NK cells under stroma-free conditions in the presence of IL-15 and IL-2. However, several other cytokines are known to promote NK cell development, activation, and function.Citation27-29 Although IL-15 plays a crucial role during NK cell development as well as in the survival and expansion of NK cells in vivo, recent studies also demonstrated that preactivation with combinations of the cytokines IL-12, IL-15, and IL-18 results in “priming” of memory-like NK cells with enhanced functional properties.Citation30-32 Although the precise underlying mechanism remains unknown, this prime-boost strategy using distinct combinations of cytokines may pave the way to improved NK cell immunotherapeutic strategies.
A previous study showed that addition of IL-12 to the culture system increased maturation of HPC-NK cells as well as their killing potency against leukemia cell lines.Citation29 In the present study, we further investigated the influence of IL-12 on ex vivo-generated HPC-NK cells in vitro and in vivo. We demonstrated that replacement of IL-2 by IL-12 favors the development of KIR-expressing NK cells, which display superior IFNγ production and cytolytic activity against AML cells, including cell lines and patient-derived material. In vitro co-culture studies indicated that higher NK cell cytolytic activity was associated with IFNγ-mediated upregulation of ICAM-1 on AML cells, thereby strengthening cell–cell contact between NK cells and tumor cells. In vivo, HPC-NK cell products generated with IL-15 and IL-12 demonstrated faster maturation potential compared to IL-15/IL-2 cultured NK cells, resulting in a higher frequency of CD16+KIR+ alloreactive NK cells shortly after adoptive transfer. In addition, infusion of NK15/12 cell products improved antileukemic responses in MHC-I+ AML cell-bearing mice. Together, our data indicate that combining IL-15 with IL-12 for the generation of clinical HPC-NK cell products is an attractive strategy to improve the antileukemic response in patients.
Results
Replacement of IL-2 by IL-12 in combination with IL-15 generates HPC-NK cells with higher NKG2A, CD16, and KIR expression
Because of its NK potentiating effect, we have examined the influence of IL-12, in combination with IL-15 and IL-2, on the generation and functionality of HPC-NK cells. To this end, IL-12 was introduced into our culture system from day 14 onwards at a low concentration that had no impact on the purity of the final product (Fig. S1A-B). Although addition of IL-12 reduced the expansion rate of the total cells, this effect was limited when combining IL-15 with IL-12 only (Fig. S1C-D). Phenotypically, replacement of IL-2 by IL-12 resulted in higher percentages of CD56+ cells expressing NKG2A and inhibitory KIRs (Fig. S1E-F). Despite variability between donors, a slightly increased percentage of CD16+ NK cells was noticed in NK15/12 compared to NK15/2 cells. Notably, NK15/12 cell products showed slightly lower levels of CD56 expression at the end of the culture process (CD56 mean fluorescent units [MFI]: 152 ± 10.7 versus 124.2 ± 9.0 for NK15/2 versus NK15/12 respectively, data not shown), but this was not related to a specific NK cell subset. We also found higher percentages of CD62L positivity. Expression of the activating receptors NKG2D, natural cytotoxicity receptors, and DNAM-1 were similar in all NK cell cultures analyzed at the end of the process (data not shown). Therefore, further investigations concentrated on comparing NK15/2 and NK15/12 cells.
Combining IL-15 with IL-12 generates HPC-NK cells with enhanced cytolytic activity against AML in vitro
First, we examined the effect of IL-12 on HPC-NK cell reactivity toward leukemia cells. Although both NK15/2 and NK15/12 cell products showed high cytolytic activity against NK-sensitive K562 cells, increased killing of MHC class I-positive AML cells THP-1 and KG-1a was observed with NK15/12 cells (). Enhanced reactivity toward target cells was confirmed by elevated levels of granzyme B produced by NK15/12 compared to NK15/2 cells (). Levels of IFNγ secretion were also increased with NK15/12 cells (), whereas production of TNFα was relatively low and similar for both NK products (). Most importantly, higher susceptibility of patient-derived primary AML cells to NK15/12-mediated killing was demonstrated at a low effector-to-target ratio, including differentiated M4-M5 AMLs as well as more immature CD34+ M1-M2 AMLs (, Fig. S2; for AML patient characteristics see Table S1). These data demonstrate that combining IL-15 with IL-12 drives the generation of HPC-NK cells with enhanced cytolytic activity against AML cells in vitro.
Figure 1. Combining IL-15 with IL-12 enhanced the cytolytic activity and IFNγ production capacity of HPC-NK cells toward AML. The functionalities of HPC-NK cell products were assessed after overnight co-culture with target cells. (A) Percentage killing of K562, THP-1, and KG-1a cells at increasing effector cell:target (E:T) ratio. Combined results (mean ± SEM) of 7 independent experiments, each performed in triplicate with different HPC-NK cell donors, are shown. (B-D) Granzyme B, IFNγ, and TNFα levels were determined by ELISA in co-culture supernatants of 5 × 104 NK cells with target cells at a E:T ratio of 1:1. Mean values ± SEM of 5 donors are shown. (E) Percentage killing (mean ± SD) of patient-derived primary AML cells (E:T = 3:1). The cytolytic activity of HPC-NK cells derived from donor I (undetermined HLA-C type) was addressed using a CFSE-based cytotoxicity assay, whereas viable AML cells were identified by flow cytometry using 7AAD and CD33/CD34/CD56 markers for donor II (HLA-C group C1/C2). Statistical analysis was performed using a 2-way ANOVA (with repeated measurements in panels A-D), *P < 0.05, **P < 0.01, ***P < 0.001.
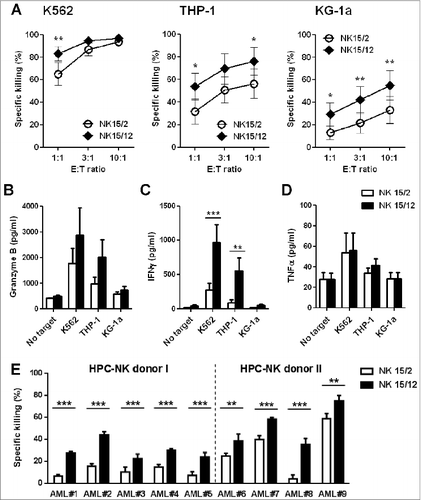
Combining IL-15 with IL-12 favors development of more mature and highly functional NKG2A+KIR+ natural killer cells
Next, we aimed to understand how IL-12 influenced the increased killing activity of HPC-NK cells. Besides similar expression of activating receptors, we could not identify any significant difference in the cytolytic machinery between both NK cell products. At the protein level, NK15/2 and NK15/12 cells displayed comparable expression of perforin and granzyme B (data not shown), as well as TRAIL and FasL (). Gene expression profiles of perforin and granzyme B were also similar, as were those for the transcription factors eomesodermin and TBX21 (), which were described as important modulators of NK cell maturation, function, and exhaustion.Citation33,34 Next, we examined NK cell reactivity at the single-cell level upon overnight stimulation with K562 cells. As shown in , a small but significant increase in CD107a+ degranulating cells was noticed among NK15/12 cells. More strikingly, the fraction of IFNγ-producing cells was strongly augmented. In accordance with the levels of IFNγ detected in co-culture supernatants, IFNγ-positivity was almost absent in NK15/2 cells whereas a clear population of CD107a+ IFNγ+ cells was visualized in all tested NK15/12 cell products. Interestingly, examination of the NK cell phenotype in combination with IFNγ production showed enrichment of responsive cells within the NKG2A+KIR+ population (), supporting the conclusion that IL-12 favors the development of more mature and highly functional NK cells.
Figure 2 (See previous page). Replacement of IL-2 by IL-12 favors the development of more mature and highly functional KIR+ natural killer cells. (A) Representative expression level of TRAIL and FasL on CD56+ HPC-NK cells. Numbers indicate ΔMFI between isotype control and specific staining. (B) Relative gene expression of the cytolytic mediators perforin (PRF1) and granzyme B (GrzB), and the transcription factors eomesodermin (EOMES) and t-bet (TBX21). Mean values ± SD of 2 HPC-NK cell donors are shown, one generated with GBGM and the second one using SCGM. (C-D) Percentages of degranulating and IFNγ-producing NK cells were determined upon overnight stimulation with K562 cells at an E:T ratio of 1:1 and in the presence of low dose IL-15 (5 ng/mL). Percentages of CD107+ and IFNγ+ cells were determined with subtraction of signals measured with unstimulated cells. One representative experiment (C) and a summary of 10 donors (D, mean ± SEM) are shown. (E-F) The IFNγ production capacity of NK15/12 cells was examined within different subsets identified using NKG2A and KIRs differentiation markers. One representative donor (E) and a summary of 4 donors (F, mean ± SEM) are shown. Differences were analyzed using a paired t-test (D) or one-way ANOVA (F), *P < 0.05, **P < 0.01.
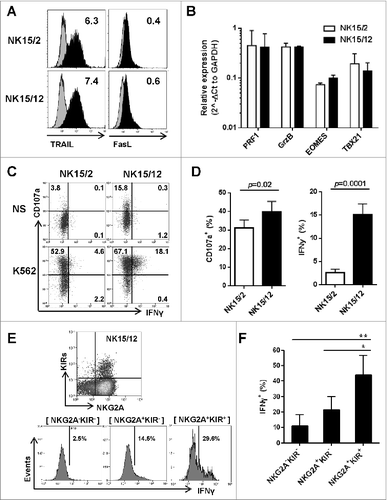
Higher cytolytic activity of NK15/12 cells is associated with superior IFNγ production capacity and ICAM-1 upregulation on AML in vitro
With respect to the level of KIRs expressed on NK15/2 and NK15/12 cells, the data described above might suggest that the enhanced AML killing relied on differential KIR-mediated alloreactivity of HPC-NK cells. However, increased cytolytic activity of NK15/12 cells was consistently observed in vitro irrespective of the HLA type of AML cells, including against C1/C2/Bw4-positive KG-1a cells and patient-derived AML blasts (, Fig. S2). In a recent study, Sun and colleagues established a direct link between cytokine production and cytolysis of NK cells, as a result of IFNγ/TNFα-induced upregulation of intercellular adhesion molecule 1 (ICAM-1) on tumor cells.Citation35 To address this possibility, we first examined the expression level of adhesion molecules on AML cell lines. As illustrated in , we found a dose-dependent upregulation of ICAM-1 on THP-1 and KG-1a cells 24 h after incubation with IFNγ and TNFα. For comparison, basal expression of LFA-3 was high in both AML cell lines and remained unchanged under inflammation. Moreover, NK15/2 and NK15/12 cells expressed a similar level of CD11a (), supporting the idea that enhanced killing capacity of NK15/12 cells might rely on their potency to strengthen the LFA-1/ICAM-1–mediated interaction with target cells through inflammatory cytokine release. Accordingly, addition of recombinant IFNγ increased killing of AML cells by NK15/2 cells (), whereas blocking ICAM-1 inhibited NK cell activity (). Thereafter, we examined ICAM-1 expression on primary AML cells. In line with previous results, upregulation of ICAM-1 was observed upon inflammation as well as in the presence of HPC-NK cells (data not shown). More interestingly, analysis of ICAM-1 levels and relative NK killing over time supported the contribution of ICAM-1 upregulation to AML cell susceptibility to NK cells. In particular, preferential lysis of ICAM-1high AML cells was observed using blasts from one AML patient (Fig. S3), therefore we could not measure increased ICAM-1 levels on the remaining viable AML cells. For another patient, in whom the ICAM-1 level was relatively homogenous within CD33+ AML cells, ICAM-1 upregulation was more pronounced in the presence of NK15/12 cells at 48 and 72 h of co-culture (). This was accompanied by a progressive increase in AML cell killing by NK15/12 cells (), while both NK cells showed sustained expression of CD11a (). In these experiments, differential IFNγ production toward primary AML cells was confirmed, but treatment with inflammatory cytokines alone did not result in any significant upregulation of NK-activating ligands on AML cells (Fig. S4). Together, these data strongly suggest that IL-12 enhances HPC-NK cell cytolytic activity through increased IFNγ production capacity.
Figure 3. IFNγ-mediated upregulation of ICAM-1 on AML cells strengthens NK cell cytolytic activity. (A) Expression of ICAM-1 and LFA-3 on AML cell lines after 24 h culture with increasing concentrations of IFNγ and TNFα. (B) Expression profile of CD11a on NK15/2 and NK15/12 cells at the end of the culture process. Histograms from one representative HPC-NK cell donor out of 3 analyzed are shown. (C) Overnight THP-1 and KG-1a cell killing by NK15/2 versus NK15/12 cells, or NK15/2 cells in the presence of 1,000 pg/mL recombinant IFNγ (E:T ratio 3:1, mean ± SD). (D) Inhibition of HPC-NK cell killing in the presence of anti-ICAM-1 antibody (10 µg/mL). (E-G) Co-culture experiments of NK15/2 or NK15/12 cells with patient-derived primary AML cells performed at an E:T ratio 2:1 and in the presence of low dose IL-15 (5 ng/mL) for 24, 48, and 72 h. (E) Relative ICAM-1 expression level on AML cells and (F) relative killing (mean ± SD) of AML cells overtime upon co-culture with NK cells. The NK specific killing of AML cells was determined at each time point based on the number of surviving AML cells alone as described in the Materials and Methods section, and was depicted as relative killing compared to NK15/2 cells. (G) Expression of CD11a on HPC-NK cells upon co-culture with AML cells measured at 48 h. Data shown in (E-G) were obtained with AML#7 cells. Statistical analysis was performed using a one-way ANOVA (C) and Student t test (D, F), **P < 0.01, ***P < 0.001, ****P < 0.0001.
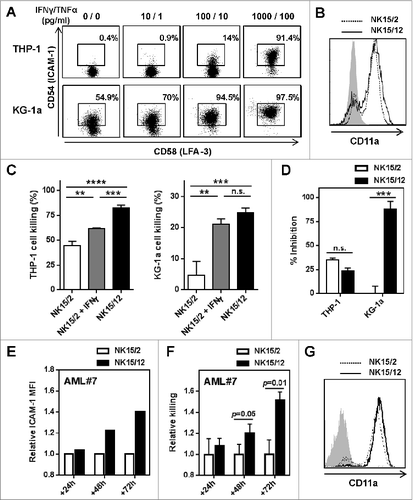
HPC-NK15/12 cell products display faster maturation potential in vivo
In addition to the analysis of HPC-NK cell products in vitro, we wondered about the NK15/2 and NK15/12 cell fate in vivo. Therefore, we conducted adoptive transfer studies in NSG mice that were infused with either NK15/2 or NK15/12 cells and sacrificed 1 week later for ex vivo flow cytometric analysis. As shown in , both NK15/2 and NK15/12 cells showed high and sustained expression of various activation markers including NKG2D, NKp46, and DNAM-1, as well as CXCR3, which is, among others, an important chemokine receptor for NK cell recruitment to tumor and inflammation sites.Citation36 In contrast, differences were found among NK cell maturation markers; notably, increased levels of NK cells expressing CD16 and KIRs were measured 1 week after infusion (). With respect to the infused NK cell products, NK15/12 cells experienced CD16 acquisition to the same extent as NK15/2 cells; however, the percentages of circulating KIR+ NK15/12 cells were significantly higher than those of NK15/2 cells (17.3 ± 0.3 versus 40.9 ± 4.0%, respectively) resulting in higher frequencies of KIR+CD16+ cells in the spleen (), as well as in the liver and bone marrow of infused mice (data not shown). To further characterize the kinetics of NK cell maturation in vivo, HPC-NK cells were infused into NSG mice and phenotyped 1, 7, or 14 d later. Remarkably, the percentages of CD16 and KIR single-positive cells following infusion of NK15/12 cells remained stable over time (). In contrast, the proportion of CD16+KIR+ NK cells strongly increased to progressively replace CD16−KIR− NK cells, suggesting concomitant acquisition of CD16 and KIR expression on immature HPC-NK cells in vivo. In line with this, no difference in proliferation capacity between NK cell subsets was found (data not shown). Moreover, CD16 and KIR acquisition profiles on NK15/12 cells appeared to be similar in the liver (Fig. S5) and the BM (data not shown) of infused mice. Notably, increasing frequencies of CD16+KIR+ cells were also observed following NK15/2 cell infusion, but to a significantly lower extent compared to NK15/12 cells (, Fig. S5). Although a discrete NKG2A−KIR+ NK cell population appeared by day 7–14, expression of NKG2A remained high with >70% positivity at all time points (). Together, these data indicate that, in addition to sustained expression of activating receptors, HPC-NK cells generated with IL-15 and IL-12 undergo rapid in vivo maturation that might favor their alloreactivity toward AML cells following adoptive transfer in situations of KIR-ligand mismatches.
Figure 4. HPC-NK cell products generated in the presence of IL-12 display faster maturation in vivo. NK15/2 or NK15/12 cells were infused into adult NSG mice (5 × 10Citation6) in combination with low-dose IL-15 support. One week later, mice were sacrificed and ex vivo flow cytometric analysis was performed on cells isolated from spleen, liver, and bone marrow (BM). (A) Representative expression of the activating receptors NKG2D, NKp46, and DNAM-1 as well as CXCR3 on NK15/2 and NK15/12 cells before infusion and isolated from spleen 1 week after adoptive transfer. (B) Percentages of CD16- and KIR-expressing NK cells before infusion and 1 week after adoptive transfer. Combined results from 2 experiments (n = 4–6 mice per group per experiment) are shown as means ± SEM. **P < 0.01, 2-way ANOVA. (C) Representative dot plots of CD16 and KIR expression on CD56+ cells isolated from spleen of mice injected with NK15/2 or NK15/12 cells.
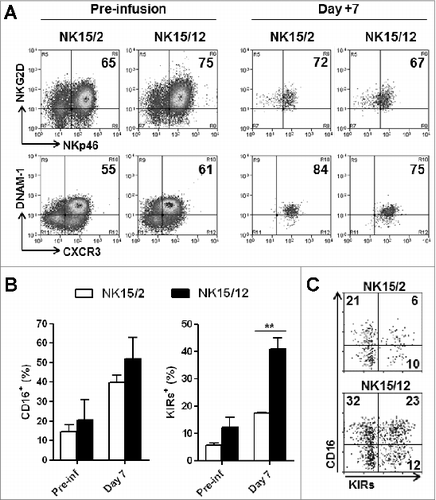
Figure 5. NK15/12 cells undergo concomitant CD16 and KIR acquisition in vivo with sustained NKG2A expression. HPC-NK cells (5 × 106) were infused into adult NSG mice in combination with IL-15 support. Ex vivo flow cytometric analysis was performed on cells isolated 1, 7, and 14 d after adoptive transfer (n = 4 per time point). (A) Percentages of splenic NK cell subsets identified using CD16 and KIR differentiation markers over time. **P < 0.01, ***P < 0.001, one-way ANOVA. (B) Percentages of liver CD16+KIR+ cells following infusion of NK15/2 versus NK15/12 cell products. *P < 0.05, ***P < 0.001, 2-way ANOVA. (C) Percentages (mean ± SD) of NK cell subsets identified using NKG2A and KIR differentiation markers over time. (D) Representative dot plots of KIR, NKG2A, and CD16 expression on human CD56+ cells of the infused product and splenocytes isolated at day 1 and 14.
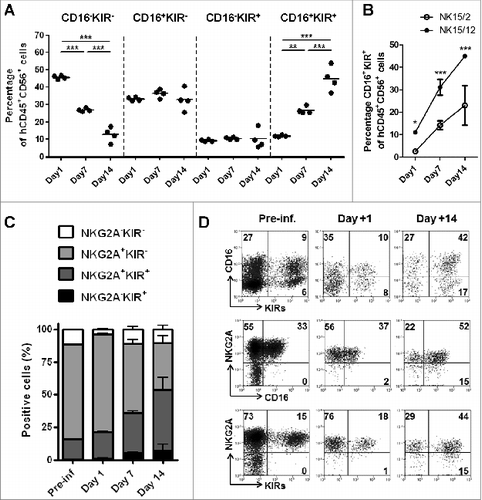
Infusion of NK15/12 cell products improves antileukemic response in MHC-I+ AML-bearing mice
To further compare the antileukemic potential of NK15/2 versus NK15/12 cells, adoptive transfer studies were designed in adult NSG mice injected in the right femur with luciferase-expressing leukemia cells (day 0). We previously reported that HPC-NK cells generated in the presence of IL-15 and IL-2 efficiently inhibited progression of BM-residing K562 cells in vivo.Citation26 Using this model, a single infusion of NK15/2 or NK15/12 cells exerted a similar effect (). Therefore, new studies were conducted in a more stringent model using the MHC class I+ THP-1 cells and with infusion of HPC-NK cells generated from partially KIR/ligand mismatched donors. Using the same experimental design, the effect of a single HPC-NK cell infusion was limited by the aggressive and fast progression of THP-1 cells in vivo (Fig. S6A-B). Nevertheless, progression of THP-1 cells was significantly reduced after 2 weeks following treatment with NK15/12 cells (Fig. S6C). Therefore, to slow down THP-1 cell progression and prolong the treatment period, conditioning of THP1-bearing mice using cyclophosphamide was introduced in our study, and an equivalent amount of HPC-NK cells was administered as 3 infusions at 3- to 4-day intervals (). In line with previous findings, the antileukemic effect of NK cell infusions was evident from day 10 onwards. More importantly, treatment with NK15/12 cells significantly reduced tumor load compared to NK15/2 cell products up to day 21 (). Analysis of peripheral blood confirmed NK cell persistence for up to 2 weeks after adoptive transfer and showed similar levels of circulating NK cells in both groups of mice (data not shown). These data demonstrate that infusion of NK15/12 cell products improves antileukemic responses in MHC-I+ AML cell-bearing hosts and support the notion that combining IL-15 with IL-12 favors HPC-NK cells alloreactivity against KIR/ligand mismatched AML cells in vivo.
Figure 6. Infusion of NK15/12 cell products improves anti-leukemic response in THP1-bearing NSG mice. (A, B) In vivo antileukemic potential of a single infusion of NK15/2 versus NK15/12 cells was compared in adult NSG mice injected in the right femur with K562.LucGFP cells. (A) Experimental study design in K562-bearing mice, and (B) tumor load over time (mean ± SD, n=6 per group, 2-way ANOVA). (C-E) Effect of multiple NK cell infusions in cyclophosphamide-conditioned mice. Data from one experiment in which HPC-NK cells were generated using SCGM and selected for partial KIR/ligand mismatch (UCB HLA type: Bw4, C1/C2, THP-1 HLA type: Bw6, C1/C1) are shown (n=8–9 per group). (C) Experimental study design. (D) Tumor load progression overview. Mean ± SD, 2-way ANOVA with repeated measures. (E) Fold increase in tumor load between day 6 and day 21. Mean ± SD, one-way ANOVA. *P < 0.05, **P < 0.01.
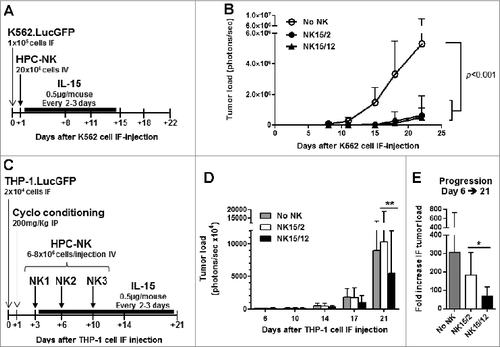
Discussion
In the past decade, adoptive transfer of allogeneic NK cells has attracted attention as a promising adjuvant treatment approach against cancer including AML. Many reports have described new methodologies to achieve higher NK cell number and activation. In particular, expansion and differentiation of HPCs allows the generation of clinically applicable NK cell products with high purity and functionality.Citation25 Nevertheless, we and others have observed that NK cells generated under stroma-free culture conditions using IL-15 and IL-2 have low surface expression of CD16 and only a subset (5–10%) express KIRs,Citation23,37 raising questions about NK cell potency and alloreactivity following adoptive transfer. Moreover, clinical studies reported so far show that adoptively transferred allogeneic NK cells have a relatively short lifespan in patients. In view of the transient elevation of endogenous IL-15, which facilitates in vivo expansion after lymphodepleting chemotherapy as well as host immune T-cell recovery and consequent rejection of the partially HLA-matched allogeneic NK cells, it might be beneficial to maximize NK cell alloreactivity toward AML shortly after adoptive transfer.
In the present study, we demonstrated that more mature and highly functional HPC-NK cells could be generated under stroma-free conditions by replacement of IL-2 for IL-12. Importantly, NK15/12 cells showed higher cytolytic activity against both cultured and patient-derived primary AML cells. Although IL-12 reduces the cell expansion rate during the differentiation phase, high numbers of HPCs can be isolated from UCB units and efficiently expanded using our culture process, thereby allowing generation of clinically-relevant doses of NK cells to treat patients. In addition, we showed that IL-12 favors the generation of CD16- and KIR-expressing NK cells, resulting in the rapid development of hyper-responsive CD16+KIR+NK cells following adoptive transfer. In line with the study of Hsu and colleagues demonstrating that NK cell responsiveness follows a clear hierarchy with respect to NKG2A and inhibitory KIR expression pattern,Citation38 we foresee that faster maturation potential of NK15/12 cells in vivo will trigger more potent HPC-NK cell alloreactivity toward AML. Accordingly, superior antileukemic activity of NK15/12 cell products was demonstrated in vivo in situations of partial KIR–ligand mismatch. Moreover, a recent study reported that hyporesponsiveness of unlicensed NK cells lacking KIR expression for self-HLA could be reversed upon CD16 engagement.Citation39 This suggests that concomitant CD16 and KIR acquisition observed among NK15/12 cells will favor HPC-NK cell potency and provides a rationale for exploring allogeneic HPC-NK cell adoptive transfer in combination with therapeutic antibodies to exploit tumor-targeted antibody-dependent cell-mediated cytotoxicity.
Consistent with a known potentiating effect of IL-12 on NK cells,Citation27 we also demonstrated higher IFNγ production capacity of NK15/12 cell products compared to IL-15/IL-2–generated cells. Expression of the IL-12 receptor CD212 was detected early on NK progenitor cells and throughout ex vivo NK cell differentiation (data not shown), indicating that in our system IL-12 has a direct effect on HPC-NK cell functions. Notably, use of IL-12 in combination with IL-2 during the differentiation phase also increased the functionalities of HPC-NK cell products (data not shown); however, this cytokine combination had a stronger impact on ex vivo NK cell expansion compared to IL-12 alone and also resulted in limited increased KIR expression (data not shown), suggesting that both IL-12 and IL-2 influence NK cell maturation and KIR acquisition. Likewise, previous studies have described that exposure to IL-12 resulted in upregulation of CD62L on NK cells,Citation40 and, more recently, that expression of CD62L identified a unique subset of polyfunctional NK cells with enhanced IFNγ production and proliferation capacity upon viral infection in vivoCitation41. In the present study, although increased frequencies of CD62L+ cells were observed using IL-12, differences in functionality could not be identified within this subset. Therefore, the mechanisms by which IL-12 influences NK cell maturation and/or proliferation should be further investigated.
In conclusion, we showed that replacement of IL-2 by IL-12 in our culture process allows generation of HPC-NK cell products with enhanced IFNγ production and cytolytic activity toward AML, as well as superior maturation potential and antileukemic activity in vivo. We foresee that higher functionality and faster CD16 and KIR acquisition will trigger more potent HPC-NK cell alloreactivity toward malignant cells in patients, making the combination of IL-15 and IL-12 an attractive strategy to generate clinical HPC-NK cell products for cancer adoptive immunotherapy.
Materials and Methods
HPC-NK cell generation
UCB units were obtained at birth after normal full-term delivery from the cord blood bank of the Radboudumc with written informed consent regarding their scientific use. NK cells were generated from cryopreserved UCB-derived HPCs as previously reported.Citation23,26 Briefly, expanded CD34+ cells were differentiated and further expanded using NK cell differentiation medium consisting of Glycostem Basal Growth Medium (GBGM®) for cord blood (Clear Cell Technologies) supplemented with 2% human serum (HS; Sanquin Blood Supply Foundation, Nijmegen, the Netherlands), 250 pg/mL G-CSF (Neupogen®; Amgen), 10 pg/mL GM-CSF, 50 pg/mL IL-6 (both CellGenix), and a high-dose cytokine cocktail consisting of 20 ng/ml IL-7, SCF, IL-15 (all CellGenix) and 1000 U/mL IL-2 (Proleukin®; Chiron). IL-12 (Immunotools, specific activity of 3 × 106 U/mg) was used at a concentration of 200 pg/mL in conjunction with IL-15, with or without IL-2. Where indicated in the figure legends, HPC-NK cells were generated using the alternative Stem Cell Growth Medium (SCGM, Cellgenix) with the following modifications to the cytokine cocktail: 50 ng/mL IL-15 and 70 pg/mL IL-12 (Miltenyi Biotech, specific activity of 1 × 106 U/mg). The cell density was checked twice a week and adjusted to >1.5 × 106 cells/ml by the addition of NK cell differentiation medium. For experiments, CD56+CD3− HPC-NK cells were used at the end of the culture process with >80% purity, which was typically achieved within 3–4 weeks in NK cell differentiation medium.
Cell lines and reagents
The K562, THP-1, and KG-1a cell lines (ATCC) were cultured in Iscove's modified Dulbecco's medium (IMDM; Invitrogen) containing 50 U/mL penicillin, 50 µg/mL streptomycin and 10% fetal calf serum (FCS; Integro). Recombinant human IFNγ and TNFα (both 2 × 107 U/mg; Immunotools) were used at the concentrations indicated in the figure legends.
Flow cytometry
Anti-human CD45 (J33; Beckman Coulter) and CD56 (HCD56; Biolegend) antibodies were used to follow cell number and NK cell differentiation during culture using the Coulter FC500 flow cytometer (Beckman Coulter). The population of living CD45+ cells was determined by exclusion of 7AAD-positive cells (Sigma). Phenotypic analysis was performed using Coulter FC500 or Cyan-ADP 9 color cytometers (Beckman Coulter) and conjugated monoclonal antibodies against the following proteins: CD3 and NKG2A (clones UCHT1 and Z199 respectively, both from Beckman Coulter); CD158a/h, b and e (clones HP-MA4, DX27, and DX9 respectively, used as a combined staining for inhibitory KIRs); CD16 (3G8), CD62L (DREG-56), CXCR3 (G025H7), CXCR4 (12G5), NKG2D (1D11), NKp46 (9E2), TRAIL (RIK-2), FasL (NOK-1; all Biolegend); and DNAM-1 (DX11; BD Bioscience).
Cellular assays
Flow cytometry-based cytotoxicity assays were performed as described previously with minor modifications.Citation23 Briefly, CFSE-labeled target cells were resuspended in IMDM/10% FCS to a final concentration of 1–3 × 105/mL. HPC-NK cells were washed with PBS and resuspended in GBGM/2% HS to a final concentration of 1–10 × 105/mL. Target cells were co-cultured with effector cells at different E:T ratios in 96-well flat-bottomed plates. NK and target cells alone were plated as controls. After overnight incubation at 37 °C, cells were harvested and the number of viable target cells was quantified by flow cytometry. Target cell survival was calculated as follows: % survival = (absolute number of viable CFSE+ target cells co-cultured with NK cells / absolute number of viable CFSE+ target cells cultured in medium)*100. The percentage of specific killing was 100 minus % survival. Co-cultures of NK with primary AML cells were supplemented with IL-3 (50 ng/mL; Cellgenix), SCF (20 ng/mL; Immunotools), Flt3L (20 ng/mL; Cellgenix), G-CSF (20 ng/mL; Amgen), and IL-15 (5 ng/mL). ICAM-1 blocking antibody (clone SM89) was used at the dilution of 1:100. NK cell degranulation and IFNγ production were determined upon stimulation with K562 cells at an E:T ratio of 1:1. Anti-CD107a (H4A3; BD Biosciences) was added at a 1:100 dilution at the start of the co-culture, and brefeldin A (1 ng/µL, BD Biosciences) was added 1 h later. Cells were collected the following day, stained for cell surface markers, and then treated with fixation/permeabilization buffer (eBioscience) and stained for intracellular IFNγ (clone B27; BD Biosciences). Flow cytometric analysis was performed with exclusion of dead cells with Fixable Viability Dye (eBioSciences) and using unstimulated cells as a control.
Cytokine production analysis
Cytokine production by target cell-stimulated NK cells was measured in the supernatant of the co-cultures by enzyme-linked immunosorbent assay (ELISA) according to the manufacturer's instructions. In these assays, a fixed number of 5 × 104 HPC-NK cells were plated with target cells at an E:T ratio of 1:1 or 2:1, as indicated in the figure legend, in a total volume of 200 µL. ELISA kits for IFNγ, TNFα, and granzyme B were purchased from Pierce Endogen, Mabtech, and Sanquin respectively, and the TMB microwell peroxidase substrate system was from KPL. Absorbance was measured at 450 nm with a Multiscan MCC/340 ELISA reader (Titertek).
RNA extraction and real-time PCR quantification
Total RNA was isolated using Quick-RNA Miniprep kit (Zymo Research) according to the manufacturer's instructions. cDNA was synthesized using M-MLV-reverse transcriptase (Invitrogen) in a standard reaction. Real-time PCR was performed using Taqman Gene expression assays (Applied Biosystems): PRF1 (Hs99999108); GZMB (Hs01554355); EOMES (Hs00172872); TBX21 (Hs00203436). Expression levels were calculated relative to GAPDH (Hs02758991) using the ΔΔCt method.
HPC-NK cell adoptive transfer, intra-femoral THP-1 model, and bioluminescence imaging
NSG mice originally purchased from Jackson Laboratories were housed and bred in the Radboudumc Central Animal Laboratory. NSG mice were aged 6 to 12 weeks at the start of the experiment (weight 20–30 g). All animal experiments were approved by the Animal Experimental Committee of the Radboudumc and conducted in accordance with institutional and national guidelines under the university permit number 10300. In adoptive transfer studies, HPC-NK cells were resuspended in PBS and injected intravenously via the tail vein. Recombinant human IL-15 (Miltenyi Biotech) was administrated subcutaneously at a dose of 0.5 μg (2,500 units) per mouse every 2–3 d starting the day of HPC-NK cell infusion. For ex vivo flow cytometric analysis, mice were sacrificed and cells were isolated from spleen, bone marrow, and liver using erythrocyte lysis solution or lympholyte-M (Cedarlane). Preclinical xenograft models were established using K562 and THP-1 cells previously engineered to express the GFP and luciferase reporter genes (K562.GFPLuc and THP1.GFPLuc). Efficient AML cell engraftment was achieved using 1 × 105 K562 and 2 × 104 THP-1 cells injected in the right femur of isoflurane gas-anesthetized mice (injection volume = 5 μL). Tumor load was monitored by bioluminescence imaging (BLI) as previously described.Citation26
Statistical analysis
Statistical analyses were performed using Graphpad Prism 5 software. Student t-tests and 1- and 2-way analysis of variance (ANOVA) were used as appropriate as indicated in the figure legends. Differences were considered to be significant for P values <0.05.
Disclosure of Potential Conflicts of Interest
The work has been performed in collaboration with Glycostem Therapeutics, a life-science company in the Netherlands. The authors Jan Spanholtz and Marleen Tordoir are employees of Glycostem Therapeutics that worked in the laboratory of Harry Dolstra, who acted as scientific consultant. Other authors have no conflict of interest to declare.
Author Contributions
JC designed and performed experiments, analyzed data and wrote the manuscript; AQ performed experiments and analyzed data; JS and MT provided instructions for applying GBGM® expansion / differentiation technology and assisted in UCB-NK cell culture; JJ, RV, and NS provided advice; HD supervised research. All authors revised and approved the manuscript.
2014ONCOIMM0420R-f07-z-bw.pdf
Download PDF (1.1 MB)Acknowledgments
We thank Rob Woestenenk and colleagues from the flow cytometry facility (Radboudumc) for technical support and cell sorting. We also thank Jos Paardekooper and other analysts from the laboratory of Hematology (Radboudumc), as well as the team of the animal facility.
Funding
This work was supported by ZonMW 11600101, Vanderes Foundation 2009–2016, RUMC-RIO 2011, KWF KUN2014-6701, and the Radboudumc.
References
- Geller MA, Miller JS. Use of allogeneic NK cells for cancer immunotherapy. Immunotherapy 2011; 3:1445-59; PMID:22091681; http://dx.doi.org/10.2217/imt.11.131
- Velardi A. Role of KIRs and KIR ligands in hematopoietic transplantation. Curr Opin Immunol 2008; 20:581-7; PMID:18675345; http://dx.doi.org/10.1016/j.coi.2008.07.004
- Moretta A, Locatelli F, Moretta L. Human NK cells: from HLA class I-specific killer Ig-like receptors to the therapy of acute leukemias. Immunol Rev 2008; 224:58-69; PMID:18759920; http://dx.doi.org/10.1111/j.1600-065X.2008.00651.x
- Pegram HJ, Ritchie DS, Smyth MJ, Wiernik A, Prince HM, Darcy PK, Kershaw MH. Alloreactive natural killer cells in hematopoietic stem cell transplantation. Leuk Res 2011; 35:14-21; PMID:20719383; http://dx.doi.org/10.1016/j.leukres.2010.07.030
- Ruggeri L, Capanni M, Urbani E, Perruccio K, Shlomchik WD, Tosti A, Posati S, Rogaia D, Frassoni F, Aversa F, et al. Effectiveness of donor natural killer cell alloreactivity in mismatched hematopoietic transplants. Science 2002; 295:2097-100; PMID:11896281; http://dx.doi.org/10.1126/science.1068440
- Ruggeri L, Mancusi A, Capanni M, Urbani E, Carotti A, Aloisi T, Stern M, Pende D, Perruccio K, Burchielli E, et al. Donor natural killer cell allorecognition of missing self in haploidentical hematopoietic transplantation for acute myeloid leukemia: challenging its predictive value. Blood 2007; 110:433-40; PMID:17371948; http://dx.doi.org/10.1182/blood-2006-07-038687
- Willemze R, Rodrigues CA, Labopin M, Sanz G, Michel G, Socie G, Rio B, Sirvent A, Renaud M, Madero L, et al. KIR-ligand incompatibility in the graft-versus-host direction improves outcomes after umbilical cord blood transplantation for acute leukemia. Leukemia 2009; 23:492-500; PMID:19151783; http://dx.doi.org/10.1038/leu.2008.365
- Miller JS, Soignier Y, Panoskaltsis-Mortari A, McNearney SA, Yun GH, Fautsch SK, McKenna D, Le C, Defor TE, Burns LJ, et al. Successful adoptive transfer and in vivo expansion of human haploidentical NK cells in patients with cancer. Blood 2005; 105:3051-7; PMID:15632206; http://dx.doi.org/10.1182/blood-2004-07-2974
- Curti A, Ruggeri L, D'Addio A, Bontadini A, Dan E, Motta MR, Trabanelli S, Giudice V, Urbani E, Martinelli G, et al. Successful transfer of alloreactive haploidentical KIR ligand-mismatched natural killer cells after infusion in elderly high risk acute myeloid leukemia patients. Blood 2011; 118:3273-9; PMID:21791425; http://dx.doi.org/10.1182/blood-2011-01-329508
- Rubnitz JE, Inaba H, Ribeiro RC, Pounds S, Rooney B, Bell T, Pui CH, Leung W. NKAML: a pilot study to determine the safety and feasibility of haploidentical natural killer cell transplantation in childhood acute myeloid leukemia. J Clin Oncol: Off J Am Soc Clin Oncol 2010; 28:955-9; PMID:20085940; http://dx.doi.org/10.1200/JCO.2009.24.4590
- Luhm J, Brand JM, Koritke P, Hoppner M, Kirchner H, Frohn C. Large-scale generation of natural killer lymphocytes for clinical application. J Hematother Stem Cell Res 2002; 11:651-7; PMID:12201953; http://dx.doi.org/10.1089/15258160260194794
- Passweg JR, Stern M, Koehl U, Uharek L, Tichelli A. Use of natural killer cells in hematopoetic stem cell transplantation. Bone Marrow Transplant 2005; 35:637-43; PMID:15654351; http://dx.doi.org/10.1038/sj.bmt.1704810
- McKenna DH Jr, Sumstad D, Bostrom N, Kadidlo DM, Fautsch S, McNearney S, Dewaard R, McGlave PB, Weisdorf DJ, Wagner JE, et al. Good manufacturing practices production of natural killer cells for immunotherapy: a six-year single-institution experience. Transfusion 2007; 47:520-8; PMID:17319835; http://dx.doi.org/10.1111/j.1537-2995.2006.01145.x
- Meyer-Monard S, Passweg J, Siegler U, Kalberer C, Koehl U, Rovo A, Halter J, Stern M, Heim D, Alois Gratwohl JR, et al. Clinical-grade purification of natural killer cells in haploidentical hematopoietic stem cell transplantation. Transfusion 2009; 49:362-71; PMID:19389215; http://dx.doi.org/10.1111/j.1537-2995.2008.01969.x
- Carlens S, Gilljam M, Chambers BJ, Aschan J, Guven H, Ljunggren HG, Christensson B, Dilber MS. A new method for in vitro expansion of cytotoxic human CD3-CD56+ natural killer cells. Hum Immunol 2001; 62:1092-8; PMID:11600215; http://dx.doi.org/10.1016/S0198-8859(01)00313-5
- Klingemann HG, Martinson J. Ex vivo expansion of natural killer cells for clinical applications. Cytotherapy 2004; 6:15-22; PMID:14985163; http://dx.doi.org/10.1080/14653240310004548
- Koehl U, Esser R, Zimmermann S, Tonn T, Kotchetkov R, Bartling T, Sörensen J, Grüttner HP, Bader P, Seifried E, et al. Ex vivo expansion of highly purified NK cells for immunotherapy after haploidentical stem cell transplantation in children. Klin Padiat 2005; 217:345-50; PMID:16307421; http://dx.doi.org/10.1055/s-2005-872520
- Berg M, Lundqvist A, McCoy P Jr, Samsel L, Fan Y, Tawab A, Childs R. Clinical-grade ex vivo-expanded human natural killer cells up-regulate activating receptors and death receptor ligands and have enhanced cytolytic activity against tumor cells. Cytotherapy 2009; 11:341-55; PMID:19308771; http://dx.doi.org/10.1080/14653240902807034
- Tanaka J, Sugita J, Shiratori S, Shigematu A, Asanuma S, Fujimoto K, Nishio M, Kondo T, Imamura M. Expansion of NK cells from cord blood with antileukemic activity using GMP-compliant substances without feeder cells. Leukemia 2012; 26:1149-52; PMID:22143670; http://dx.doi.org/10.1038/leu.2011.345
- Lim O, Lee Y, Chung H, Her JH, Kang SM, Jung MY, Min B, Shin H, Kim TM, Heo DS, et al. GMP-compliant, large-scale expanded allogeneic natural killer cells have potent cytolytic activity against cancer cells in vitro and in vivo. PloS One 2013; 8:e53611; PMID:23326467; http://dx.doi.org/10.1371/journal.pone.0053611
- Kao IT, Yao CL, Kong ZL, Wu ML, Chuang TL, Hwang SM. Generation of natural killer cells from serum-free, expanded human umbilical cord blood CD34+ cells. Stem Cells Dev 2007; 16:1043-51; PMID:17999637; http://dx.doi.org/10.1089/scd.2007.0033
- Woll PS, Grzywacz B, Tian X, Marcus RK, Knorr DA, Verneris MR, Kaufman DS. Human embryonic stem cells differentiate into a homogeneous population of natural killer cells with potent in vivo antitumor activity. Blood 2009; 113:6094-101; PMID:19365083; http://dx.doi.org/10.1182/blood-2008-06-165225
- Spanholtz J, Tordoir M, Eissens D, Preijers F, van der Meer A, Joosten I, Schaap N, de Witte TM, Dolstra H. High log-scale expansion of functional human natural killer cells from umbilical cord blood CD34-positive cells for adoptive cancer immunotherapy. PloS One 2010; 5:e9221; PMID:20169160; http://dx.doi.org/10.1371/journal.pone.0009221
- Yoon SR, Lee YS, Yang SH, Ahn KH, Lee JH, Lee JH, Kim DY, Kang YA, Jeon M, Seol M, et al. Generation of donor natural killer cells from CD34(+) progenitor cells and subsequent infusion after HLA-mismatched allogeneic hematopoietic cell transplantation: a feasibility study. Bone Marrow Transplant 2010; 45:1038-46; PMID:19881555; http://dx.doi.org/10.1038/bmt.2009.304
- Spanholtz J, Preijers F, Tordoir M, Trilsbeek C, Paardekooper J, de Witte T, Schaap N, Dolstra H. Clinical-grade generation of active NK cells from cord blood hematopoietic progenitor cells for immunotherapy using a closed-system culture process. PloS One 2011; 6:e20740; PMID:21698239; http://dx.doi.org/10.1371/journal.pone.0020740
- Cany J, van der Waart AB, Tordoir M, Franssen GM, Hangalapura BN, de Vries J, Boerman O, Schaap N, van der Voort R, Spanholtz J, et al. Natural killer cells generated from cord blood hematopoietic progenitor cells efficiently target bone marrow-residing human leukemia cells in NOD/SCID/IL2Rg(null) mice. PloS One 2013; 8:e64384; PMID:23755121; http://dx.doi.org/10.1371/journal.pone.0064384
- Trinchieri G. Interleukin-12 and the regulation of innate resistance and adaptive immunity. Nat Rev Immunol 2003; 3:133-46; PMID:12563297; http://dx.doi.org/10.1038/nri1001
- Sun JC, Beilke JN, Bezman NA, Lanier LL. Homeostatic proliferation generates long-lived natural killer cells that respond against viral infection. J Exp Med 2011; 208:357-68; PMID:21262959; http://dx.doi.org/10.1084/jem.20100479
- Lehmann D, Spanholtz J, Sturtzel C, Tordoir M, Schlechta B, Groenewegen D, Hofer E. IL-12 directs further maturation of ex vivo differentiated NK cells with improved therapeutic potential. PloS One 2014; 9:e87131; PMID:24498025; http://dx.doi.org/10.1371/journal.pone.0087131
- Cooper MA, Elliott JM, Keyel PA, Yang L, Carrero JA, Yokoyama WM. Cytokine-induced memory-like natural killer cells. Proc Natl Acad Sci U S A 2009; 106:1915-9; PMID:19181844; http://dx.doi.org/10.1073/pnas.0813192106
- Romee R, Schneider SE, Leong JW, Chase JM, Keppel CR, Sullivan RP, Cooper MA, Fehniger TA. Cytokine activation induces human memory-like NK cells. Blood 2012; 120:4751-60; PMID:22983442; http://dx.doi.org/10.1182/blood-2012-04-419283
- Sun JC, Madera S, Bezman NA, Beilke JN, Kaplan MH, Lanier LL. Proinflammatory cytokine signaling required for the generation of natural killer cell memory. J Exp Med 2012; 209:947-54; PMID:22493516; http://dx.doi.org/10.1084/jem.20111760
- Townsend MJ, Weinmann AS, Matsuda JL, Salomon R, Farnham PJ, Biron CA, Gapin L, Glimcher LH. T-bet regulates the terminal maturation and homeostasis of NK and Valpha14i NKT cells. Immunity 2004; 20:477-94; PMID:15084276; http://dx.doi.org/10.1016/S1074-7613(04)00076-7
- Gill S, Vasey AE, De Souza A, Baker J, Smith AT, Kohrt HE, Florek M, Gibbs KD Jr, Tate K, Ritchie DS, et al. Rapid development of exhaustion and down-regulation of eomesodermin limit the antitumor activity of adoptively transferred murine natural killer cells. Blood 2012; 119:5758-68; PMID:22544698; http://dx.doi.org/10.1182/blood-2012-03-415364
- Wang R, Jaw JJ, Stutzman NC, Zou Z, Sun PD. Natural killer cell-produced IFN-gamma and TNF-alpha induce target cell cytolysis through up-regulation of ICAM-1. J Leukocyte Biol 2012; 91:299-309; PMID:22045868; http://dx.doi.org/10.1189/jlb.0611308
- Wendel M, Galani IE, Suri-Payer E, Cerwenka A. Natural killer cell accumulation in tumors is dependent on IFN-gamma and CXCR3 ligands. Cancer Res 2008; 68:8437-45; PMID:18922917; http://dx.doi.org/10.1158/0008-5472.CAN-08-1440
- Dezell SA, Ahn YO, Spanholtz J, Wang H, Weeres M, Jackson S, Cooley S, Dolstra H, Miller JS, Verneris MR. Natural killer cell differentiation from hematopoietic stem cells: a comparative analysis of heparin- and stromal cell-supported methods. Biol Blood marrow Transplant: J Am Soc Blood Marrow Transplant 2012; 18:536-45; PMID:22155502; http://dx.doi.org/10.1016/j.bbmt.2011.11.023
- Yu J, Heller G, Chewning J, Kim S, Yokoyama WM, Hsu KC. Hierarchy of the human natural killer cell response is determined by class and quantity of inhibitory receptors for self-HLA-B and HLA-C ligands. J Immunol 2007; 179:5977-89; PMID:17947671; http://dx.doi.org/10.4049/jimmunol.179.9.5977
- Tarek N, Le Luduec JB, Gallagher MM, Zheng J, Venstrom JM, Chamberlain E, Modak S, Heller G, Dupont B, Cheung NK, et al. Unlicensed NK cells target neuroblastoma following anti-GD2 antibody treatment. J Clin Invest 2012; 122:3260-70; PMID:22863621; http://dx.doi.org/10.1172/JCI62749
- Frey M, Packianathan NB, Fehniger TA, Ross ME, Wang WC, Stewart CC, Caligiuri MA, Evans SS. Differential expression and function of L-selectin on CD56bright and CD56dim natural killer cell subsets. J Immunol 1998; 161:400-8
- Juelke K, Killig M, Luetke-Eversloh M, Parente E, Gruen J, Morandi B, Ferlazzo G, Thiel A, Schmitt-Knosalla I, Romagnani C. CD62L expression identifies a unique subset of polyfunctional CD56dim NK cells. Blood 2010; 116:1299-307; PMID:20505160; http://dx.doi.org/10.1182/bloxod-2009-11-253286