ABSTRACT
Radiation therapy (RT) can induce upregulation of programmed death ligand 1 (PD-L1) on tumor cells or myeloid cells, which may affect response to PD-1-based immunotherapy. PD-L1 upregulation during RT is a dynamic process that has been difficult to monitor during treatment. The aim of this study was to evaluate the RT-induced PD-L1 upregulation in the tumor and its microenvironment using immunoPET/CT imaging of two syngeneic murine tumor models (HPV+ head and neck squamous cell carcinoma (HNSCC) or B16F10 melanoma). Tumors were established in two locations per mouse (neck and flank), and fractionated RT (2 Gy × 4 or 2 Gy × 10) was delivered only to the neck tumor, alone or during anti-PD-1 mAb immunotherapy. PD-L1 expression was measured by PET/CT imaging using Zr-89 labeled anti-mouse PD-L1 mAb, and results were validated by flow cytometry. PET/CT imaging demonstrated significantly increased tracer uptake in irradiated neck tumors compared with non-irradiated flank tumors. Ex vivo analysis by biodistribution and flow cytometry validated PD-L1 upregulation specifically in irradiated tumors. In the HNSCC model, RT-induced PD-L1 upregulation was only observed after 2 Gy × 10 fractionated RT, while in the B16F10 model upregulation of PD-L1 occurred after 2 Gy × 4 fractionated RT. Fractionated RT, but not anti-PD-1 therapy, upregulated PD-L1 expression on tumor and infiltrating inflammatory cells in murine models, which could be non-invasively monitored by immunoPET/CT imaging using Zr-89 labeled anti-mouse PD-L1 mAb, and differentially identified anti-PD-1 responsive as well as selectively irradiated tumors in vivo.
Introduction
Recent success of immune checkpoint receptor immunotherapy in several cancersCitation1,2 has motivated its use in locally advanced, curative disease stages, often in conjunction with conventional therapy. Combining programmed death (PD)-1/programmed death ligand 1 (PD-L1) blockade with radiation therapy (RT) may lead to better outcomes than RT alone,Citation3-5 which is being tested in the clinic. The mechanisms involved in this synergy have not been clarified. However, PD-L1 is a frequently used biomarker for anti-PD-1-based immunotherapy. It has been reported that surface PD-L1 expression levels on some cancers are upregulated after a single high dose of RTCitation4 or lower doses of fractionated RT.Citation3 These studies suggest that RT may reactivate the host immunity and that RT-induced PD-L1 upregulation could be a predictive marker for response to the combination of anti-PD-1/PD-L1 blockade with RT.
PD-L1 expression is heterogeneous within the tumor and its microenvironmentCitation6-8 and expression patterns likely change during different types of therapy.Citation9,10 This heterogeneity is difficult to evaluate in single, baseline tumor biopsies due to the dynamic variability in PD-L1 expression levels across the entire tumor. Non-invasive imaging of tumor PD-L1 expression and kinetics could be quite helpful, providing information regarding PD-L1 expression and changes in different tissues and tumor sites, as opposed to relying on biopsies from localized regions of a tumor or organ. Additionally, positron emission tomography/computed tomography (PET/CT) imaging could provide assessment of PD-L1-positive tumor cells in lymph nodes as well as visualize metastases.
Preclinical immunoPET/CT has emerged as an important tool to evaluate the dynamics and levels of receptor expression in experimental animal models. Previous reports on PET imaging of PD-L1 used a variety of anti-mouse and anti-human monoclonal antibodies (mAbs),Citation11,12 high-affinity consensus (HAC) PD-1,Citation13 or PD-L1 binding peptide Citation14 labeled with either Cu-64 or Zr-89. However, these studies were performed in mice of varying immune competence, which limited the outcomes to validation of the tracers in terms of specificity. In a novel approach that is analogous to current clinical practice, we quantified the extent of RT-induced PD-L1 upregulation in two different tumor models by immunoPET/CT, since RT is increasingly used in combination with anti-PD-1 immunotherapy in the clinic. Using a Zr-89-labeled anti-mouse PD-L1 mAb and immuno-PET/CT, we analyzed the dynamics of RT-induced effects on PD-L1 expression levels in HPV+ head and neck squamous cell carcinoma (HNSCC) and B16F10 melanoma tumors in mice. The ultimate goal is to perform clinical imaging of PD-L1, where the high sensitivity and resolution of immunoPET/CT will be useful for diagnosis and treatment planning, as well as monitoring therapy.Citation15 Quantification of PD-L1 expression in tumors by PET/CT in the clinic has great potential for optimizing the radiotherapy strategy (i.e. determining RT alone or RT combined with immune checkpoint inhibitor(s)). We anticipate that during RT therapy, PET/CT will be sensitive to dynamic and heterogeneous PD-L1 expression patterns that are beyond the capacity of traditional immunohistochemistry (IHC) to accurately assess.
By performing PD-L1-targeted PET/CT imaging before and during RT alone or with PD-1-based immunotherapy, we could select appropriate patients with high PD-L1 expression or those with upregulation after initiation of local stimuli. In addition, non-invasive imaging presents an opportunity to alter the therapeutic strategy during the course of RT by altering the combination of immunotherapeutic or conventional agents to enhance immunogenicity.
Results
RT-induced PD-L1 upregulation on MEER or B16F10 cells in vitro
To determine the effect of RT on tumor cell PD-L1 expression, MEER and B16F10 cells were treated with a single fraction of 2 Gy or 10 Gy in vitro (Fig. S1). At baseline, PD-L1 expression of MEER cells was found to be significantly lower than that of B16F10 cells (p < 0.0001). PD-L1 expression of MEER cells was significantly upregulated 1 d after 2 Gy or 10 Gy of RT (p < 0.05 for both doses) and this upregulation persisted until day 3. PD-L1 expression of B16F10 was significantly upregulated 3 d after 2 Gy or 10 Gy of irradiation (p < 0.0001 for both doses), but not as early as 1 d after RT.
Biodistribution and PET/CT imaging
Study 1: 2 Gy × 4 in MEER and B16F10 models
Biodistribution and PET/CT imaging of Zr-89-DFO-PD-L1 mAb was performed 3 d after the completion of fractionated RT (48 h post injection) in mice bearing either MEER tumors or B16F10 tumors (, ; ). In the MEER model, there were no differences in SUVmean, %ID/g, or the tumor-to-tissue ratios between the IR and non-IR tumors. However, in the B16F10 model, significantly higher uptake of Zr-89-DFO-PD-L1 mAb was observed in the neck tumors (IR) relative to flank tumors (non-IR) (, ; ). Furthermore, tumor-to-blood (T/B) and tumor-to-muscle (T/M) for the biodistribution were significantly higher for the IR B16F10 neck tumors relative to the non-IR flank tumors. These results demonstrate that PET imaging can discriminate RT-induced PD-L1 upregulation in B16F10 tumors but not in MEER tumors after 2 Gy × 4 fractionated RT ( and ).
Figure 1. Ex vivo biodistribution of radiotracer Zr-89-DFO-PD-L1 mAb (10F.9G2) uptake in mice with MEER tumors treated by 2 Gy × 4 fractionated RT (A) or in mice with B16F10 tumors treated by 2 Gy × 4 fractionated RT (B) at 48 h after injection (3 d after completion of RT). Results are expressed in %ID/g and represent mean ± SEM (n = 5). *p < 0.05, paired t-test.
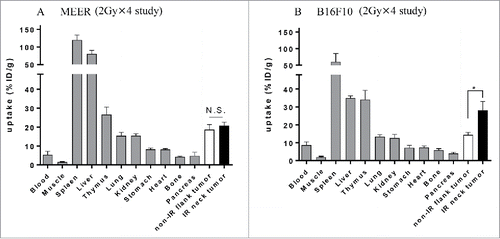
Figure 2. 2 Gy × 4 fractionated RT-induced PD-L1 upregulation in B16F10 was identified by PET/CT and its validity was corroborated by flow cytometry. (A) C57BL/6 mouse bearing MEER tumors or B16F10 tumors in two locations, neck and flank were treated with 2 Gy × 4 fractionated RT (only to the neck tumor) and anti PD-L1 PET/CT imaging and biodistribution study or flow cytometry were performed on day 20 after tumor implantation. (B) and (C) On day 1 after completion of RT, mice bearing MEER (B) or B16F10 (C) were injected Zr-89-DFO-PD-L1 mAb and PET/CT images were acquired at 48 h after injection. In-vivo or ex-vivo tracer uptake values (SUVmean and %ID/g, respectively) were compared between IR neck tumors and non-IR flank tumors (n = 5). Representative PET/CT images and tracer uptake values of IR and non-IR tumors were shown. (D) and (E) on day 3 after completion of RT, MEER tumors (D) or B16F10 tumors (E) were taken and flow cytometry analysis was performed. Representative flow cytometry histograms for PD-L1 expression and PD-L1 MFI of IR neck tumors and non-IR flank tumors of mice bearing MEER (n = 7, panel D) or B16F10 (n = 7), panel E). Sp, spleen; Li, liver. *p < 0.05, **p < 0.01; paired t-test.
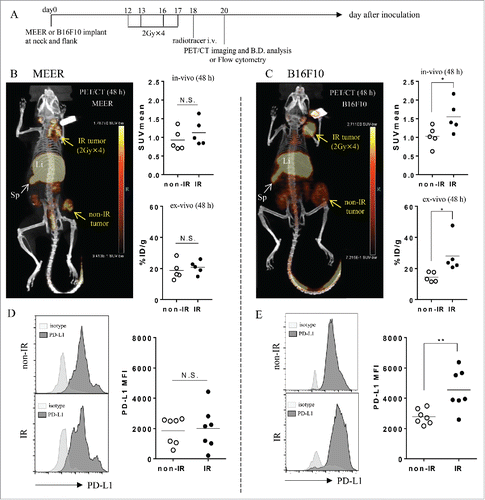
Table 1. Comparison of in vivo and ex vivo uptake values.
Study 2: 2Gy × 10 ± anti-PD-1 Ab in MEER model
Biodistribution and PET/CT imaging of Zr-89-DFO-PD-L1 mAb was performed 4 d after the completion of fractionated RT (96 h post injection) in mice bearing MEER tumors treated with RT in combination with anti-PD-1 Ab or its isotype control Ab, revealing high uptake of the tracer in PD-L1 positive organs of spleen and thymus (Table S1 and ). While the tumor uptake was not blocked with a large quantity of the unlabeled antibody, spleen uptake was reduced with a concomitant increase in blood, which apparently increased the overall uptake in the tumors. Anti-PD-1 mAb therapy did not influence tracer uptake, as there was no significant difference in uptake between non-IR (isotype) tumors and non-IR (anti-PD-1) tumors (; ). Therefore, the data from both groups of mice treated with anti-PD-1 and isotype Abs were combined. In this combined group, significantly higher uptake in IR neck tumors than that in non-IR flank tumors was observed (). Furthermore, T/B and T/M ratios for the biodistribution were higher for the IR MEER tumors relative to non-IR MEER tumors (; ). These results demonstrate that PET/CT imaging can discriminate RT-induced PD-L1 upregulation in MEER tumors after 2 Gy × 10-fractionated RT ± anti-PD-1 therapy.
Figure 3. Ex vivo biodistribution of Zr-89-DFO-PD-L1 mAb uptake at 96 h after injection (at 4 d after completion of 2 Gy × 10 fractionated RT ± anti-PD-1 Ab). Biodistribution of radiotracer uptake in anti-PD-1 Ab treated mice and isotype control Ab treated mice with MEER tumors (n = 6 per group). Each mouse has two tumors in two locations, neck and flank, and fractionated RT was delivered only to the neck tumor. Results are expressed in %ID/g and represent mean ± SEM (n = 6). **p < 0.01; paired t-test.
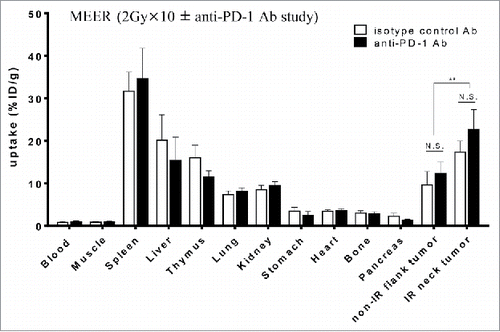
Figure 4. 2 Gy × 10 fractionated RT-induced PD-L1 upregulation in MEER was identified by PET/CT and its validity was corroborated by flow cytometry. (A) C57BL/6 mouse bearing MEER tumors in two locations, neck and flank were treated with fractionated RT (2 Gy × 10 only to the neck tumor) combined with anti-PD-1 Ab or isotype control Ab and PET/CT imaging and biodistribution study or flow cytometry were performed on day 33 after tumor implantation. (B) Tumor growth curves are presented as tumor volume measurements from day 10 to day 29 after tumor inoculation. Non-IR (isotype) tumors (n = 19), non-IR (anti-PD-1) tumors (n = 25), IR (isotype) tumors (n = 19) or IR (anti-PD-1) tumors (n = 25) were separately monitored. Data represent mean ± SEM (n = 5–25 per group). *p < 0.05, **p < 0.01; non-paired t-test. (C) RECIST outcome on day 29 after tumor inoculation (the last day of irradiation). (D) On the same day after completion of RT, mice bearing MEER treated with anti-PD-1 Ab or isotype control Ab were injected Zr-89-DFO-PD-L1 mAb and PET/CT images were acquired at 96 h after injection. In-vivo or ex-vivo tracer uptake values (SUVmean and %ID/g, respectively) were compared between non-IR (isotype) tumors and non-IR (anti-PD-1) tumors for analysis of anti-PD-1 Ab effect (n = 6), and thereafter compared between non-IR (isotype or anti-PD-1) tumors and IR (isotype or anti-PD-1) tumors for analysis of RT effect (n = 12). Representative PET/CT images and (E) SUVmean or %ID/g were shown. **p < 0.01; paired t-test. (F) On day 4 after completion of RT, MEER tumors were taken and flow cytometry analysis was performed. Representative flow cytometry histograms for PD-L1 expression and (G) the comparison of PD-L1 MFI between non-IR (isotype) tumors and non-IR (anti-PD-1) tumors for analysis of anti-PD-1 Ab effect (n = 5) and the comparison between non-IR (isotype or anti-PD-1) tumors and IR (isotype or anti-PD-1) tumors for analysis of RT effect (n = 10) were shown. Li, liver. *p < 0.05; paired t-test.
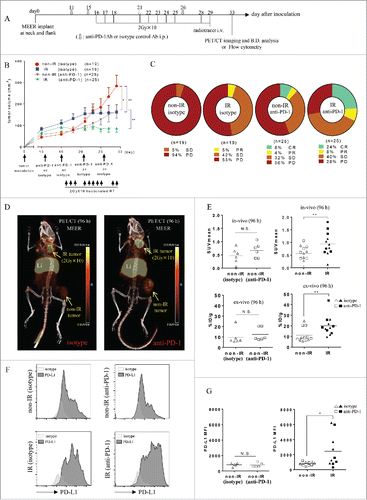
Treatment response
Study 1: 2 Gy × 4 in MEER and B16F10 models
On day 17 after tumor inoculation (the last day of RT), no significant therapy responses were observed in either MEER or B16F10 tumor model.
Study 2: 2 Gy × 10 ± anti-PD-1 Ab in MEER model
Tumor growth curves are shown in . On day 29 after tumor inoculation (the last day of RT), a significant beneficial effect of anti-PD-1 Ab was seen by comparing the tumor volumes of non-IR (isotype) and non-IR (anti-PD-1, p < 0.01). Tumor response/regression (using adapted RECIST measurements) outcomes on day 29 () indicate IR tumors were significantly smaller than non-IR tumors in each group of anti-PD-1 and isotype (p < 0.01 and p < 0.01, respectively).
RT of 2 Gy × 4 fractions upregulated PD-L1 expression in MEER or B16F10 tumors
To corroborate PD-L1 expression on tumor cells in vivo, MEER or B16F10 tumors at days 0, 1, 2 and 3 after completion of RT were disaggregated and flow cytometry was performed to quantify the extent of PD-L1 expression (Fig. S2). After fractionated RT (2 Gy × 4), significant PD-L1 upregulation on MEER tumors was seen at days 0 and 1 (p < 0.05, respectively). However, the expression of PD-L1 decreased to untreated level by day 3. In contrast, after the same schedule of fractionated RT, significant RT-induced PD-L1 upregulation in B16F10 tumors could be seen until day 3 after completion of RT (p < 0.05) (Fig. S2A). These data corroborated well with the PET/CT data, which show no significant difference in PD-L1 expression is observed between IR and non-IR MEER tumors 3 d after completion of RT.
PD-L1 expression on the different populations of cells in MEER or B16F10 tumors (CD45− or CD45+ tumor-infiltrating lymphocytes (TIL) populations, , , , ) on day 3 after completion of RT was measured by flow cytometry. Significant RT-induced PD-L1 upregulation was observed in population of both CD45− and CD45+ cells in B16F10 tumors (p < 0.05, respectively) (). Frequencies of CD45− and CD45+ cells among all live cells isolated from digested tumors on day 3 after RT in MEER or B16F10 tumors were measured by flow cytometry (, ). In B16F10 tumors, the frequencies of CD45+ cells were significantly increased (p < 0.05).
Figure 5. Flow cytometry analysis of CD45− cells or CD45+ cells in tumor tissues. Quantification of PD-L1 MFI and cell frequencies were measured. (A) Day 3 after completion of 2 Gy × 4 fractionated RT in MEER tumors. (B) Day 3 after completion of 2 Gy × 4 fractionated RT in B16F10 tumors. (C) Day 4 after completion of fractionated RT (2 Gy × 10) combined with anti-PD-1 Ab or its isotype control Ab in MEER tumors. Each mouse had two tumors in neck and flank, and only neck tumor was irradiated. *p < 0.05, **p < 0.01; paired t-test.
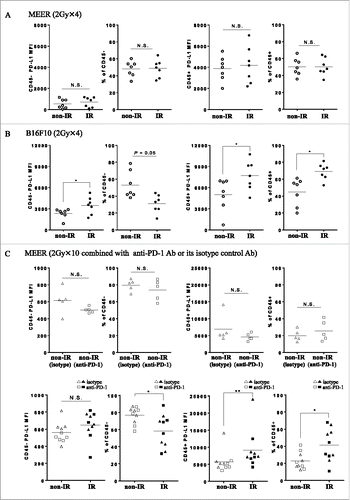
Anti-PD-1Ab did not upregulate PD-L1 expression in MEER tumors
The effect of anti-PD-1 Ab on PD-L1 upregulation was estimated by comparing non-IR (isotype) tumors and non-IR (anti-PD-1) tumors taken on day 4 after completion of RT. There were no significant PD-L1 median fluorescence intensity (MFI) differences between those tumors in either CD45+ or CD45− gated populations of all live cells ( and ). Frequencies of CD45− cells and CD45+ cells among all live cells isolated from digested tumor on day 4 after completion of RT in MEER tumors were also measured by flow cytometry. The frequencies of either CD45− cells or CD45+ cells were not significantly changed by anti-PD-1 therapy ().
RT of 2 Gy × 10 fractions ± anti-PD-1 Ab upregulated PD-L1 expression in MEER tumors, primarily on CD45+cells
To corroborate PD-L1 expression on tumors in vivo, we disaggregated MEER tumors (isotype group) on days 2 and 4 after completion of RT and performed flow cytometry to quantify the extent of PD-L1 expression (Fig. S2B). After fractionated RT (2 Gy × 10) without anti-PD-1 Ab, significant RT-induced PD-L1 upregulation by MEER tumors was observed on day 2 (p < 0.01) and remained elevated by day 4 (p < 0.05). In addition, the effect of RT on PD-L1 upregulation was also estimated by comparing neck tumors with flank tumors, by combining both groups of anti-PD-1 and isotype (day 4). After fractionated RT (2 Gy × 10) with or without anti-PD-1 Ab, significant RT-induced PD-L1 upregulation by MEER tumors was measured on day 4 (). PD-L1 expression on bulk CD45− or CD45+ TIL populations was also observed; however, RT-induced PD-L1 upregulation only occurred on CD45+ cells in MEER tumors (p < 0.01) ().
RT of 2 Gy × 10 fractions decreased frequencies of CD45− cells and increased frequencies of CD45+ cells in MEER tumors
Frequencies of CD45− cells and CD45+ cells among all live cells isolated from digested tumor on day 4 after completion of RT in MEER tumors were measured by combining both groups of anti-PD-1 and isotype Ab by flow cytometry (). The frequencies of CD45− cells were significantly decreased, whereas those of CD45+ cells were significantly increased (p < 0.05 and p < 0.05, respectively).
Intratumor heterogeneity of PD-L1 expression is present MEER and B16F10 models
The intratumor heterogeneity of PD-L1 IHC expression was observed in our experimentation subjects with an index of positive cells in total number of cells. Notable variation in PD-L1 expression was observed in both models, MEER and B16F10. This heterogeneity was also present in both IR neck and non-IR flank tumors (Fig. S3).
Vascular changes induced after fractionated RT
Morphologic parameters were measured to monitor the tumor hemodynamic physiology after RT. There were no differences observed in the number of blood vessels identified with CD31 between IR neck and non-IR flank tumors. However, marked dilation of the capillary microvasculature was evident in our B16F10 samples (p < 0.001) and a slight variation was observed in MEER (p = 0.07) (). No differences in perivascular space and active endothelial cells between neck and flank were observed in both B16F10 and MEER ( and ).
Figure 6. Quantitative immunohistochemical analysis of tumor vascular morphology. Morphologic parameters, including A, vascular size B, endothelial cell activation and C, perivascular space were measured to monitor the vascular changes that would influence tracer uptake. Marked vascular dilation after RT was seen in B16F10, however, no significant changes in the endothelial cell activation and the perivascular space were observed. ***p < 0.001; non-paired t-test.
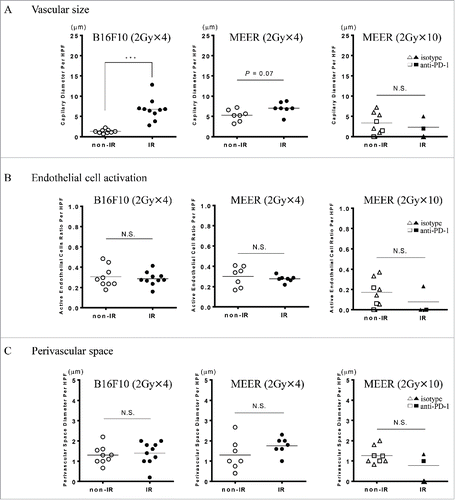
Discussion
While the immune system has the capacity to inhibit tumor growth, tumor cells can evade death through the expression of inhibitory proteins, such as PD-L1, which exert an immunosuppressive effect on PD-1+ TIL.Citation22 PD-L1, which can be upregulated during RT,Citation3,4,23 potentially suppresses antitumor immunity. However, combining RT with checkpoint inhibitor therapy (anti PD-L1 Ab) has been reported to enhance the efficacy of either treatment alone.Citation3-5 As such, PD-L1 upregulation during RT may serve a potential predictive biomarker for the efficacy of combining immune checkpoint inhibition with RT. Trials testing this combination are underway (NCT02759575) but are limited by static, baseline histopathologic assessment of the heterogeneous PD-L1 expression of small fragments of tissue. A better selection of possible clinical responders could be substantially improved by an imaging technique to permit monitoring of this important biomarker during therapy in the whole organ of interest.
It has been reported that clinical response and therapeutic benefit of anti-PD-1 therapy for recurrent HNSCC is observed in tumors scored as PD-L1 negative on baseline histologic specimens.Citation1 Having a non-invasive diagnostic molecular imaging strategy for PD-L1 expression might circumvent this issue for two reasons. First, sampling bias can be avoided by imaging the entire tumor mass. Second, dynamic changes to the tumor and its microenvironment induced by therapy can be readily monitored by molecular imaging. In this present study, we evaluated the IHC tumor heterogeneity of PD-L1 expression, and observed dramatic variation between different areas within the same tumor. Therefore, quantification of PD-L1 levels in patients non-invasively with PET/CT could elude these tissue-sampling biases and provide real-time information of PD-L1 levels to direct the commencement of therapy in patients. To better understand the dynamics and levels of PD-L1 induction during RT, we used preclinical immunoPET/CT, in conjunction with multi-channel flow cytometry, to determine if in vivo PET quantification correlated with ex vivo PD-L1 density on the tumor and tumor infiltrating myeloid cells.
The detailed mechanisms of RT-induced PD-L1 upregulation are not well defined. However, research has indicated that its expression is upregulated in a JAK/STAT1 dependent manner,Citation5 including responses to type I interferonCitation4,24 or type II interferonCitation25,26 produced after localized RT. RT-induced PD-L1 upregulation may vary depending on tumor histopathology, location (primary tumor or metastatic lesion), total dose of RT or even on the method of irradiation, such as stereotactic radiosurgery, hypo-fractionated regimens or conventional-dose fractionated RT. Deng et al. reported increased PD-L1 expression on TUBO tumor cells and on DCs 3 d after a single dose of 12 Gy. Dovedi et al. found that low doses of fractionated RT led to PD-L1 upregulation on tumor cells in a variety of syngeneic mouse models of cancer.Citation3 Contrary to these reports, Bernstein et al. observed that PD-L1 expressions in human prostate cancer cell lines were decreased 3 d after single dose of 10 Gy. In the current study, after fractionated RT of 2 Gy × 4 fractions, PD-L1 upregulation was observed on B16F10 tumors from day 0 to day 3 by flow cytometric analysis, which correlated well with the PET results on day 3 after completion of RT. In contrast, PD-L1 upregulation was not observed on MEER tumors on day 3 after completion of RT by either flow cytometric analysis or by PET imaging. Increasing RT to 2 Gy × 10 fractions in the MEER model resulted in PD-L1 upregulation on days 2 or 4 after completion of RT by flow cytometry and PET imaging. Further study is needed to determine which cancer types more readily upregulate PD-L1 expression due to RT and what doses or schedule of RT induce PD-L1 expression most avidly.
Interestingly, we found that RT-induced PD-L1 upregulation in B16F10 (2 Gy × 4) or MEER (2 Gy × 10 ± anti-PD-1 Ab) tumors was much higher in CD45+ cells than in CD45− cells (). In addition, a significant increase of CD45+ cell frequencies after completion of RT was seen in both tumor models (). Local RT results in massive intra-tumoral infiltration by CD45+CD11b+ myeloid derived cells in the B16F10 model, accompanied by upregulation of their activation products and production of chemotactic factors.Citation27,28 The RT-induced PD-L1 upregulation in tumors in the current study seemed to be mainly mediated by the increased number of myeloid cells with highly upregulated expression of PD-L1, indicating that these cells may be a novel therapeutic target within the tumor microenvironment.
To determine whether we could utilize immunoPET/CT to quantify the dynamic changes in PD-L1 expression in the animal models, we injected the radiotracer at 1 d after the completion of fractionated RT (2 Gy × 4) or on the same day of the completion of fractionated RT (2 Gy × 10) and performed PET scans 2 d or 4 d after the radiotracer injections, respectively. We compared RT-induced PD-L1 expression of an IR tumor (neck) with that of a non-IR tumor (flank) in the same mouse in conjunction with a separate cohort of mice that were killed for flow cytometry analysis of PD-L1 levels of the dissected tumors. For B16F10 (2 Gy × 4) on day 3 after completion of RT (48 h post injection) or MEER (2 Gy × 10 ± anti-PD-1 Ab) on day 4 after completion of RT (96 h post injection), tracer uptake values in the IR neck tumors were higher than those of non-IR tumors ex vivo (%ID/g, T/M and T/B) and correlated with SUVmean. These data correlate well with flow cytometric MFI of total PD-L1 expression in these models. Taken together, the data indicate that immunoPET/CT at 48 h or 96 h post injection can quantify changes in these models and would be suitable for confirming PD-L1 increases in IR tumors before the commencement of immune checkpoint immunotherapy or other interventions. In the MEER model, RT-induced PD-L1 upregulation was not observed in PET/CT imaging acquired on day 3 after completion of 2 Gy × 4, in agreement with lack of staining by flow cytometry on day 3, which had declined from relatively high induction of PD-L1 at day 0 to day 1 after completion of RT. These data suggest that the uptake values of immunoPET/CT with Zr-89-DFO-anti-mouse-PD-L1 mAb are weighted toward the latter part of the 48/96 h interval between injection of the tracer and imaging time.
Previous work with radiolabeled anti-PD-L1 mAbs (both anti-human and anti-mouse) has encompassed both PET and SPECT imaging in mice.Citation29-33 One feature that emerged from these studies is that there is a large antigen reservoir and that receptor mediated target tissue uptake may be highly dependent on specific activity. Hettich et al. showed that most cells of the spleen as well as number of other natural tissues express PD-L1 and found relatively high specific activities were required for PD-L1 mediated uptake in the xenografted tumor.Citation29,31 Other work showed little dependence on specific activity.Citation30 The specific activity of Zr-89-DFO-anti-mouse-PD-L1 mAb that we achieved (2 μCi/μg), while relatively low, was sufficient for good target tissue uptake in the mouse tumor models investigated. Additionally, the significant antigen sink can confound blocking studies to demonstrate target specificity in the xenografted tumor. We observed relatively high spleen uptake that was blocked with the administration of unlabeled antibody with concomitant increases in blood and tumor. This effect was also observed in blocking studies completed by Chatterjee et al.Citation30 Despite the encumbrance of significant antigen sink, we could clearly visualize differences in PD-L1 expression and achieve significant differences in SUV values that corresponded to the biodistribution data as well as to PD-L1 densities determined by flow cytometry. This is the first demonstration of PD-L1 imaging by immunoPET/CT with a clinically relevant RT protocol known to increase PD-L1 expression in the tumor microenvironment. These data indicate that immunoPET/CT with commercially available clone 10F.9G2 is suitable for quantification of PD-L1 densities in preclinical models.
It was previously demonstrated that external beam RT can induce alterations in tumor blood vessels that enhance permeability by RT-mediated damage to endothelial cells creating an altered hemodynamic state.Citation34,35 Enhanced permeability would increase tracer uptake in irradiated tumors above and beyond that mediated by PD-L1 upregulation. Moreover, increased capillary densities, because of tumor placement in the mouse, could increase tracer uptake such that tumor accumulation would be proportional to both the increased vascularization as well as increased PD-L1 accumulation. These effects depend on RT dose level, fraction size and time elapsed after exposure; as well as on biologic factors such as the tumor type, tumor site and the stage of tumor growth; and in dynamic variables as hypoxia. Therefore, to determine whether external beam RT could induce changes in the vasculature that would influence tracer uptake, we performed quantitative immunohistochemical analysis and we evaluated tumor capillary microcirculation morphology. We measured maximum dilation diameter, perivascular space size and endothelial cell activity to monitor the tumor hemodynamic physiology of irradiated neck and non-irradiated flank tumors. Marked dilation after RT in B16F10 capillary microvasculature and a slight increase was also observed in MEER. Despite that this effect can lead to diminished blood flow and enhanced permeability, the absence of changes in the perivascular space and cell activity variations in the endothelial cells suggest that the fluid interchange was maintained stable and edema was not developed. The development of tumoral edema after RT may be a consequence of imbalance between the interstitial and vascular hydrostatic pressures, and an altered permeability by dysfunctional endothelial cells. In our case, an increased intravascular hydrostatic pressure could be arrested by interstitial hypertension secondary to the high metabolic rate observed in tumors and the absence of a functional lymphatic system within the tumor. Therefore, we can conclude that the increased Zr-89-DFO-anti-mouse-PD-L1 mAb accumulation in B16F10 after RT of 2 Gy × 4 and MEER after RT of 2 Gy × 10 reflects increases in PD-L1 levels and does not reflect vascular changes. Other early changes induced also by radiotherapy including thrombosis, and perivascular hemorrhages were not observed and not considered in our analysis.
In conclusion, we demonstrate that fractionated, external beam RT upregulates PD-L1 in mouse models of HNSCC and melanoma, and that PD-L1 level can be non-invasively monitored by immunoPET/CT imaging using Zr-89 labeled anti-mouse PD-L1 mAb after therapeutic intervention in these models. Although the data presented here are with murine tumors and a Zr-89-labeled anti-mouse PD-L1 mAb, this is the first report showing that changes in PD-L1 expression post-RT can be quantified by PET imaging. Rapid translation of PET/CT imaging with a Zr-89-labeled anti-human PD-L1 mAb to RT-treated patients, possibly in combination with immune checkpoint inhibition therapy, is warranted.
Materials and methods
Cell lines
The murine tonsil epithelium E6/E7/H-ras transformed (MEER) cell lineCitation16 was kindly provided by Dr John Lee (Sanford Institute, Sioux Falls, SD). MEER cells were maintained in DMEM/F12 50/50 medium (Mediatech) supplemented with 10% FBS, 1% Pen/Strep (Life Technologies), 1% L-glutamine (Life Technologies, Carlsbad, CA), hydrocortisone (0.5 μg/mL, Sigma), cholera toxin (8.4 μg/μL, Sigma), transferrin (5 μg/mL, Sigma), insulin (5 μg/mL, Sigma), tri-iodo-thyronine (1.36 μg/μL, Sigma) and EGF (5 μg/μL, Sigma, St. Louis, MO). B16F10 cells were maintained in IMDM medium (Life Technologies), with 10% FBS (Mediatech, Manassas, VA), 1% Pen/Strep (Life Technologies), and 1% L-glutamine (Life Technologies), at 37°C in a humidified atmosphere with 5% CO2. All cell lines were routinely screened to confirm the absence of Mycoplasma contamination.
Tumor cell line irradiation (in vitro)
Resuspended MEER and B16F10 cells were irradiated in 1 mL of media in a 15 mL conical tube. The cells were treated with a single dose of 2 Gy or 10 Gy. A GammaCell 1000 irradiator was used to irradiate the suspension cultures at a constant dose rate of 10.6 Gy/min. PD-L1 expression was analyzed by flow cytometry on days 1 and 3 after irradiation.
Murine tumor models
Mouse studies were performed in compliance with approval from the Division of Laboratory Animal Resources at the University of Pittsburgh. Female, 6–8 weeks old, C57BL/6 mice (Jackson Laboratory) were anesthetized intraperitoneally with ketamine (90 mg/kg) and xylazine (7.5 mg/kg). Mice were inoculated with tumor cells (1 × 106 MEER or 2.5 × 105 B16F10 suspended in 100 μL PBS) subcutaneously in the nape of the neck and separately with tumor cells (0.5–1 × 106 MEER or 2.5 × 105 B16F10 suspended in 100 μL PBS) subcutaneously at the right flank on the same day.
Treatment with RT
Tumors established in the neck and flank were allowed to grow to 4–10 mm at the largest diameter for the neck and flank. Prior to RT, mice were immobilized in a modified 50 mL conical tube. The tip of the conical tube was removed and paper was used in the posterior portion to secure the mouse anteriorly toward the aerated tip before closure. Fractionated RT (2 Gy × 4 or 2 Gy × 10 fractions) was delivered only to the neck tumor using the Varian TrueBeam™ STx (Varian Medical Systems Palo Alto, CA), where the multi-leaf collimator was used to create a 2.0 cm × 40.0 cm field. Immobilized mice were secured on the treatment couch and positioned so that the tumor was within the center of the light field. A 1.0 cm tissue-equivalent bolus was placed over the tumor and a 100 cm SSD set-up was used to deliver 6 mV photons and a total of 8 or 20 Gy (2 Gy per fraction). Mice receiving 2 Gy × 10 fractionated RT also were treated with anti-mouse PD-1 Ab or its isotype control Ab. After the completion of either treatment, mice were randomized into two different cohort groups: (1) PET/CT imaging and biodistribution study; or (2) flow cytometry (Fig. S4A). Tumor diameter was measured every 2 to 3 d with an electronic caliper and tumor volume was calculated using the formula: (major axis; mm) × (minor axis; mm)Citation2 × 0.5. Mice were killed when tumors reached 1.5 cm at the largest diameter or if the tumors ulcerated.
Study 1: 2 Gy × 4 in MEER and B16F10 models
C57BL/6 mice were inoculated with 1 × 106 MEER or 2.5 × 105 B16F10 cells at both neck and flank and 23 mice bearing either MEER and 24 mice bearing B16F10 tumors in both sites (47 mice total) were enrolled in this study. Neck tumors were irradiated (2 Gy × 4 fractionated RT) starting on day 12. For all mice, fractionated RT was delivered on days 12, 13, 16 and 17 after tumor inoculation (Fig. S4B). After the completion of fractionated RT, mice of each tumor type (n = 5) were allocated to the PET/CT imaging/biodistribution study group and the remaining mice bearing MEER (n = 18) or B16F10 (n = 19) tumors were allocated to the flow cytometry group.
Study 2: 2 Gy × 10 ± anti-PD-1 Ab in MEER model
C57BL/6 mice were inoculated 1 × 106 MEER cells in neck and 5 × 105 MEER cells in flank. Neck tumors were irradiated (2 Gy × 10 fractionated RT, starting on day 16 after tumor inoculation). Mice received intraperitoneal injections of 60 μg anti-mouse-PD-1 mAb (clone 4H2) (anti-PD-1 group) or its isotype control mAb (clone MOPC-21) (isotype group) starting 5 d before irradiation and every 4–6 d thereafter for a total of four injections (Fig. S4C). Of the 44 mice bearing MEER tumors in the neck and flank, 25 mice were allocated to anti-PD-1 group and 19 mice were allocated to isotype group. Tumor growth of flank/neck tumors in isotype group (n = 19), and flank/neck tumors in anti-PD-1 group (anti-PD-1, n = 25) was monitored. After treatment, 8 of 25 mice in anti-PD-1 group and 3 out of 19 mice in isotype group were excluded from this study because of complete tumor regression/response in the neck (n = 6 and 1 in the anti-PD-1 or isotype groups, respectively), flank tumor (n = 1 in the anti-PD-1 group), both tumors (n = 1, in the anti-PD-1 group) or due to excessive tumor growth with bleeding (n = 2, isotype group). As a result, 17 mice in the anti-PD-1 group were enrolled in this study, and thereafter were randomly allocated into either the PET/CT imaging/biodistribution study group of 12 mice or the flow cytometry group (n = 5 mice). Similarly, mice in the isotype group (n = 16) were randomly allocated into the PET/CT imaging/biodistribution group (n = 6) and flow cytometry group (n = 10).
Preparation of Zr-89-DFO anti-PD-L1 mAb
p-NCS-Bz-DFO (Macrocyclics, Plano, TX), DMSO) was added to a mixture of anti-PD-L1 mAb (clone 10F.9G2, BioXCell (West Lebanon, NH) and phosphate buffer (0.1M, pH = 8.0) in a 5:1 molar ratio (p-NCS-Bz-DFO: mAb), and the reaction mixture was incubated at 4 °C with gentle rotation. The resulting DFO-anti-PD-L1 mAb conjugate was purified by Centricon 100 centrifuged filters, and the purity and concentration of the DFO- anti-PD-L1 mAb conjugate were determined by size exclusion chromatography (SEC).Citation17 The number of chelators per antibody was determined by titration with a known amount of non-radioactive zirconium oxalate.Citation18 DFO-anti-PD-L1 mAb was radiolabeled by mixing Zr-89-oxalic acid solution (1.0 mCi, Washington University (St. Louis, MO)) with the DFO-anti-PD-L1 mAb conjugate (0.5 mg, 1.0 M HEPES, pH 7.2, 37 °C, 1 h). Radiochemical purity was assessed by instant thin layer chromatograpy (iTLC) and radio-SEC. immunoreactivity was determined under antigen excess with 7 × 106 B16F10 cells/mL and ∼18,000 cpm Zr-89-DFO-anti-mouse-PD-L1 (n = 4).Citation19
Zr-89-DFO anti-PD-L1 mAb PET/CT and biodistribution of tumor bearing mice
Zr-89-DFO-anti-mouse-PD-L1 mAb (50 μg, 100 μCi) was administered intravenously (tail vein) one day after the completion of RT in 2 Gy × 4 model (n = 5, MEER and B16F10, respectively) or on the day of completion of RT in the 2 Gy × 10 ± anti-PD-1 Ab model (n = 12 and 6, anti-PD-1 group and isotype group, respectively). Of the 12 mice in the anti-PD-1 group, 6 mice were given 1 mg non-labeled anti-mouse-PD-L1 Ab (clone 10F.9G2) 2 h before tracer injection for a blocking study. PET/CT imaging was performed 48 h after tracer injection in mice receiving 2 Gy × 4, and 96 h for the groups receiving 2 Gy × 10 ± anti-PD-1 Ab. The mice were anesthetized using 2% isoflurane during PET/CT imaging. A small animal Inveon PET/CT scanner (Siemens Medical Solution, Knoxville, TN; tangential and radial full width at half maximum are 1.5 mm at the center of field of view (FOV) and 1.8 mm at the edge of FOV was used to collect static images (5 min). PET and CT images were co-registered using Inveon Research Workstation (IRW) software (Siemens Medical Solutions, Knoxville, TN). PET images were reconstructed with the ordered-subsets expectation maximization 3D/maximum a-posteriori probability (OSEM-3D/MAP) algorithm, and the analysis of images was done using IRW. Regions of interest were drawn based on the CT and the associated PET activities were calculated by the IRW software to generate mean standard uptake values (SUVmean; [nCi/mL] × [animal weight]/injected dose [nCi]). After PET/CT scanning, the mice were killed by cervical dislocation for biodistribution studies. Blood and organs (muscle, spleen, liver, thymus, lung, kidney, stomach, heart, bone, pancreas, non-irradiated (non-IR) flank tumor and irradiated (IR) neck tumor) were collected and weighed. The radioactivity for the blood and tissues was measured by gamma counting and converted to the percent-injected dose per gram of tissue (%ID/g).
Production of Zr-89-DFO-anti-mouse-PD-L1 mAb
Titration experiments with a known amount of zirconium oxalate showed ∼2 to 3 DFO chelators per antibody. Radiochemical yields were quantitative. Radio-SEC of Zr-89-DFO-anti-mouse-PD-L1 mAb demonstrated a monomeric species with no aggregates or degradants. The yield was determined by radio-iTLC and SEC (≥99%). The final specific activity was 2 μCi/μg and the immunoreactivity ranged from 55% to 75%.
Flow cytometric analysis
Tumor sample preparation
Mice were killed consecutively from day 0 (the same day immediately after the completion of fractionated RT) to day 3 after completion of RT in 2 Gy × 4 model (n = 3, 4, 4 and 7, or 4, 4, 4 and 7 on day 0, 1, 2 and 3 in MEER and B16F10 models, respectively) or day 2 and day 4 in 2 Gy × 10 ± anti-PD-1 Ab model (n = 5 in the isotype group on day 2; n = 5 in the isotype group and n = 5 in anti-PD-1 group on day 4). Both neck and flank tumors were harvested on each day. To prepare single cell suspensions, harvested tumors were minced into small pieces manually, transferred to 70-mm cell strainers (BD), and mechanically separated using the plunger of a 5-mL syringe.Citation20 Cells passing through the cell strainer were collected without subsequent density separation and washed by centrifugation (PBS × 2,400 rpm, 5 min). After washing, the cells were immediately stained for flow cytometry.
Antibodies and flow cytometry
The following anti-mouse antibodies (BioLegend (San Diego, CA) were used for staining: PD-L1-PE (Clone: 10F.9G2), CD45-Pacific blue (Clone: 30-F11), and IgG2b-PE isotype control (Clone: RTK4530). Cell viability was determined by Zombie Aqua staining for 15 min at 4 °C (BioLegend; Citation21), then incubated with fluorophore-conjugated antibodies at 1:100 dilution for 30 min at 4 °C, then washed twice, resuspended in 2% paraformaldehyde (PFA) solution for 30 min at room temperature and resuspended in PBS until analysis. Flow cytometric analysis was performed using a Fortessa cytometer (BD Biosciences (San Jose, CA)), and FlowJo version10 software. Briefly, cell surface PD-L1 expression on all disaggregated cells from each tumor (gated live cell populations of zombie aqua staining negative) and the PD-L1 expression on gated populations of CD45− (regarded as the tumor cell containing population) or CD45+ (regarded as lymphocytes or antigen presenting cells) were analyzed by measuring PD-L1 MFI. The PD-L1 MFI-fold change was calculated by normalizing the PD-L1 MFI of the IR neck tumor divided by the PD-L1 MFI of non-IR flank tumor. Frequencies of CD45− cells or CD45+ cells among all live cells isolated from digested tumor were also analyzed. In the vitro study using the cell lines, PD-L1 MFI-fold change was calculated by normalizing the PD-L1 MFI of non-IR or IR (2 Gy or 10 Gy) cell lines divided by the isotype control MFI.
Immunohistochemistry analysis
For immunohistochemical analyses of tumor the samples were fixed using neutral phosphate buffered 10% formalin (Fisher Scientific, Pittsburgh, PA) for at least 24 h. Samples were dehydrated using an EtOH gradient from 70% to 100% in a lapse of 24 h. Then samples were cleared with histology grade xylene (Fisher Scientific, Pittsburgh, PA) and embedded in granular paraffin. Five micron sections were prepared on polylysine slides. For hematoxylin and eosin staining, the sections were stained with Harris hematoxylin (Fisher Scientific, Pittsburgh, PA) and Shandon eosin (Fisher Scientific, Pittsburgh, PA). Immunostains were prepared after antigen retrieval through boiling with citrate buffer. Samples were blocked with 10% normal serum of the species in which the secondary antibody was made, and then the samples were incubated overnight at 4°C with the appropriate primary antibody. Primary antibodies used in this study were all rabbit polyclonal antibodies: PD-L1 (Proteintech Group Inc., Chicago, IL, dilution 1:200) and CD31 (Abcam, Cambridge, MA; dilution 1:150). Evnvision Dual Link + (EDL+) polymer detection (Dako, Carpenteria, CA) was used to detect the primary antibodies. The staining signal was amplified with avidin–biotin complex (Vector Laboratories) and developed using chromogen 3-amino-9-ethylcarbazole (Sigma-Aldrich, St. Louis, MO). A histomorphologic analysis of the tumors was performed with the HE slides. Tumor immunostaining was evaluated by a board certified dermatopathologist using a Karl Zeiss microscope. Pictures in TIFF format where collected mapping the whole tissue. Metric measurements where done in NIH ImageJ 1.50i (National Institutes of Health, Bethesda, MD) after standardize pixels to μm. Vascular size was assessed as the max blood vessel diameter observed, perivascular space as the maximum size from the blood vessel to the tumor parenchyma, and endothelial cell activation from the change from heterochromatin to euchromatin, including prominent nucleoli. Then the total cell count and the positive cells for DAB were measured by NIH ImageJ after generate 8 bit and inverted gray scale images.
Statistical analysis
All statistical analyses were performed using GraphPad Prism 6. Statistics were done with a paired or unpaired two-sided t-test and significance was assumed as p <0.05. Each column and error bar represents the mean and SEM. When estimating the effect of anti-PD-1 Ab or RT in the murine tumor model, the anti-PD-1Ab effect was first tested by comparing non-IR (isotype) tumors and non-IR (anti-PD-1) tumors before testing the RT effect, to justify testing the effect of RT by each group (anti-PD-1 or isotype) or by combining both groups. The RT effect was tested by each group if we found significant effects of anti-PD-1 Ab, whereas it was tested by combining both groups if there were no significant effects from the addition of anti-PD-1 Ab.
Disclosure of potential conflicts of interest
Robert L. Ferris: consulting or advisory role: AstraZeneca/Medimmune, Bristol Myers-Squibb, Merck. Research funding: AstraZeneca/Medimmune (Inst.) Bristol-Myers Squibb (Inst.) and VentiRx (Inst.).
KONI_A_1329071_s02.pptx
Download MS Power Point (756.5 KB)Acknowledgments
We thank William E. Gooding for the help with statistical analysis, and Kathryn Day and Joseph Latoche for their technical support.
Funding
This project used the UPCI Animal Facility, In Vivo Imaging Facility and Cytometry Facility that are supported in part by award P30CA047904. This work was supported by National Institute of Health grants R01 CA206517, DE019727, P50 CA097190, T32 CA060397 (RLF), R01 CA214018 (CJA), the University of Pittsburgh Cancer Institute award P30 CA047904 (RLF), R21 CA180211 (CJA), R21 EB023364 (WBE) and a University of Pittsburgh Physicians (UPP) Academic Foundation Award (WBE).
References
- Ferris RL, Blumenschein G Jr, Fayette J, Guigay J, Colevas AD, Licitra L, Harrington K, Kasper S, Vokes EE, Even C et al. Nivolumab for recurrent squamous-cell carcinoma of the head and neck. N Engl J Med 2016; 375:1856-67; PMID:27718784; https://doi.org/10.1056/NEJMoa1602252
- Wolchok JD, Kluger H, Callahan MK, Postow MA, Rizvi NA, Lesokhin AM, Segal NH, Ariyan CE, Gordon RA, Reed K et al. Nivolumab plus ipilimumab in advanced melanoma. N Engl J Med 2013; 369:122-33; PMID:23724867; https://doi.org/10.1056/NEJMoa1302369
- Dovedi SJ, Adlard AL, Lipowska-Bhalla G, McKenna C, Jones S, Cheadle EJ, Stratford IJ, Poon E, Morrow M, Stewart R et al. Acquired resistance to fractionated radiotherapy can be overcome by concurrent PD-L1 blockade. Cancer Res 2014; 74:5458-68; PMID:25274032; https://doi.org/10.1158/0008-5472.CAN-14-1258
- Deng L, Liang H, Burnette B, Beckett M, Darga T, Weichselbaum RR, Fu YX. Irradiation and anti-PD-L1 treatment synergistically promote antitumor immunity in mice. J Clin Invest 2014; 124:687-95; PMID:24382348; https://doi.org/10.1172/JCI67313
- Azad A, Yin Lim S, D'Costa Z, Jones K, Diana A, Sansom OJ, Kruger P, Liu S, McKenna WG, Dushek O, et al. PD-L1 blockade enhances response of pancreatic ductal adenocarcinoma to radiotherapy. EMBO Mol Med 2017; 9, 167–80; PMID:27932443; https://doi.org/10.15252/emmm.201606674.
- Ilie M, Long-Mira E, Bence C, Butori C, Lassalle S, Bouhlel L, Fazzalari L, Zahaf K, Lalvée S, Washetine K et al. Comparative study of the PD-L1 status between surgically resected specimens and matched biopsies of NSCLC patients reveal major discordances: A potential issue for anti-PD-L1 therapeutic strategies. Ann Oncol 2016; 27:147-53; PMID:26483045; https://doi.org/10.1093/annonc/mdv489
- McLaughlin J, Han G, Schalper KA, Carvajal-Hausdorf D, Pelekanou V, Rehman J, Velcheti V, Herbst R, LoRusso P, Rimm DL. Quantitative assessment of the heterogeneity of PD-L1 expression in non-small-cell lung cancer. JAMA Oncol 2016; 2:46-54; PMID:26562159; https://doi.org/10.1001/jamaoncol.2015.3638
- Mansfield AS, Aubry MC, Moser JC, Harrington SM, Dronca RS, Park SS, Dong H. Temporal and spatial discordance of programmed cell death-ligand 1 expression and lymphocyte tumor infiltration between paired primary lesions and brain metastases in lung cancer. Ann Oncol 2016; 27:1953-8; PMID:27502709; https://doi.org/10.1093/annonc/mdw289
- Sheng J, Fang W, Yu J, Chen N, Zhan J, Ma Y, Yang Y, Huang Y, Zhao H, Zhang L. Expression of programmed death ligand-1 on tumor cells varies pre and post chemotherapy in non-small cell lung cancer. Sci Rep 2016; 6:20090; PMID:26822379; https://doi.org/10.1038/srep20090 10.1038/srep23850
- Lim SH, Hong M, Ahn S, Choi YL, Kim KM, Oh D, Ahn YC, Jung SH, Ahn MJ, Park K et al. Changes in tumour expression of programmed death-ligand 1 after neoadjuvant concurrent chemoradiotherapy in patients with squamous oesophageal cancer. Eur J Cancer 2016; 52:1-9; PMID:26623522; https://doi.org/10.1016/j.ejca.2015.09.019
- Hettich M, Braun F, Bartholoma MD, Schirmbeck R, Niedermann G. High-resolution PET imaging with therapeutic antibody-based PD-1/PD-L1 checkpoint tracers. Theranostics 2016; 6:1629-40; PMID:27446497; https://doi.org/10.7150/thno.15253
- Lesniak WG, Chatterjee S, Gabrielson M, Lisok A, Wharram B, Pomper MG, Nimmagadda S. PD-L1 detection in tumors using [(64)Cu]Atezolizumab with PET. Bioconjug Chem 2016; 27:2103-10; PMID:27458027; https://doi.org/10.1021/acs.bioconjchem.6b00348
- Maute RL, Gordon SR, Mayer AT, McCracken MN, Natarajan A, Ring NG, Kimura R, Tsai JM, Manglik A, Kruse AC et al. Engineering high-affinity PD-1 variants for optimized immunotherapy and immuno-PET imaging. Proc Natl Acad Sci USA 2015; 112:E6506-14; PMID:26604307; https://doi.org/10.1073/pnas.1519623112
- Chatterjee S, Lesniak WG, Miller MS, Lisok A, Sikorska E, Wharram B, Kumar D, Gabrielson M, Pomper MG, Gabelli SB et al. Rapid PD-L1 detection in tumors with PET using a highly specific peptide. Biochem Biophys Res Commun 2017; 483:258-63; PMID:28025143; https://doi.org/10.1016/j.bbrc.2016.12.156
- McCabe KE, Wu AM. Positive progress in immunoPET—not just a coincidence. Cancer Biother Radiopharm 2010; 25:253-61; PMID:20578830; https://doi.org/10.1089/cbr.2010.0776
- Hoover AC, Spanos WC, Harris GF, Anderson ME, Klingelhutz AJ, Lee JH. The role of human papillomavirus 16 E6 in anchorage-independent and invasive growth of mouse tonsil epithelium. Arch Otolaryngol Head Neck 2007; 133:495-502; PMID:17515506; https://doi.org/10.1001/archotol.133.5.495
- Zeng D, Guo Y, White AG, Cai Z, Modi J, Ferdani R, Anderson CJ. Comparison of conjugation strategies of cross-bridged macrocyclic chelators with cetuximab for copper-64 radiolabeling and PET imaging of EGFR in colorectal tumor-bearing mice. Mol Pharm 2014; 11:3980-7; PMID:24720806; https://doi.org/10.1021/mp500004m
- Meares CF, McCall MJ, Reardan DT, Goodwin DA, Diamanti CI, McTigue M. Conjugation of antibodies with bifunctional chelating agents: Isothiocyanate and bromoacetamide reagents, methods of analysis, and subsequent addition of metal ions. Anal Biochem 1984; 142:68-78; PMID:6440451; https://doi.org/10.1016/0003-2697(84)90517-7
- Lindmo T, Bunn PA Jr. Determination of the true immunoreactive fraction of monoclonal antibodies after radiolabeling. Methods Enzymol 1986; 121:678-91; PMID:3523136
- Li J, Jie HB, Lei Y, Gildener-Leapman N, Trivedi S, Green T, Kane LP, Ferris RL. PD-1/SHP-2 inhibits Tc1/Th1 phenotypic responses and the activation of T cells in the tumor microenvironment. Cancer Res 2015; 75:508-18; PMID:25480946; https://doi.org/10.1158/0008-5472.CAN-14-1215
- Schmitt NC, Trivedi S, Ferris RL. STAT1 activation is enhanced by cisplatin and variably affected by EGFR inhibition in HNSCC cells. Mol Cancer Ther 2015; 14:2103-11; PMID:26141950; https://doi.org/10.1158/1535-7163.MCT-15-0305
- Dong H, Strome SE, Salomao DR, Tamura H, Hirano F, Flies DB, Roche PC, Lu J, Zhu G, Tamada K et al. Tumor-associated B7-H1 promotes T-cell apoptosis: A potential mechanism of immune evasion. Nat Med 2002; 8:793-800; PMID:12091876; https://doi.org/10.1038/nm0902-1039c
- Vanpouille-Box C, Diamond JM, Pilones KA, Zavadil J, Babb JS, Formenti SC, Barcellos-Hoff MH, Demaria S. TGFbeta is a master regulator of radiation therapy-induced antitumor immunity. Cancer Res 2015; 75:2232-42; PMID:25858148; https://doi.org/10.1158/0008-5472.CAN-14-3511
- Schreiner B, Mitsdoerffer M, Kieseier BC, Chen L, Hartung HP, Weller M, Wiendl H. Interferon-beta enhances monocyte and dendritic cell expression of B7-H1 (PD-L1), a strong inhibitor of autologous T-cell activation: Relevance for the immune modulatory effect in multiple sclerosis. J Neuroimmunol 2004; 155:172-82; PMID:15342209; https://doi.org/10.1016/j.jneuroim.2004.06.013
- Bellucci R, Martin A, Bommarito D, Wang K, Hansen SH, Freeman GJ, Ritz J. Interferon-gamma-induced activation of JAK1 and JAK2 suppresses tumor cell susceptibility to NK cells through upregulation of PD-L1 expression. Oncoimmunology 2015; 4:e1008824; PMID:26155422; https://doi.org/10.1080/2162402X.2015.1008824
- Lugade AA, Sorensen EW, Gerber SA, Moran JP, Frelinger JG, Lord EM. Radiation-induced IFN-gamma production within the tumor microenvironment influences antitumor immunity. J Immunol 2008; 180:3132-9; PMID:18292536; https://doi.org/10.4049/jimmunol.180.5.3132
- Shahabi V, Postow MA, Tuck D, Wolchok JD. Immune-priming of the tumor microenvironment by radiotherapy: Rationale for combination with immunotherapy to improve anticancer efficacy. Am J Clinical Oncol 2015; 38:90-7; PMID:25616204; https://doi.org/10.1097/COC.0b013e3182868ec8
- Burnette BC, Liang H, Lee Y, Chlewicki L, Khodarev NN, Weichselbaum RR, Fu YX, Auh SL. The efficacy of radiotherapy relies upon induction of type i interferon-dependent innate and adaptive immunity. Cancer Res 2011; 71:2488-96; PMID:21300764; https://doi.org/10.1158/0008-5472.CAN-10-2820
- Heskamp S, Hobo W, Molkenboer-Kuenen JDM, Olive D, Oyen WJG, Dolstra H, Boerman OC. Noninvasive imaging of tumor PD-L1 expression using radiolabeled anti-PD-L1 antibodies. Cancer Res 2015; 75:2928-36; PMID:25977331; https://doi.org/10.1158/0008-5472.CAN-14-3477
- Chatterjee S, Lesniak WG, Gabrielson M, Lisok A, Wharram B, Sysa-Shah P, Azad BB, Pomper MG, Nimmagadda S. A humanized antibody for imaging immune checkpoint ligand PD-L1 expression in tumors. Oncotarget 2016; 7:10215-27; PMID:26848870; https://doi.org/10.18632/oncotarget.7143
- Hettich M, Braun F, Bartholomä MD, Schirmbeck R, Niedermann G. High-resolution PET imaging with therapeutic antibody-based PD-1/PD-L1 checkpoint tracers. Theranostics 2016; 6:1629; PMID:27446497; https://doi.org/10.7150/thno.15253
- Josefsson A, Nedrow JR, Park S, Banerjee SR, Rittenbach A, Jammes F, Tsui B, Sgouros G. Imaging, biodistribution, and dosimetry of radionuclide-labeled PD-L1 antibody in an immunocompetent mouse model of breast cancer. Cancer Res 2016; 76:472-9; PMID:26554829; https://doi.org/10.1158/0008-5472.CAN-15-2141
- Lesniak WG, Chatterjee S, Gabrielson M, Lisok A, Wharram B, Pomper MG, Nimmagadda S. PD-L1 detection in tumors using [64Cu] atezolizumab with PET. Bioconjug Chem 2016; 27:2103-10; PMID:27458027; https://doi.org/10.1021/acs.bioconjchem.6b00348
- Potchen EJ, Kinzie J, Curtis C, Siegel BA, Studer RK. Effect of irradiation on tumor microvascular permeability to macromolecules. Cancer 1972; 30:639-43; PMID:4677852; https://doi.org/10.1002/1097-0142(197209)30:3%3c639::AID-CNCR2820300308%3e3.0.CO;2-3
- Kobayashi H, Reijnders K, English S, Yordanov AT, Milenic DE, Sowers AL, Citrin D, Krishna MC, Waldmann TA, Mitchell JB et al. Application of a macromolecular contrast agent for detection of alterations of tumor vessel permeability induced by radiation. Clin Cancer Res 2004; 10:7712-20; PMID:15570005; https://doi.org/10.1158/1078-0432.CCR-04-1175