Abstract
An NADH-dependent reductase (ClCR) was discovered by genome data mining. After ClCR was overexpressed in E. coli BL21, recombinant E. coli CCZU-T15 with high reductase activity and excellent stereoselectivity for the reduction of ethyl 4-chloro-3-oxobutanoate (COBE) into ethyl (S)-4-chloro-3-hydroxybutanoate [(S)-CHBE] was screened using a modified high-throughput colorimetric screening strategy. After the reaction optimization, a highly stereoselective bioreduction of COBE into (S)-CHBE (>99% ee) with the resting cells of E. coli CCZU-T15 was successfully demonstrated in toluene-water (50:50, v/v) biphasic system. Biotransformation of 1000 mM COBE for 24 h in the biphasic system, (S)-CHBE (>99% ee) could be obtained in the high yield of 96.4%. Significantly, E. coli CCZU-T15 shows high potential in the industrial production of (S)-CHBE (>99% ee).
Introduction
It is well-known that optically active alcohols containing at least an asymmetric center are important building blocks for the production of agricultural and pharmaceutical chemicals.Citation1,2 Ethyl (S)-4-chloro-3-hydroxybutanoate [(S)-CHBE], one of the optically active alcohols, is a key chiral synthon for the side-chain of cholesterol-lowering drug atorvastatin (Lipitor),Citation3,4 which is the inhibitor of hydroxymethylglutaryl CoA (HMG-CoA) reductase. It also can be employed in the synthesis of Slagenins B, Slagenins C, and 1,4-dihydropyridine type β-blockers.Citation5,6 Recently, the biocatalytic synthesis of (S)-CHBE is of significant interest due to mild reaction condition and high stereroselectivity compared with conventional chemical synthesis.Citation7,8 Notably, enzymatic asymmetric bioreduction of inexpensive ethyl 4-chloro-3-oxobutanoate (COBE), which can be easily synthesized from diketene, is a promising and alternative way to synthesize (S)-CHBE.Citation9,10
However, asymmetric biotransformation of COBE to (S)-CHBE become quite challenging due to the requirement of expensive cofactors (e.g., NADH or NADPH).Citation11 The coupled substrate approach, coupled enzyme approach, in vivo (i.e., relying on the natural cofactor regeneration system of living organisms) approach, electro-enzymatic approach, electro-chemical approach, and photo-chemical approach have been used for the regeneration cofactors.Citation12 To make industrial-scale biotransformations to be of economic viability, in situ cofactor regeneration is an effective and practical way.Citation12,13 It has been reported that enzyme-coupled and substrate-coupled approaches could been used for the cost-effective recycling of cofactor.Citation13,14 Formate dehydrogenase (FDH) or glucose dehydrogenase (GDH) has been used as enzyme-coupled systems for recycling NAD+ or NADP+. 2-Propanol can be chosen as an ideal co-substrate, because of its relatively low cost and the feasibility of forcing the reaction toward completion by removing the co-product (acetone) under reduced pressure. Many reductases (e.g., LsADH from Leifsonia sp., PsCR from Pichia stipitis, S1 from Candida magnoliae, etc.) have already been cloned for the asymmetric biosynthesis of chiral alcohols.Citation13-16 However, the substrate loading are still not high, and the enantioselectivity was unsatisfactory, thereby hindering their practical application. Therefore, there is still an urgent need for novel and highly efficient biocatalyst for the cost-effective production of chiral alcohols.
In the post-genomic era, the booming growth of genome sequences in the database offers an unprecedented opportunity for accessing numerous biocatalysts with high potential for practical application.Citation17 Compared with time-consuming and laborious traditional screening from soil samples, genome mining is a more time-effective and promising strategy for the fast identification of target enzymes with desired properties.Citation18 To obtain more COBE-reducing enzymes for the synthesis of (S)-CHBE, it is necessary to build a convenient, rapid and accuracy screening strategy for screening the desired COBE-reducing biocatalysts. Many high-throughput methods have been employed to screen biocatalysts.Citation19,20 The use of the conventional chromatographic method is usually timeconsuming and inefficient. In the previous report, one spectrophotometric determination of COBE in aqueous solutions was developed based on the formation of purple ferric compound from the enol structure of COBE in the presence of acidic ferric perchlorate (Fe(ClO4)3) solution.Citation20 However, the Fe(ClO4)3 is not cheap.
In this study, a modified high-throughput screening strategy was employed to screen COBE-reducing biocatalysts. Moreover, an NADH-dependent reductase (ClCR) from Clavispora lusitaniae was discovered by genome data mining. After ClCR was overexpressed in E. coli BL21, a high activity of recombinant E. coli CCZU-T15 was used for the efficient biotransformation of COBE into (S)-CHBE (>99% ee). To improve the biocatalytic efficiency, a toluene-water (50:50, v/v) biphasic system could be employed for the synthesis of (S)-CHBE. Finally, a highly stereoselective bioreduction of COBE into (S)-CHBE by E. coli CCZU-T15 was successfully demonstrated in the toluene-water (50:50, v/v) biphasic system.
One Modified High-Throughput Screening Strategy
COBE, one of β-carbonyl carboxylic ester, has 2 tautomers (e.g., enol and ketone).Citation20 Notably, COBE with enol structure can form purple ferric compound in the presence of acidic Fe3+ solution (). In this study, a modified colorimetric reaction was developed when ferric perchlorate (Fe(ClO4)3) was substituted by the cheap ferric chlorate (FeCl3) at room temperature (20–40°C). It was found that the highest characteristic peak was observed at 500 nm in the absorption spectrum (). When the product CHBE was replaced by the substrate COBE, there was not a clear peak at this wavelength, and no significant color change was also found, indicating the background of the product CHBE could be eliminated during the assay. The purple color compound formed immediately when 120 mM COBE was added into the acidic FeCl3 solution (0.080 M FeCl3, 0.70 M HClO4). Furthermore, the effects of FeCl3 concentration (0.010–0.10 M), incubation temperature (20–40°C), and incubation time (2–30 min) were examined, and the optimum color-generating conditions were obtained: FeCl3 0.080 M, incubation temperature 30°C, incubation time 15 min.
Figure 1. Proposed color-generating mechanism (a). Effects of asorbance wavelength (b), FeCl3 concentration (c), incubation temperature (d), and incubation time (e) on color-developing.
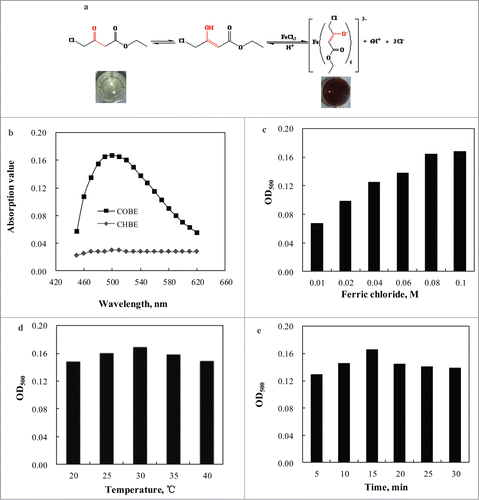
Therefore, the spectrophotometric determination of COBE in aqueous solutions was performed in a 24-well plate as follows: 390 μL acidic f FeCl3 solution (0.080 M FeCl3, 0.70 M HClO4) and 10 μL COBE samples were mixed for 15 min at 30°C, and then 3600 μL ethanol was added into the mixture. This spectrophotometric assay was conducted at 500 nm. The calibration curve was plotted and OD500 was linear with the concentrations of COBE on the 24-well plate (CCOBE(mM) = 714.3 × OD500; R2 = 0.9915). Compared with the previous report,Citation20 the inexpensive FeCl3 was used, and incubation time was shortened.
Screening for The Ideal Reductase-Producer
To effectively biotransform COBE into corresponding optically active (S)-CHBE, a genome data mining was employed to find desired reductases. Cloning and expression of ClCR gene in Escherichia coli was performed as followings: genomic DNA was extracted from Clavispora lusitaniae CGMCC 2.1597, using a TIANamp Bacteria DNA Kit from Tiangen (Shanghai). Oligonucleotide primers (ClCR-F: 5′-CATGCTAGCATGGTTCAATCTCATATCAACG-3′ and ClCR-R: 5′-CCGCTCGAGCTATGGTGCACAGTAACCAC-3′) with Nhe I and XhoI restriction sites were designed according to the ClCR gene sequence. The resulting plasmid, pET-28a-ClCR, was transformed into E. coli BL21 (DE3) cells. Furthermore, the established high-throughput screening strategy on the 24-well plate was used for screening the high COBE-reducing activity of recombinant E. coli. This strain named recombinant E. coli CCZU-T15 was preserved in our laboratory. The expression of ClCR was about 30.2 kDa on SDS–PAGE (, lane 2).
Establishment and Optimization of Aqueous-Solvent Biphasic Media
Since COBE and its product are unstable in aqueous system and toxic to reductases.Citation17 A biphasic biotransformation system can be effectively employed for the asymmetric bioreduction of COBE to (S)-CHBE. Notably, the selection of the biocompatible organic cosolvent as the carrier for substrate COBE and product CHBE is based on its biocompatibility toward the biocatalyst and adequate partition of both COBE and CHBE to provide a pool for these chemicals.Citation17,20 Organic cosolvents with different log P values for COBE and CHBE and stabilities of ClCR in various organic solvent-water biphasic systems were investigated. As shown in , lower reductase activities of ClCR were observed when using dibutylphthalate (log P = 5.4). The reductase activity of COBE increased when ethyl acetate (log P = 0.68), toluene (log P = 2.5), n-hexane (log P = 3.5) and n-heptane (log P = 4.0) were used. Significantly, the highest activity was found in the presence of toluene, and no effect on the optical purity of the product (S)-CHBE was found. Consequently, toluene (log P = 2.5) was selected as a suitable organic phase of the biphasic system for the asymmetric bioreduction of COBE to (S)-CHBE.
Figure 3. Effects of the organic solvent (a) and potential cosubstrate (b) on the reductase activity.
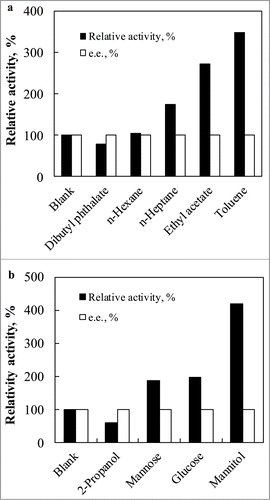
ClCR prefers the inexpensive cofactor NAD(H) instead of NADP(H) as the electron donor (Data not shown), and the system employed did not require the use of cells expressing additional enzyme such as glucose dehydrogenase. To choose the appropriate cosubstrate, 4 potential cosubstrates (glucose, 2-propanol, mannitol and mannose) were texted on the reductase activity, respectively. As shown in , 3 cosubstrates (glucose, mannitol and mannose) could improve the reductase activity significantly. Clearly, the recombinant E. coli CCZU-T15 had substrate-coupled system. Using mannitol as cosubstrate, the highest reductase activity was obtained, and no significant effect on the optical purity of the product (S)-CHBE was found. Thus, a substrate-coupled biocatalytic process was demonstrated using mannitol as a cosubstrate.
Based on the above results, the appropriate reaction system was the toluene-water (50:50, v/v) biphasic media. In this biphasic system, the optimum reaction conditions were obtained: NAD+ 0.1 μmol/(mmol COBE), cosubstrate mannitol 1 mmol/(mmol COBE), reaction temperature 30°C, reaction pH 6.5, and cell dosage 0.060 g (wet weight)/mL, respectively.
Asymmetric Reduction of COBE in the Toluene-Water Biphasic System
To effectively synthesize (S)-CHBE (>99% ee), asymmetric biotransformation of COBE (500–1000 mM) by recombinant E. coli CCZU-T15 were carried out in the toluene-water (50:50, v/v) biphasic system. Furthermore, time courses for the reduction of COBE into (S)-CHBE with the resting cells were investigated in the biphasic media (). 500 mM COBE could be completely biotransformed after 4 h. Biotransformation of 1000 mM COBE for 24 h, (S)-CHBE (>99% ee) was obtained in the high yield of 96.4%. Prolonged the reaction time for another 24 h, 1000 mM COBE could be completely transformed into (S)-CHBE. However, COBE at 50–200 mM could be reduced into (S)-CHBE with the yields of 54.1–79.4% after 24 h in the monophasic aqueous media. Using whole cells of recombinant E. coli CCZU-Y10 for biotransforming 1,000 mM COBE for 24 h, the yield of (S)-CHBE was obtained at nearly 90.0% in the dibutyl phthalate-water biphasic system.Citation18 Clearly, a highly stereoselective bioreduction of COBE into (S)-CHBE by E. coli CCZU-T15 was successfully demonstrated in the toluene-water (50:50, v/v) biphasic system.
Conclusions
Chiral molecules have become an important part of the pharmaceutical and agricultural industries.Citation1,20,21 Based on a modified high-throughput screening strategy for β-carbonyl carboxylic ester reductase, an NADH-dependent reductase (ClCR) from recombinant E. coli CCZU-T15 with high biocatalytic activity and excellent stereoselectivity was used for synthesising (S)-CHBE (>99% ee) from COBE. After the reaction optimization, the optimum reaction conditions were obtained. The good performance was obtained in the toluene-water (50:50, v/v) biphasic system. Furthermore, high concentration of COBE (1000 mM) could be asymmetrically reduced to (S)-CHBE with high yield (96.4%) and excellent ee value (>99%) after 24 h. In conclusion, recombinant E. coli CCZU-T15 has high potential in the synthesis of (S)-CHBE (>99% ee).
Disclosure of Potential Conflicts of Interest
No potential conflicts of interest were disclosed.
Funding
This work was financially supported by the National Natural Science Foundation of China (No. 21102011) and the Natural Science Foundation of Jiangsu Province (No. BK20141172).
References
- Breuer M, Ditrich K, Habicher T, Hauer B, Kesseler M, Sturmer R, Zelinski T. Industrial methods for the production of optically active intermediates. Angew Chem Int Ed Engl 2004; 43:788-824; PMID: 14767950; http://dx.doi.org/10.1002/anie.200300599
- Cao H, Mi L, Ye Q, Zhang G L, Yan M, Wang Y, Zhang Y Y, Li X M, Xu L, Xiong J, et al. Purification and characterization of a novel NADH-dependent carbonyl reductase from Pichia stipitis involved in biosynthesis of optically pure ethyl (S)-4-chloro-3-hydroxybutanoate. Bioresour Technol 2011; 102:1733-9; PMID: 20933386; http://dx.doi.org/10.1016/j.biortech.2010.08.072
- He Y C, Tao Z C, Zhang X, Yang Z X, Xu J H. Highly efficient synthesis of ethyl (S)-4-chloro-3-hydroxybutanoate and its derivatives by a robust NADH-dependent reductase from E. coli CCZU-K14. Bioresour Technol 2014; 461-4; PMID: 24745897; http://dx.doi.org/10.1016/j.biortech.2014.03.133
- Ošlaj M, Cluzeau J, Orkić D, Kopitar G, Mrak P, Časar Z. A highly productive, whole-cell DERA chemoenzymatic process for production of key lactonized side-chain intermediates in statin synthesis. 2013; PLoS One. 2013; 8(5): e62250; PMID: 23667462; http://dx.doi.org/10.1371/journal.pone.0062250
- Choi H J, Uhm K N, Kim H K. Production of chiral compound using recombinant Escherichia coli cells co-expressing reductase and glucose dehydrogenase in an ionic liquid/water two phase system. J Mol Catal B: Enzym 2011; 70:114-8; http://dx.doi.org/10.1016/j.molcatb.2011.02.013
- Ema T, Ide S, Okita N, Sakai T. Highly efficient chemoenzymatic synthesis of methyl (R)-o-chloromandelate, a key intermediate for clopidogrel, via asymmetric reduction with recombinant Escherichia coli. Adv Synth Catal 2008; 350:2039-44; http://dx.doi.org/10.3390/biom4010117;
- Jung J, Park H J, Uhm K N, Kim D, Kim H K. Asymmetric synthesis of (S)-ethyl-4-chloro-3-hydroxy butanoate using a Saccharomyces cerevisiae reductase: enantioselectivity and enzyme-substrate docking studies. Biochim Biophys Acta 2010; 1804:1841-9; PMID: 20601218; http://dx.doi.org/10.1016/j.bbapap.2010.06.011
- Ye Q, Cao H, Mi L, Yan M, Wang Y, He Q T, Li J, Xu L, Chen Y J, Xiong J, et al. Biosynthesis of (S)-4-chloro-3-hydroxybutanoate ethyl using Escherichia coli co-expressing a novel NADH-dependent carbonyl reductase and a glucose dehydrogenase. Bioresour Technol 2010; 101:8911-4; PMID: 20630744; http://dx.doi.org/10.1016/j.biortech.2010.06.098
- Inoue K, Makino K, Itoh N. Purification and characterization of a novel alcohol dehydrogenase from Leifsonia sp. strain S749: a promising biocatalyst for an asymmetric hydrogen transfer bioreduction. Appl Environ Microbiol 2005; 71:3633-41; PMID: 16000771; http://dx.doi.org/10.1128/AEM.71.7
- Gröger H, Chamouleau F, Orologas N, Rollmann C, Drauz K, Hummel W, Weckbecker A, May O. Enantioselective reduction of ketones with "designer cells" at high substrate concentrations: highly efficient access to functionalized optically active alcohols. Angew Chem Int Ed 2006; 45:5677-81; PMID: 16858704; http://dx.doi.org/10.1002/anie.200503394
- Goldberg K, Schroer K, Lütz S, Liese A. Biocatalytic ketone reduction - a powerful tool for the production of chiral alcohols-part I: processes with isolated enzymes. Appl Microbiol Biotechnol 2007; 76:237-48; PMID: 17486338; http://dx.doi.org/10.1007/s00253-007-1002-0
- De Wildeman S M, Sonke T, Schoemaker H E, May O. Biocatalytic reductions: from lab curiosity to "first choice". Acc Chem Res 2007; 40:1260-6; PMID: 17941701; http://dx.doi.org/10.1021/ar7001073
- Yamamoto H, Mitsuhashi K, Kimoto N, Esaki N, Kobayshi Y. A novel NADH-dependent carbonyl reductase from Kluyveromyces aestuarii and comparison of NADH-regeneration system for the synthesis of ethyl (S)-4-chloro-3-hydroxybutanoate. Biosci Biotechnol Biochem 2004; 68:638-49; PMID: 15056898; http://dx.doi.org/10.1271/bbb.68.638
- Yamamoto H, Matsuyama A, Kobayashi Y. Synthesis of ethyl (S)-4-chloro-3-hydroxybutanoate using fabG-homologues. Appl Microbiol Biotechnol 2003; 61:133-9; PMID: 12655455; http://dx.doi.org/10.1007/s00253-002-1188-0
- Wang Q, Shen L, Ye T, Cao D, Chen R, Pei X, Xie T, Li Y, Gong W, Yin X. Overexpression and characterization of a novel (S)-specific extended short-chain dehydrogenase/reductase from Candida parapsilosis. Bioresour Technol 2012; 123:690-4; PMID: 22939596; http://dx.doi.org/10.1016/j.biortech.2012.07.060
- Ye Q, Yan M, Yao Z, Xu L, Cao H, Li Z J, Chen Y, Li S Y, Bai J X, Xiong J, et al. A new member of the short-chain dehydrogenases/reductases superfamily: purification, characterization and substrate specificity of a recombinant carbonyl reductase from Pichia stipitis. Bioresour Technol 2009; 100:6022-7; PMID: 19574038; http://dx.doi.org/10.1016/j.biortech.2009.06.014
- Wang L J, Li C X, Ni Y, Zhang J, Liu X, Xu J H. Highly efficient synthesis of chiral alcohols with a novel NADH-dependent reductase from Streptomyces coelicolor. Bioresour Technol 2011; 102:7023-8; PMID: 21570826; http://dx.doi.org/10.1016/j.biortech.2011.04.046
- He Y C, Yang Z X, Zhang D P, Tao Z C, Chen C, Chen Y T, Guo F, Xu J H, Huang L, Chen R J, et al. Biosynthesis of ethyl (S)-4-chloro-3-hydroxybutanoate by NADH-dependent reductase from E. coli CCZU-Y10 discovered by genome data mining using mannitol as cosubstrate. Appl Biochem Biotechnol 2014; 173:2042-53; PMID: 24880894; http://dx.doi.org/10.1007/s12010-014-1001-4
- He Y C, Ma C L, Xu J H, Zhou L. A high-throughput screening strategy for nitrile-hydrolyzing enzymes based on ferric hydroxamate spectrophotometry. Appl Microbiol Biotechnol 2011; 89:817-23; PMID: 21038095; http://dx.doi.org/10.1007/s00253-010-2977-5
- He Y C, Zhang D P, Tao Z C, Zhang X, Yang Z X. Discovery of a reductase-producing strain recombinant E. coli CCZU-A13 using colorimetric screening and its whole cell-catalyzed biosynthesis of ethyl (R)-4-chloro-3-hydroxybutanoate. Bioresour Technol 2014; 172:342-8; PMID: 25277262; http://dx.doi.org/10.1016/j.biortech.2014.09.062
- Nakagawa A, Minami H, Kim J S, Koyanagi T, Katayama T, Sato F, Kumagai H. Bench-top fermentative production of plant benzylisoquinoline alkaloids using a bacterial platform. Bioeng Bugs. 2012; 3(1):49-53; PMID: 22179145; http://dx.doi.org/10.1038/ncomms1327