Abstract
Glycerol is an interesting feedstock for biomaterials such as biofuels and bioplastics because of its abundance as a by-product during biodiesel production. Here we demonstrate glycerol metabolism in the nitrogen-fixing species Azotobacter vinelandii through metabolomics and nitrogen-free bacterial production of biopolymers, such as poly-d-3-hydroxybutyrate (PHB) and alginate, from glycerol. Glycerol-3-phosphate was accumulated in A. vinelandii cells grown on glycerol to the exponential phase, and its level drastically decreased in the cells grown to the stationary growth phase. A. vinelandii also overexpressed the glycerol-3-phosphate dehydrogenase gene when it was grown on glycerol. These results indicate that glycerol was first converted to glycerol-3-phosphate by glycerol kinase. Other molecules with industrial interests, such as lactic acid and amino acids including γ-aminobutyric acid, have also been accumulated in the bacterial cells grown on glycerol. Transmission electron microscopy revealed that glycerol-grown A. vinelandii stored PHB within the cells. The PHB production level reached 33% per dry cell weight in nitrogen-free glycerol medium. When grown on glycerol, alginate-overproducing mutants generated through chemical mutagenesis produced 2-fold the amount of alginate from glycerol than the parental wild-type strain. To the best of our knowledge, this is the first report on bacterial production of biopolymers from glycerol without addition of any nitrogen source.
Introduction
Azotobacter vinelandii is a free living, nitrogen-fixing bacterium.Citation1,2 One of the remarkable characteristics of this species is that it can grow sufficiently in a nitrogen-free minimal medium.Citation3 In A. vinelandii, nitrogenases prerequisite for nitrogen fixation catalyze the reduction of nitrogen to ammonia using a large amount of energy derived from ATP hydrolysis.Citation4,5 In the recently determined genome sequence of A. vinelandii, 3 different types of nitrogenases have been identified.Citation2 In addition, it has been established that A. vinelandii has the potential to produce industrially useful biopolymers, including extracellular alginate and intracellular poly-d-3-hydroxybutyrate (PHB).Citation6-9 Alginate is a linear polysaccharide consisting of (1–4)-β-d-mannuronic acid and α-l-guluronic acid. Commercially available alginate is classically derived from seaweed, although 2 bacterial genera, Pseudomonas and Azotobacter, are expected to be potential alginate producers.Citation8 PHB belongs to the polyhydroxyalkanoate (PHA) family of polyesters, and many bacterial species accumulate PHAs as intracellular granules for energy storage.Citation10 PHAs are also promising alternatives to plastics because of their biodegradability, biocompatibility, and thermoplasticity.Citation6,11 Hence, A. vinelandii is considered to be an attractive bacterium for production of 2 industrially useful biopolymers, alginate and PHB, in the absence of nitrogen sources.Citation6,9,12-14
The biosynthetic pathways for alginate and PHB production in A. vinelandii have previously been reviewed by Galindo et al.Citation6 As shown in , alginate is synthesized from fructose 6-phosphate by many enzymes encoded by the alg cluster,Citation8,15 whereas PHB is synthesized in 3 steps from acetyl-CoA and 3 phb genes are essential for synthesis.Citation16 The regulatory mechanisms for the production of these biopolymers have been analyzed.Citation16-25 Thus, the biopolymers are expected to be produced from excess and/or unused resource by A. vinelandii.
Figure 1. Putative synthetic pathway for PHB and alginate from glycerol in A. vinelandii. The genes involved in glycerol metabolism are also indicated.
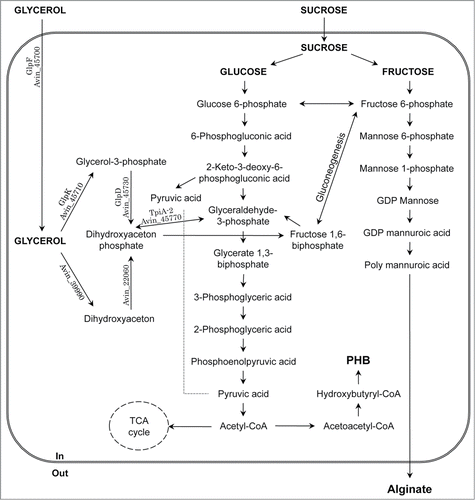
A. vinelandii is known to assimilate various carbon sources. Sucrose, glucose, fructose, mannose, sorbitol, mannitol, glycerol, gluconate, and acetate can all be used as the sole carbon source for cell growth.Citation26,27 Furthermore, some of them are also used for biopolymer production.Citation26 However, little information exists on bacterial glycerol metabolism including biopolymer production in a nitrogen-free environment. Glycerol is generated as a major by-product during biodiesel production, and its efficient utilization is now sought in various areas of food, pharmaceutical, agricultural, and environmental research. Moreover, microorganism-mediated conversion of glycerol to other valuable materials is being developed worldwide. Production of hydrogen, ethanol, butanol, 1,3-propanediol, propionic acid, and PHAs has previously been undertaken using bacteria.Citation28,29
To the best of our knowledge, no report exists on glycerol utilization in a nitrogen-free environment. Moreover, glycerol metabolism and production of biopolymers from glycerol in A. vinelandii remain to be clarified. The present study involves metabolomics-based identification of the glycerol metabolic pathway in A. vinelandii and the bacterial production of biopolymers from glycerol in a nitrogen-free environment.
Results and Discussion
Sucrose and glycerol metabolism
Metabolites in A. vinelandii cells grown on sucrose or glycerol were analyzed by a capillary electrophoresis time-of-flight mass spectrometry (CE-TOFMS) (). Small amounts of glucose-, fructose-, and glycerol-related metabolites, but not glycerol-3-phosphate accumulated in the cells (). Considerable amounts (400 pmol/OD600 ml) of glycerol-3-phosphate were detected in the glycerol-grown cells collected at the exponential growth phase, whereas its level drastically decreased (82 pmol/OD600 ml) in cells collected at the stationary growth phase. Moreover, the dihydroxyacetone phosphate level in the glycerol-grown cells was much higher than that in the sucrose-grown cells. These results suggest that glycerol was first catabolized to glycerol-3-phosphate, then to dihydroxyacetone phosphate, and finally converged into the glycolytic pathway. Because cell growth on glycerol was slower than that on sucrose as described later, accumulation of glycerol-3-phosphate was considered to be a rate-limiting step.
Table 1. Intracellular metabolites determined through metabolomics
In the recently determined genome sequence of A. vinelandii strain DJ,Citation2 putative genes for glycerol uptake and degradation were found at a locus containing 4 genes, glpF, glpK, glpR, and glpD. GlpF and GlpR are annotated as putative glycerol-uptake and -repressor proteins, respectively, whereas GlpK and GlpD are annotated as glycerol kinase and glycerol-3-phosphate dehydrogenase, respectively. Although GlpK and GlpD are classified into a group involved in phospholipid metabolism according to genome annotation, metabolomics in the present study demonstrated that glycerol and glycerol-3-phosphate were substrates of GlpK and GlpD, respectively, and that both enzymes were necessary for glycerol metabolism.
Two pathways were postulated for the conversion of glycerol to dihydroxyacetone phosphate. One is through glycerol-3-phosphate and the other through dihidroxyaceton. A number of microorganisms can use glycerol as the sole carbon source through dihydroxyacetone.Citation29-31 Furthermore, the genome sequence of A. vinelandii revealed the presence of glycerol dehydrogenase- and dihydroxyacetone kinase-like genes (). Hence, a dehydrogenase for glycerol and one for glycerol-3-phosphate were assayed to determine the main glycerol assimilation pathway. However, no activity of either enzyme was detected in the bacterial cell extract, possibly due to their low protein expression level. Hence, transcription levels of the genes of the 2 dehydrogenases were monitored by quantitative PCR (). As shown in , the total RNA of bacteria grown in all conditions was extracted without degradation. In the case of the glycerol-3-phosphate dehydrogenase gene (), the cells grown to the exponential growth phase showed higher gene expression than those grown to the stationary growth phase. In bacteria grown to the exponential growth phase, gene expression in the glycerol medium was 38-fold higher than that of bacteria grown in the sucrose medium. In contrast, the glycerol dehydrogenase gene was transcribed at basal levels in bacteria grown to the exponential growth phase in media (). The expression of glycerol dehydrogenase in all cases tested was extremely low, but near the detectable limit for our experimental conditions (data not shown). These metabolomic and quantitative PCR results demonstrate that the glycerol-3-phosphate pathway is predominant in A. vinelandii.
Figure 2. Gene expression of glycerol-3-phosphate and glycerol dehydrogenases. (A) Total RNA from A. vinelandii grown in G-MB or S-MB to the exponential and stationary growth phases. (B) Relative gene expression of glycerol-3-phosphate (left) and glycerol dehydrogenases (right). For quantitative PCR, cDNA synthesized from was used as a template in all samples. The expression levels of both genes in S-MB at the stationary phase were standardized as the relative expression of 1.
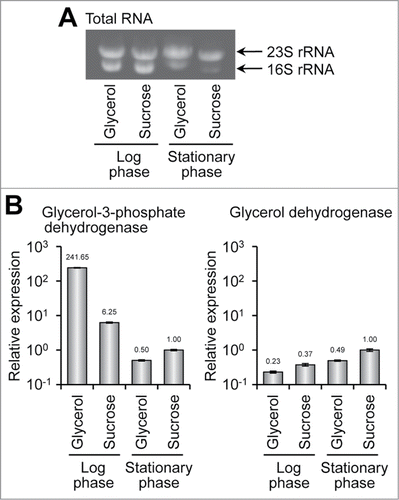
Sucrose metabolites, such as fructose 6-phosphate, mannose 6-phosphate, mannose 1-phosphate, and GDP mannose, were detected even in glycerol-grown A. vinelandii (). This result indicates that gluconeogenesis, which involves the conversion of fructose 1,6-biphosphate to fructose 6-phosphate, occurred in the bacteria. In addition, occurance of gluconeogenesis in A. vinelandii demonstrates its potential for producing alginate concomitantly with PHB from glycerol, which is described later.
No conspicuous rate-limiting metabolites were identified between fructose 1,6-biphosphate and acetyl CoA (). However, accumulation of pyruvic acid (42.5 pmol/OD600 ml) was observed in glycerol-grown cells collected at the exponential growth phase. Pyruvic acid is an important precursor for many metabolites, including ethanol, acetyl CoA, and lactic acid.Citation32 In the present study, A. vinelandii produced large amounts of lactic acid in all cases, especially in glycerol-grown cells (), and levels of several amino acids accumulated in glycerol-grown cells were higher than those in sucrose-grown cells. Both glutamine and glutamic acid were considerably produced in all cases, especially in glycerol-grown cells collected at the exponential growth phase. Arginine, lysine, and proline are synthesized from glutamic acid in most bacteria. A similar accumulation profile of these amino acids was observed in A. vinelandii, as shown in . Thus, A. vinelandii demonstrated the potential for amino acid production under nitrogen-free conditions. Furthermore, γ-aminobutyric acid (GABA), a neurotransmitter of clinical interest,Citation33 was stored in the cells grown to the exponential growth phase in sucrose and glycerol. Because bacteria-produced GABA is known as a molecule of intracellular pH management due to decarboxylation of glutamic acid,Citation34 this molecule is suggested to play an important role in the neutralization of organic acids accumulated in the cells.
Table 2. Intracellular amino acids and other organic acids
Metabolomic analysis performed in the present study supported the role of the metabolic pathway predicted previously, as shown in . In addition, based on results of the present study, A. vinelandii is promising as a potential producer of many useful materials, such as amino acids, GABA, lactic acid, and biopolymers, in a nitrogen-free environment.
PHB production
PHB accumulation was investigated in wild-type (WT) A. vinelandii grown on sucrose or glycerol. In addition, the mutant ΔalgD cells, which have a disrupted alginate synthetic gene algD, were also subjected to the PHB assay because PHB and alginate productions are thought to be competitive. First, intracellular PHB granules were monitored by transmission electron microscopy (TEM) (). Similar to many PHB-producing bacteria, WT cells produced white and globular PHB granules, which were found in both sucrose- and glycerol-grown cells. On the other hand, globular PHB granules were scarcely observed and apparently degraded in ΔalgD cells.
Figure 3. PHB granules in A. vinelandii cells revealed by TEM. Bacteria were cultured for 72 h in S-MB or G-MB as indicated.
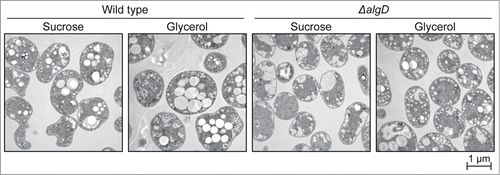
The time course of cell growth and PHB production are shown in . In the sucrose medium, WT and ΔalgD cells showed similar growth profiles (). Cell growth of both strains exceeded an OD600 of 14. However, the lag phase of both strains grown on glycerol was longer than that on sucrose. WT growth reached an OD600 of 6.12, whereas that of ΔalgD reached an OD600 of 16.8.
Figure 4. Time course of PHB production by A. vinelandii. (A) Cell growth. (B) PHB accumulation in dry cells. Open circles, WT grown on sucrose; closed circles, WT grown on glycerol; open triangles, ΔalgD grown on sucrose; closed triangles, ΔalgD grown on glycerol.
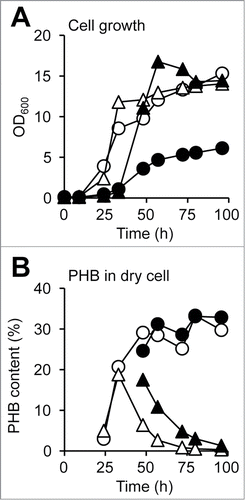
indicates the time course of intracellular PHB accumulation (per dry cell). The intracellular PHB level in WT cells grown on sucrose as well as glycerol reached approximately 33%. Interestingly, the PHB level of ΔalgD cells grown on either source initially increased, but subsequently decreased. As shown in , PHB was degraded in ΔalgD cells. A. vinelandii is known to convert from vegetative cells to cysts under unfavorable environments for growth, and PHB has been observed in the cyst-forming cells as a probable energy and carbon storage material.Citation14 Although the reasons for PHB degradation have yet to be determined, the necessity for PHB might waver due to the lack of alginate production following cyst formation. Production of PHB and alginate is closely regulated in A. vinelandii.Citation10 The results obtained herein may provide valuable hints on the relationships among alginate, PHB, and cyst formation.
Although A. vinelandii grown on glucose together with nitrogen sources synthesizes much PHB (74% per dry cell),Citation35 and some bacteria such as Burkholderia cepacia,Citation36 Chelatococcus daeguensis,Citation37 Cupriavidus necator (formerly Ralstonia eutropha),Citation38,39 Paracoccus denitrificans,Citation40 Pseudomonas oleovorans,Citation41 and Zobellella denitrificans,Citation42 have also been known to produce PHB from glycerol with nitrogen sources, the bacterial production of PHB from glycerol without a nitrogen source contributes to green chemistry.
Alginate production
The alginate production level was determined in A. vinelandii grown on sucrose or glycerol. The sucrose-grown cells obtained at the stationary phase produced alginate at 0.3–0.4 mg/ml, whereas those grown in the glycerol medium produced enhanced levels of approximately 0.5 mg/ml. To elevate the alginate production level, a random NTG-treated mutation library was used to screen alginate-overproducing mutants. Unlike for PHB, it was easy to select alginate-overproducing mutants because these mutants seemed to form high mucoid colonies. More than 100 mutants were isolated as high mucoid colonies in comparison with WT colonies.
One of the mutants (MT1) was subjected to TEM analysis after preparation of cell thin section (). Recently, A. vinelandii cells were demonstrated to be equipped with a special secretion system for alginate through formation of cell-surface blebs. These blebs containing alginate are formed on the bacterial cell surface and are subsequently released around the cells.Citation43 MT1 cells formed a large number of blebs on the cell surface (). On the other hand, few blebs were observed on WT cells (). Thus, WT cells were subjected to scanning electron microscopy (SEM) analysis. A few blebs were observed on the cell surface (). The time course of alginate secretion for WT and MT1 cells grown on glycerol is characterized in . Cell growth (3.97 at OD600) of MT1 at 96 h was lower than that (6.64 at OD600) of WT (). In contrast, alginate secretion by MT1 cells (0.87 mg/ml) was higher than that by WT cells (0.52 mg/ml) (). This result indicates that MT1 cells have the potential for more alginate production through improvement in their growth conditions.
Figure 5. Alginate-overproducing mutants of A. vinelandii. (A) TEM observation of MT1 cells grown on glycerol. (B) TEM observation of WT cells grown on glycerol. (C) SEM observation of WT cells grown on glycerol. Panels (A′ B′, and C′) are magnified views of the regions of interest indicated in panels (A, B, and C), respectively.
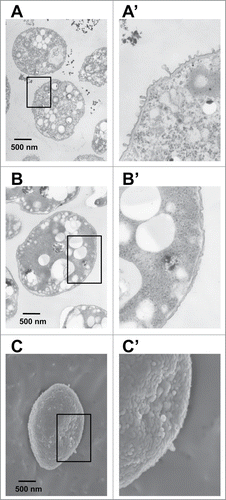
Figure 6. Alginate production from glycerol-grown A. vinelandii. (A) Cell growth. (B) Extracellular alginate. Circles, WT; triangles, alginate-overproducing mutant.
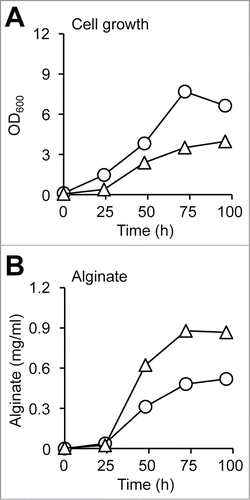
In conclusion, this is the first report on glycerol metabolism in A. vinelandii analyzed through the metabolomic approach. The results indicate that A. vinelandii grown on glycerol, but in the absence of any nitrogen source, may be useful for producing many substances, including amino acids and biopolymers (especially PHB and alginate), with industrial interests.
Materials and Methods
Bacteria and culture conditions
The bacterial strains used in the present study are listed in . Cells of WT A. vinelandii ATCC 12837 and an alginate-deficient mutant with a disruption of algD (ΔalgD)Citation43 were grown aerobically in a minimal glycerol medium, i.e., modified Burk's medium (G-MB; 20 mg/ml glycerol, 200 μg/ml NaCl, 50 μg/ml CaSO4, 200 μg/ml MgSO4 7H2O, 2.9 μg/ml Na2MoO4 2H2O, 27 μg/ml FeCl3, 0.66 mg/ml K2HPO4, and 0.16 mg/ml KH2PO4) at 30°C with agitation of 120 strokes per min. Sucrose (20 mg/ml) was also used for a carbon source instead of glycerol in G-MB (S-MB).
Table 3. Bacterial strains used in the present study
Metabolome analysis
Metabolome analysis was supported by Human Metabolome Technologies (Tsuruoka, Japan). Precultured WT cells were inoculated in 50 ml fresh S-MB or G-MB, and grown to the exponential or stationary growth phase. To reach the stationary growth phase, cells were grown in each medium for 84 h. To reach the exponential growth phase, cells were grown in S-MB and G-MB for 40 and 60 h (approximately 1.2 at OD600), respectively. The cells were harvested by centrifugation (5700 × g, 4°C, 5 min), and were washed twice with 10 ml pure water. Each cell pellet was homogenized in 2 ml methanol. After homogenization, chloroform (1.6 ml) was added to 1.6 ml of cell extract, and the mixture was well agitated. Aqueous and chloroform layers were separated by centrifugation (2300 × g, 4°C, 5 min), and the aqueous layer was subjected to ultrafiltration (9100 × g, 4°C, 120 min) using the Ultrafree-MC UFC3 LCC (molecular weight cut-off, 5000; Millipore, Bedford, MA). The filtrate was dried and resolved in 50 μl of pure water. Metabolites were identified and quantified using CE-TOFMS system (Agilent Technologies, Santa Clara, CA).
Enzyme assay
Dehydrogenases for glycerol and glycerol-3-phosphate were assayed according to a previously described method.Citation44 Briefly, WT cells were inoculated in 50 ml of fresh S-MB or G-MB, and cultured to the exponential or stationary growth phase. The cells were washed twice with 10 mM potassium phosphate buffer (pH 7.0), and resuspended in 2 ml of the same buffer. The cells were ultrasonically disrupted at 4°C and 9 kHz for 10 min (Insonator model 201M; Kubota, Tokyo, Japan). Insoluble substances were removed by centrifugation (20,000 × g, 4°C, 15 min), and the supernatant was used as the cell extract for analysis. The cell extract (20 μl) was mixed with 10 mM (final concentration) potassium phosphate buffer (pH 7.0), 5 mM substrate (glycerol or glycerol-3-phosphate), and 0.5 mM coenzyme (NAD+ or NADP+). The change in the absorbance of the reaction mixture at a wavelength of 340 nm was monitored at 30°C for 10 min.
Quantitative PCR
Total RNA extraction, in vitro cDNA synthesis, and quantitative PCR were performed to monitor the expression levels of specific genes in the bacterial samples. A. vinelandii grown in S-MB or G-MB was harvested at the exponential and stationary growth phases. Total RNA was extracted using the standard hot phenol method. DNA degradation and subsequent RNA purification were performed using the RNase-Free DNase Set (Qiagen, Tokyo, Japan) and an RNeasy Mini Kit (Qiagen), respectively. cDNA, synthesized from a 200 ng RNA sample as using the ReverTra Ace qPCR RT Kit (Toyobo, Osaka, Japan) according to the manufacturer's instructions, was used as a template. Quantitative PCR was performed using the SYBR Premix Ex Taq GC (Takara Bio, Shiga, Japan) and the LineGene instrument (Toyobo).
Electron microscopy
TEM and SEM analyses were entrusted to Tokai Electron Microscopy Analysis Co. (Nagoya, Japan). A. vinelandii was grown in S-MB or G-MB media (30 ml of working volume) for 72 h. In the case of TEM analysis, the culture and fixing solution A (2% paraformaldehyde, 2% glutaraldehyde, and 0.1 M potassium phosphate buffer, pH 7.4) were mixed in a ratio of 1:1 (total 1 ml), and stored at 4°C for 60 min. Cell pellets were collected by centrifugation (5000 × g, 4°C, 5 min). Fixing solution B (2% osmium tetroxide and 0.1 M potassium phosphate buffer, pH 7.4) was added to each cell pellet and agitated. Preparation of an ultrathin section and TEM analysis using a JEM-1200EX instrument (JEOL, Tokyo, Japan) were carried out as described previously.Citation43 In the case of SEM analysis, the bacterial cells were prefixed at 4°C for 1 h by mixing the culture with an equal volume of fixative consisting of 4% paraformaldehyde and 4% glutaraldehyde in 100 mM cacodylate buffer (pH 7.4). After centrifugation, the bacterial cells were fixed at 4°C for 24 h with 2% glutaraldehyde in 0.1 M cacodylate buffer (pH 7.4), additionally fixed at 4°C for 2 h with 1% tannic acid in 0.1 M cacodylate buffer (pH 7.4), and washed at 4°C with the same buffer. The fixed cells were treated at 4°C for 3 h with 2% osmium tetroxide in 0.1 M cacodylate buffer (pH 7.4) and dehydrated in a series of ethanol (50%, 70%, 90%, and 100%, each 30 min). After dehydration, the cells were continuously dehydrated with 100% ethanol at room temperature overnight. The cells were substituted into tert-butyl alcohol at room temperature, followed by freeze drying under vacuum. The cells were coated with a thin layer (60 nm) of osmium by an osmium plasma coater (NL-OPC80NS, Nippon Laser & Electronics Laboratory, Nagoya, Japan). The cells were observed using a scanning electron microscope (JSM-6340F, JEOL, Tokyo, Japan) at an acceleration voltage of 5.0 kV.
PHB detection
Intracellular PHB was detected according to a previously described procedure with slight modification.Citation45,46 Dried cells were treated at 100°C for 140 min with 1 ml chloroform containing 0.5% (w/v) benzoic acid as an internal standard and 1 ml methanol containing 3% sulfuric acid. After addition of 1 ml distilled water, each solution was agitated for 1 min and centrifuged (1000 ×g, 4°C, 5 min). The lower organic solvent layer of each sample was subjected to gas chromatography (GC) analysis using a GC-2014 instrument (Shimadzu, Kyoto, Japan) and a DB-5 column (30 m × 0.25 mm × 0.25 μm; Agilent Technologies). Helium was used as the carrier gas. Detector and injector temperatures were set to 275 and 230°C, respectively. Initial and end temperatures were set to 60 and 200°C, respectively, with a gradient of 8°C/min. An authentic sample of PHB (Sigma, St. Louis, MO) was also treated as described above and was used for the identification and quantitative determination of PHB.
Isolation of alginate-overproducing mutant
A. vinelandii was grown in G-MB to the exponential growth phase (approximately 0.8 at OD600), and the harvested cells obtained by centrifugation were washed with MB buffer (i.e., MB without a carbon source). To create random mutation in the species, the cells were treated with 50 μg/ml N-methyl-N′-nitro-N-nitrosoguanidine (NTG; Sigma) at 30°C for 30 min. The NTG solution was removed from the sample by centrifugation, and the cells were incubated overnight in S-MB. After colony formation on an S-MB plate, the highly mucoid cells were selected as candidates for alginate-overproducing mutants.
Alginate assay
Alginate was assayed according to the method of Knutson and Jeanes.Citation47 Briefly, the culture broth (200 μl) was mixed with 0.5 M EDTA (12 μl) and 5 M NaCl (4 μl). Cells were removed from the mixture by centrifugation (7000 ×g, room temperature, 5 min). The supernatant (87.5 μl) was mixed with an ice-chilled mixture of sulfuric acid (732.5 μl) and boric acid solution (17.5 μl; 45 mM KOH and 1 M boric acid) as well as 0.1% (w/v) carbazol (25 μl). The mixture was incubated at 55°C for 30 min, and its absorbance 530 nm was subsequently measured. The alginate concentration in the culture broth was determined based on the calibration using seaweed alginate as a standard.
Disclosure of Potential Conflicts of Interest
No potential conflicts of interest were disclosed.
Funding
This work was supported by Promotion of Basic Research Activities for Innovative Bioscience (PROBRAIN) in Japan (to KM), Grants-in-Aid from the Japan Society for the Promotion of Science (to KM and WH), and by the Research Grant (to WH) from Japan Soap and Detergent Association.
References
- Kennedy C, Toukdarian A. Genetics of azotobacters: applications to nitrogen fixation and related aspects of metabolism. Annu Rev Microbiol 1987; 41:227-58; PMID:3318669; http://dx.doi.org/10.1146/annurev.mi.41.100187.001303
- Setubal JC, dos Santos P, Goldman BS, HErtesvåg H, Espin G, LRubio LM, Valla S, Almeida NF, Balasubramanian D, Cromes L, et al. Genome sequence of Azotobacter vinelandii, an obligate aerobe specialized to support diverse anaerobic metabolic processes. J Bacteriol 2009; 191:4534-45
- Burk D, Lineweaver H. The influence of fixed nitrogen on Azotobacter. J Bacteriol 1930; 19:389-414; PMID:16559435
- Lee CC, Hu Y, Ribbe MW. Unique features of the nitrogenase VFe protein from Azotobacter vinelandii. Proc Natl Acad Sci USA 2009; 106:9209-14.
- Oelze J. Respiratory protection of nitrogenase in Azotobacter species: is a widely held hypothesis unequivocally supported by experimental evidence? FEMS Microbiol Rev 2000; 24:321-33; PMID:10978541
- Galindo E, Pena C, Núñez C, Segura D, Espín G. Molecular and bioengineering strategies to improve alginate and polydydroxyalkanoate production by Azotobacter vinelandii. Microb Cell Fact 2007; 6:7; PMID:17306024
- Page WJ, Knosp O. Hyperproduction of poly-β-hydroxybutyrate during exponential growth of Azotobacter vinelandii UWD. Appl Environ Microbiol 1989; 55:1334-9; PMID:16347925
- Remminghorst U, Rehm BH. Bacterial alginates: from biosynthesis to applications. Biotechnol Lett 2006; 28:1701-12; PMID:16912921; http://dx.doi.org/10.1007/s10529-006-9156-x
- Sabra W, Zeng AP, Deckwer WD. Bacterial alginate: physiology, product quality and process aspects. Appl Microbiol Biotechnol 2001; 56:315-25; PMID:11548998; http://dx.doi.org/10.1007/s002530100699
- Keshavarz T, Roy I. Polyhydroxyalkanoates: bioplastics with a green agenda. Curr Opin Microbiol 2010; 13:321-6; PMID:20227907; http://dx.doi.org/10.1016/j.mib.2010.02.006
- Braunegg G, Lefebvre G, Genser KF. Polyhydroxyalkanoates, biopolyesters from renewable resources: physiological and engineering aspects. J Biotechnol 1998; 65:127-61; PMID:9828458; http://dx.doi.org/10.1016/S0168-1656(98)00126-6
- Parker LT, Socolofsky MD. Central body of the Azotobacter cyst. J Bacteriol 1966; 91:297-303; PMID:4955249
- Pindar DF, Bucke C. The biosynthesis of alginic acid by Azotobacter vinelandii. Biochem J 1975; 152:617-22; PMID:179528
- Stevenson LH, Socolofsky MD. Cyst formation and poly-β-hydroxybutyric acid accumulation in Azotobacter. J Bacteriol 1966; 91:304-10; PMID:5903098
- Remminghorst U, Rehm BH. In vitro alginate polymerization and the functional role of Alg8 in alginate production by Pseudomonas aeruginosa. Appl Environ Microbiol 2006; 72:298-305; PMID:16391057
- Peralta-Gil M, Segura D, Guzmán J, Servín-González L, Espín G. Expression of the Azotobacter vinelandii poly-β-hydroxybutyrate biosynthetic phbBAC operon is driven by two overlapping promoters and is dependent on the transcriptional activator PhbR. J Bacteriol 2002; 184:5672-7; PMID:12270825
- Castañeda M, Guzmán J, Moreno S, Espín G. The GacS sensor kinase regulates alginate and poly-β-hydroxybutyrate production in Azotobacter vinelandii. J Bacteriol 2000; 182:2624-8; PMID:10762268
- Castañeda M, Sánchez J, Moreno S, Núñez C, Espín G. The global regulators GacA and σS form part of a cascade that controls alginate production in Azotobacter vinelandii. J Bacteriol 2001; 183:6787-93; PMID:11698366
- Martínez-Salazar JM, Moreno S, Nájera R, Boucher JC, Espín G Soberón-Chávez G, Deretic V. Characterization of the genes coding for the putative sigma factor AlgU and its regulators MucA, MucB, MucC, and MucD in Azotobacter vinelandii and evaluation of their roles in alginate biosynthesis. J Bacteriol 1996; 178:1800-8; PMID:8606151
- Moreno S, Nájera R, Guzmán J, Soberón-Chávez G, Espín G. Role of alternative sigma factor algU in encystment of Azotobacter vinelandii. J Bacteriol 1998; 180:2766-9; PMID:9573166
- Núñez C, León R, Guzmán J, Espín G, Soberón-Chávez, G. Role of Azotobacter vinelandii mucA and mucC gene products in alginate production. J Bacteriol 2000; 182:6550-6; PMID:11073894
- Pyla R, Kim TJ, Silva JL, Jung YS. Overproduction of poly-β-hydroxybutyrate in the Azotobacter vinelandii mutant that does not express small RNA ArrF. Appl Microbiol Biotechnol 2009; 84:717-24
- Segura D, Espín G. Mutational inactivation of a gene homologous to Escherichia coli ptsP affects poly-β-hydroxybutyrate accumulation and nitrogen fixation in Azotobacter vinelandii. J Bacteriol 1998; 180:4790-8; PMID:9733679
- Senior PJ, Dawes EA. The regulation of poly-β-hydroxybutyrate metabolism in Azotobacter beijerinckii. Biochem J 1973; 134:225-38; PMID:4723225
- Wu G, Moir AJ, Sawers G, Hill S, Poole RK. Biosynthesis of poly-β-hydroxybutyrate (PHB) is controlled by CydR (Fnr) in the obligate aerobe Azotobacter vinelandii. FEMS Microbiol Lett 2001; 194:215-20; PMID:11164311
- Horan NJ, Jarman TR, Dawes EA. Effects of carbon source and inorganic phosphate concentration on the production of alginic acid by a mutant of Azotobacter vinelandii and on the enzymes involved in its biosynthesis. J Gen Microbiol 1981; 127:185-91
- Page WJ, von Tigerstrom M. Induction of transformation competence in Azotobacter vinelandii iron-limited cultures. Can J Microbiol 1978; 24:1590-4; PMID:747819
- Coombs A. Glycerin bioprocessing goes green. Nat Biotechnol 2007; 25:953-4.
- da Silva GP, Mack M, Contiero J. Glycerol: a promising and abundant carbon source for industrial microbiology. Biotechnol Adv 2009; 27:30-9; PMID:18775486; http://dx.doi.org/10.1016/j.biotechadv.2008.07.006
- Bizzini A, Zhao C, Budin-Verneuil A, Sauvageot N, Giard JC, Auffray Y, Hartke A. Glycerol is metabolized in a complex and strain-dependent manner in Enterococcus faecalis. J Bacteriol 2010; 192:779-85; PMID:19966010
- Wang ZX, Zhuge J, Fang H, Prior BA. Glycerol production by microbial fermentation: a review. Biotechnol Adv 2001; 19:201-23; PMID:14538083; http://dx.doi.org/10.1016/S0734-9750(01)00060-X
- Wendisch VF, Bott M, Eikmanns BJ. Metabolic engineering of Escherichia coli and Corynebacterium glutamicum for biotechnological production of organic acids and amino acids. Curr Opin Microbiol 2006; 9:268-74; PMID:16617034
- Petty F. GABA and mood disorders: a brief review and hypothesis. J Affect Disord 1995; 34:275-81; PMID:8550953; http://dx.doi.org/10.1016/0165-0327(95)00025-I
- Castanie-Cornet MP, Penfound TA, Smith D, Elliott JF, Foster JW. Control of acid resistance in Escherichia coli. J Bacteriol 1999; 181:3525-35; PMID:10348866
- Page WJ, Manchak J, Rudy B. Formation of poly(hydroxybutyrate-co-hydroxyvalerate) by Azotobacter vinelandii UWD. Appl Environ Microbiol 1992; 58:2866-73; PMID:1444399
- Zhu C, Nomura CT, Perrotta JA, Stipanovic AJ, Nakas JP. Production and characterization of poly-3-hydroxybutyrate from biodiesel-glycerol by Burkholderia cepacia ATCC 17759. Biotechnol Prog 2010; 26:424-30; PMID:19953601; http://dx.doi.org/10.1002/btpr.355
- Cui B, Huang S, Xu F, Zhang R, Zhang Y. Improved productivity of poly (3-hydroxybutyrate) (PHB) in thermophilic Chelatococcus daeguensis TAD1 using glycerol as the growth substrate in a fed-batch culture. Appl Microbiol Biotechnol 2015; In press
- Cavalheiro JMBT, de Almeida MCMD, Grandfils C, da Fonseca MMR. Poly(3-hydroxybutyrate) production by Cupriavidus necator using waste glycerol. Process Biochem 2009; 44:509-15.
- Tanadchangsaeng N, Yu J. Microbial synthesis of polyhydroxybutyrate from glycerol: gluconeogenesis, molecular weight and material properties of biopolyester. Biotechnol Bioeng 2012; 109:2808-18; PMID:22566160; http://dx.doi.org/10.1002/bit.24546
- Kalaiyezhini D, Ramachandran KB. Biosynthesis of poly-3-hydroxybutyrate (PHB) from glycerol by Paracoccus denitrificans in a batch bioreactor: effect of process variables. Prep Biochem Biotechnol 2015; 45:69-83; PMID:24547951
- Ashby R, Solaiman D, Strahan G. Efficient utilization of crude glycerol as fermentation substrate in the synthesis of poly(3-hydroxybutyrate) biopolymers. J Am Oil Chem Soc 2011; 88:949-59
- Ibrahim MHA, Steinbüchel A. Poly(3-hydroxybutyrate) production from glycerol by Zobellella denitrificans MW1 via high-cell-density fed-batch fermentation and simplified solvent extraction. Appl Environ Microbiol 2009; 75:6222-31; PMID:19666728
- Hashimoto W, Miyamoto Y, Yamamoto M, Yoneyama F, Murata K. A novel bleb-dependent polysaccharide export system in the nitrogen-fixing Azotobacter vinelandii subjected to low nitrogen gas levels. Int Microbiol 2013; 16:35-44; PMID:24151780
- Matsuzawa T, Ohashi T, Hosomi A, Tanaka N, Tohda H, Takegawa K. The gld1+ gene encoding glycerol dehydrogenase is required for glycerol metabolism in Schizosaccharomyces pombe. Appl Microbiol Biotechnol 2010; 87:715-27; PMID:20396879
- Braunegg G, Sonnleitner B, Lafferty RM. A rapid gas chromatographic method for the determination of poly-3-hydroxybutyric acid in microbial biomass. Eur J Appl Microbiol Biotechnol 1978; 6:29-37; http://dx.doi.org/10.1007/BF00500854
- Tsuge T, Tanaka K, Ishizaki A. Development of a novel method for feeding a mixture of L-lactic acid and acetic acid in fed-batch culture of Ralstonia eutropha for poly-D-3-hydroxybutyrate production. J Biosci Bioeng 2001; 91:545-50; PMID:16233037
- Knutson CA, Jeanes A. Determination of the composition of uronic acid mixtures. Anal Biochem 1968; 24:482-90; PMID:5723303; http://dx.doi.org/10.1016/0003-2697(68)90155-3