Abstract
The glycolytic pathway is a main driving force in the fermentation process as it produces energy, cell component precursors, and fermentation products. Given its importance, the glycolytic pathway can be considered as an attractive target for the metabolic engineering of industrial microorganisms. However, many attempts to enhance glycolytic flux, by overexpressing homologous or heterologous genes encoding glycolytic enzymes, have been unsuccessful. In contrast, significant enhancement in glycolytic flux has been observed in studies with bacteria, specifically, Corynebacterium glutamicum. Although there has been a recent increase in the number of successful applications of this technology, little is known about the mechanisms leading to the enhancement of glycolytic flux. To explore the rational applications of glycolytic pathway engineering in biocatalyst development, this review summarizes recent successful studies as well as past attempts.
Abbreviations
ADH | = | alcohol dehydrogenase |
DHA | = | dihydroxyacetone |
DHAP | = | dihydroxyacetone phosphate |
ED | = | Entner-Doudoroff |
EMP | = | Embden-Meyerhof-Parnas |
ENO | = | enolase |
FBP | = | fructose 1,6-bisphosphate aldolase |
GAPDH | = | glyceraldehyde 3-phosphate dehydrogenase |
GLK | = | glucokinase |
GPI | = | glucose 6-phosphate isomerase |
PDC | = | pyruvate decarboxylase |
PFK | = | 6-phosphofructokinase |
PGK | = | phosphoglycerate kinase |
PGM | = | phosphoglycerate mutase |
PYK | = | pyruvate kinase |
TPI | = | triosephosphate isomerase. |
Introduction
Over the past decades, the development of various metabolic engineering technologies has facilitated the expansion of the repertoire of chemical compounds produced by microbial fermentation.Citation1,2 The basic strategy of metabolic engineering focuses largely on engineering the latter part of the metabolic pathway, specifically, the key enzymes that are directly involved in its regulation. These undesirable regulatory mechanisms, including feedback inhibition and transcriptional repression, can be circumvented by screening for feedback-resistant enzymes and simply gene overexpression. In some cases, such attempts have successfully redirected the carbon flux toward the chemicals of interest.Citation3-5 Despite its central role in sugar metabolism, the glycolytic pathway is hardly recognized as a target in metabolic engineering. This is likely because of the many unsuccessful attempts at enhancing the rate of sugar uptake.Citation6-9 In one such study aimed toward improving ethanol fermentation in Saccharomyces cerevisiae, it was demonstrated that overexpression of the genes encoding for glycolytic enzymes resulted in no positive effects on ethanol productivity.Citation7,10 In contrast, recent studies in glycolytic pathway engineering have demonstrated significant improvements in sugar consumption. While the current strategy in metabolic engineering is aimed at redirecting carbon flow toward a desired compound, the strategy in glycolytic pathway engineering is to enhance the rate of sugar uptake, which directly improves productivity of microbial catalysts.
In this review, we describe past and recent attempts in metabolic engineering, showing contrasting results regarding sugar metabolism. Subsequently, we discuss the potential factors contributing to the different results. However, the regulation mechanism of sugar metabolism is beyond the scope of this review.
Successful Studies to Enhance the Rate of Sugar Uptake by Overexpression of Glycolytic Genes
Engineering of the glycolytic pathway has been performed mainly in industrial microorganisms that have established techniques for genetic modification, including Escherichia coli, S. cerevisiae, Zymomonas mobilis, and Corynebacterium glutamicum. S. cerevisiae and C. glutamicum possess the Embden-Meyerhof-Parnas (EMP) pathway for sugar metabolism, whereas Z. mobilis lacks the gene encoding 6-phosphofructokinase (PFK), an essential enzyme in the EMP pathway, and therefore instead metabolizes sugars via the Entner-Doudoroff (ED) pathway (). E. coli possesses both pathways. The EMP pathway is the main route for sugar metabolism in E. coli, whereas sugar acids are metabolized via the ED pathway. In textbooks, 6-phosphofructokinase (PFK) and pyruvate kinase (PYK) are often described as “key regulatory enzymes” in glycolysis since, under physiological conditions, they catalyze irreversible reactions and their activities are controlled allosterically by ATP and ADP. As a result, PFK and PYK become primary targets for engineering.
Figure 1. Glycolysis via the EMP and ED pathways. Names of enzymes are underlined. Abbreviations: 6PGD, gluconate 6-phosphate dehydratase; 6PGL, 6-phosphogluconolactonase; ENO, enolase; FBA, fructose 1,6-bisphosphate aldolase; G6PDH, glucose 6-phosphate dehydrogenase; GAPDH, glyceraldehyde 3-phosphate dehydrogenase; GLK, glucokinase; GPI, glucose 6-phosphate isomerase; KDPGA, 2-keto-3-deoxy-6-phosphogluconate aldolase; PFK, 6-phosphofructokinase; PGK, phosphoglycerate kinase; PGM, phosphoglycerate mutase; PTS, phosphoenolpyruvate:carbohydrate phosphotransferase system; PYK, pyruvate kinase; TPI, triosephosphate isomerase; DHAP, dihydroxyacetone phosphate; GAP, glyceraldehyde 3-phosphate; KDPG, 2-keto-3-deoxy-6-phosphogluconate.
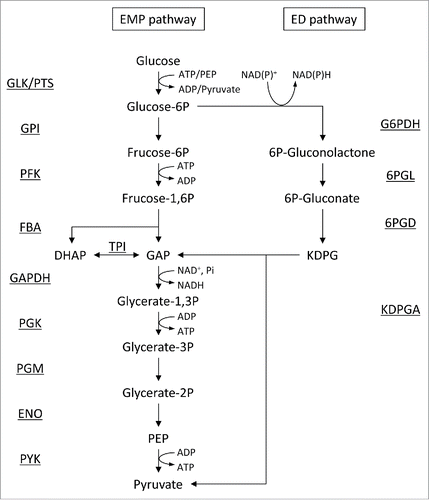
Studies with C glutamicum
C. glutamicum is a gram-positive bacterium used for industrial production of amino acids such as l-glutamic acid and l-lysine.Citation11 Normally, this microbe is cultivated aerobically for amino acid production. Under anaerobic conditions, C. glutamicum consumes sugars to produce lactic acid, succinic acid, and acetic acid, but is unable to grow during these conversion reaction.Citation12,13 The inherent abilities of C. glutamicum has enabled the development of a novel bioprocess which decouples microbial growth-phase from production-phase. A wide range of commodity chemicals including organic acids (d-lactate,Citation14 succinate,Citation15) amino acids (alanine.Citation16,17 and l-valine,Citation18,19) and fuels (ethanol.Citation20,21 and isobutanol.Citation22) can be produced by this growth-arrested bioprocess.Citation23
Successful engineering of the glycolytic pathway was achieved in the studies with C. glutamicum. Overexpression of some genes encoding glycolytic enzymes in oxygen-deprived C. glutamicum enhances conversion reaction rates (). In the first attempt aimed at improving alanine productivity, the gapA gene encoding glyceraldehyde 3-phosphate dehydrogenase (GAPDH) was selected as a target. GAPDH was chosen as it was speculated that under oxygen deprivation inhibition of GAPDH by high intracellular NADH/NAD+ ratio suppresses glucose consumption.Citation16 The wild-type strain of C. glutamicum produces small amounts of alanine from sugar under oxygen deprivation, but overexpression of alanine dehydrogenase from Lysinibacillus sphaericus results in hyperproduction of alanine by C. glutamicum.Citation16 When GAPDH activity was increased in the alanine-producing strain of C. glutamicum, alanine productivity was enhanced by 2.7-fold compared to that of the parental strain. Further investigations demonstrated that stepwise overexpression of the genes encoding glucose 6-phosphate isomerase (GPI), PFK, and PYK successively improved alanine productivity and enhanced glucose consumption.Citation17 This metabolic engineering strategy also improved productivity of other engineered strains to produce ethanol,Citation21 l-valine,Citation19 and isobutanol.Citation22 ()
Table 1. Improvement of productivity by engineering of the glycolytic pathway.
These investigations prompted the question: which enzymes in the glycolytic pathway are able to enhance glucose consumption in C. glutamicum? To answer this question, Tsuge et al.Citation24 examined the effects of overexpressing each of genes coding for the glycolytic enzymes on d-lactate productivity. A d-lactate-producing strain was developed by overexpression of d-lactate dehydrogenase from Lactobacillus delbrueckii and inactivation of endogenous l-lactate dehydrogenase. The study showed that increases in the activities of glucokinase (GLK), GAPDH, PFK, fructose 1,6-bisphosphate aldolase (FBP), and triose phosphate isomerase (TPI) enhanced glucose consumption and d-lactate productivity when a sufficient amount of d-lactate dehydrogenase activity was present. Interestingly, all these enzymes catalyze the reactions upstream of the EMP pathway.
Overexpression of the glycolytic genes also affects end-product yields. The yield of alanine was shown to increase with increasing volumetric productivity by overexpression of the glycolytic genes.Citation17 Metabolic flux via the EMP pathway increases by overexpression of glycolytic genes, whereas metabolic flux to the by-products is unchanged. The net result is that the metabolic flux toward alanine is relatively increased and the alanine yield is improved.Citation17 While this model explains the decreased yields of acetate and succinate, the major by-products of the growth-arrested bioprocess, it cannot be applied to the production of dihydroxyacetone (DHA) and glycerol. In the d-lactate-producing C. glutamicum, overexpression of glk significantly enhances glucose consumption; however, glycerol and DHA production increases at least 5-fold.Citation25 This observation suggests that the overflow in metabolism is caused by differences in the metabolic flux upstream and downstream of the EMP pathway.Citation26-28 Co-overexpression of glk and gapA reverts the DHA and glycerol yields back to levels of the non-glk-overexpressing strain. Under oxygen deprivation, glycerol is produced in C. glutamicum via DHA, from the glycolytic intermediate dihydroxyacetone phosphate (DHAP), by DHAP phosphatase HdpA and butanediol dehydrogenase.Citation27,28 The model mentioned above assumes that the metabolic flux toward the by-products remains unchanged even when the concentrations of the intracellular precursors of succinate and acetate increase. However, the metabolic fluxes to DHA and glycerol should increase with increasing concentrations of the precursors DHAP and DHA, the substrates of HdpA and ButA, respectively, because the Michaelis constant Km of HdpA and ButA for their substrates are very high.Citation27,28 Consequently, the production of DHA and glycerol are increased when overexpression of glycolytic enzymes enhances the intracellular concentrations of DHAP.
Studies with other microorganisms
Overexpression of the PFK gene in Lactococcus lactis LM0230 enhances the rates of glucose consumption and lactate production in proportion to PFK activity.Citation29 A recombinant L. lactis strain showing a 2-fold increase in PFK activity by overexpression of pfkA and its truncated gene fragment (pfk13) from Aspergillus niger exhibited a 1.5-fold increase in the maximum rate of glucose consumption under glucostat culture conditions. Interestingly, the results of Koebmann et al.Citation30 were in stark contrast to those of this aforementioned study. They constructed recombinant L. lactis strains with various levels of PFK activities, showing 1.4- to 11-fold differences compared to the wild-type level, and demonstrated that increased PFK activity has no effect on glucose consumption in L. lactis. These controversial results will be further discussed in the next section, though the results obtained in the former study clearly indicate that improvement of glucose uptake rate by engineering of the glycolytic pathway is not limited in C. glutamicum.
Increased enzyme activities of PFK and PYK in ethanol-producing E. coli KO20 moderately enhances glucose consumption under specific conditions.Citation31 Resting cells of the recombinant strain with simultaneous increased PFK and PYK activities exhibit 25% higher glucose consumption and 35% higher ethanol production compared to the parental strain when the cells are harvested from aerobic cultures. However, glycolytic flux is not enhanced when the recombinant strain is cultured anaerobically. Because the glucose consumption of E. coli KO20 is maximized under anaerobic conditions, the authors concluded that glucose flux under submaximal (aerobic) conditions can be enhanced by overexpression of glycolytic enzymes to the level observed under maximum (anaerobic) conditions. Similarly, immobilized S. cerevisiae cells with increased PFK activity show 1.3-fold higher rate of glucose consumption under aerobic conditions, but not under anaerobic conditions.Citation32 Both studies imply that the increased rate of glucose consumption, induced by the overproduction of glycolytic enzymes, does not exceed the maximum glucose consumption rate of the organisms. This idea emphasizes the importance of the glycolytic pathway engineering for improving fermentation performance of newly isolated microorganisms because optimal culture conditions of those microorganisms are hardly established especially in the early phase of strain development.
Z. mobilis is well known as an efficient ethanol producer.Citation33 This microorganism possesses the ED pathway for sugar metabolism. The recombinant strain of Z. mobilis, displaying 20% increase in glucose 6-phosphate dehydrogenase activity, shows 10% higher glycolytic flux. However, further increase in enzyme activity shows no effect on glycolytic flux.Citation34
Difference Between Success and Failure in Glycolytic Pathway Engineering
In the last section, we described studies that were successful at enhancing the rate of glucose consumption by overexpression of the glycolytic gene(s). However, historically, there have been many studies where overexpression of the glycolytic genes resulted in no positive effects on glucose consumption. In the following sections, we summarize those studies and discuss the factors determining success and failure in glycolysis engineering.
Past studies showing no improvement in glucose uptake
Schaaff et al. constructed recombinant S. cerevisiae strains overexpressing each of the genes encoding for the following 6 glycolytic enzymes: hexokinase, GPI, PFK, phosphoglycerate mutase (PGM) or PYK, and an enzyme for ethanol fermentation, pyruvate decarboxylase (PDC) or alcohol dehydrogenase (ADH).Citation10 Although enzyme activities were increased 3.7- to 13.9-fold compared to wild-type levels, overexpression of the genes had no effect on the rate of ethanol production. The authors speculated that simultaneous increase in several enzyme activities would be required to enhance the glycolytic flux. The study by Hauf et al. tested this hypothesis by simultaneously overexpressing the genes encoding for the following 7 enzymes: GAPDH, phosphoglycerate kinase (PGK), PGM, enolase (ENO), PYK, PDC, and ADH. However, the results showed that ethanol fermentation was almost equal in both recombinant and wild-type strains and only a slight increase in initial ethanol production rate was observed in the recombinant strain.Citation7
The effects of overproduction of PFK, PGK, PYK, and sugar transporter GalP in ethanol-producing E. coli KO11 were investigated.Citation35 GalP is a galactose:H+ symporter that also shows glucose uptake activity. Most of the recombinant strains showed similar levels of glucose flux, but the strains overexpressing the genes for PFK and PYK exhibited a 60% lower rate in glucose consumption. This decrease in glucose consumption rate, observed when glycolytic genes were overexpressed, was also demonstrated in the study with Z. mobilis.Citation8 This negative effect on glucose metabolism could be caused by a protein burden effect that is often observed during overproduction of proteins in recombinant cells.
By using metabolic control analysis, Jensen and co-workers investigated the role of glycolytic enzymes on the control of glycolytic flux.Citation30,36-39 They developed a series of synthetic promoters showing various expression levels and used those promoters to construct L. lactis strains with multilevel activities of glycolytic enzymes (PFK, PYK, TPI, GAPDH, ENO, PGM). These studies showed that recombinant strains with higher activities of glycolytic enzymes exhibited similar levels of glucose consumption to wild-type L. lactis. The authors concluded that none of these enzymes influenced the glycolytic flux, and these enzymes were therefore present in excess in L. lactis.
There has also been report of an unsuccessful study in oxygen deprived C. glutamicum where the gapA gene was overexpressed in succinate-producing strain.Citation40 This study aimed at improving succinate yield, which is normally limited by shortage of reducing equivalents. To achieve this, the fdh gene encoding formate dehydrogenase from Mycobacterium vaccae was introduced into the C. glutamicum genome and formate was added as a co-substrate to provide additional NADH for succinate production. The authors examined the effect of gapA overexpression in the fdh-harboring strain. The succinate yield was improved in the fdh-harboring strain in the presence of formate, but the strain enters a dormancy phase for approximately 10h after the 3h reaction. The dormancy phase disappears by overexpression of gapA. However, the glucose consumption rate of the gapA-overexpressing strain was not enhanced.
Is overexpression of glycolytic genes really effective?
Contrasting results described in the last sections make us hesitate to conclude that overexpression of the glycolytic genes is an effective means to enhance glycolytic flux. What is different between successful and failed attempts? Two studies with PFK-overproducing L. lactis conducted by different research groups will be used as examples. Papagianni et al.Citation29 demonstrated a 2-fold increase in glucose consumption by overproduction of PFK, whereas Jensen and coworkers showed that PFK has no control on glycolytic flux,Citation30 but they also found that a mutant strain having 40% reduced PFK activity showed 34% decrease in glycolytic flux compared to the wild-type strain.Citation41 This suggests that the glycolytic flux of L. lactis is sensitive to PFK activity. Moreover, the strains and culture conditions were different between the studies showing enhanced.Citation29 and unchanged.Citation30 glycolytic flux. If these differences affect the protein level of PFK in the each strain, it might provide an explanation for the contrasting results.
From the studies on S. cerevisiae described above, it appears that the glycolytic enzyme level is high enough to drive glucose metabolism in the wild-type strain. A recent kinetic model of S. cerevisiae glycolysis demonstrated that control of the glycolytic pathway is distributed among many enzyme reactions.Citation42 There is a paradoxical example in S. cerevisiae where overexpression of PGM2, which is involved in galactose metabolism, actually enhances galactose uptake by 70%.Citation43 Thus, overexpression of a glycolytic gene will enhance metabolic flux when a cell faces a deficiency in an enzyme. There is another possible factor leading to disappointed results on strain development. The effect of protein burden might be underestimated because of the difficulty to quantitatively evaluate its effects. It is possible that the negative effects of the protein burden, caused by overproduction of the glycolytic enzymes, will mask positive effects on glycolytic flux. In the study by Hauf et al., activities of PYK and PGM were increased 10- to 20-fold in S. cerevisiae. The expression levels of glycolytic enzymes are generally high, and according to published data,Citation42 PYK and PGM in S. cerevisiae were estimated to account for approximately 25 wt% among the glycolytic enzymes. Increasing protein levels of PGM and PYK by 10-fold, assuming that the protein levels of other glycolytic enzymes are unchanged, results in an increase in the total amount of glycolytic enzymes by approximately 3-fold compared to the level of the wild-type strain. Such a large increase in protein concentrations might cause a protein burden.
Although the most successful results from glycolytic pathway engineering have been observed from the studies in C. glutamicum under oxygen deprivation, under other conditions, no positive effects were found. For example, under oxygen-deprived conditions, gapA overexpression in alanine-producing C. glutamicum enhanced the rate of glucose consumption. However, under aerobic conditions, glucose consumption was almost the same as that of the reference strain.Citation16 GAPDH is not inhibited under aerobic conditions because in the presence of oxygen, the intracellular NADH/NAD+ ratio does not increase to levels that can inhibit GAPDH. Given this, it can be understood that no enhancement was observed in the study with succinate-producing C. glutamicum.Citation40 Due to redox imbalance (i.e., 2 mols of succinate produced requires more reducing equivalents that are produced from one mole of glucose), the NADH/NAD+ ratio is expected to remain low. Although no positive effects of gapA overexpression on succinate production by C. glutamicum have been reported, this does not necessarily mean that glycolytic engineering is a futile approach for improving succinate productivity. For the development of d-lactate-producing C. glutamicum strains, all the genes encoding for glycolytic enzymes were examined to find one that had positive effects on glycolytic flux.Citation24,25 This approach can be similarly applied in developing an improved strain for succinate production.
On the basis of the above discussions, the effects of glycolytic pathway engineering vary depending on the microbial species, culture conditions, and concentrations of key intracellular compounds, including NAD(P)H. To apply this metabolic engineering strategy, all the glycolytic enzymes should be explored to improve the fermentation performance of the production strain of interest. In addition, moderate levels of gene overexpression appear to be sufficient for enhancing glycolytic flux.
Outlook
Engineering of the glycolytic pathway is a developing technology, and its outcomes are currently not predictable prior to testing, since the degree of improvement of glucose uptake seems to vary depending on the host species considered. For rational modification of the glycolytic pathway, a remaining challenge is to gain a detailed understanding of the mechanism of the technology in a model organism. An ideal model for further studies would be with C. glutamicum under oxygen deprivation conditions. Under these conditions, its growth is arrested and the number of active pathways is limited, which simplifies the choice of targets in future research. In contrast, since microorganisms grow in normal fermentation processes, glucose consumption must be influenced by complex mechanisms associating the regulation of cell growth. Another reason to select C. glutamicum is that this microbe is currently used in the biotechnology industry. Consequently, knowledge from basic research can be directly transferred to industrial applications.
In E. coli, ATP demand is known to control glycolytic flux.Citation44 Significant increase in glycolytic flux is observed when the genes encoding the F1 portion of (F1F0) H+-ATP synthase are overexpressed to deplete ATP. Since growth of C. glutamicum is arrested under oxygen deprivation, under these conditions, ATP demand is supposed to be low. Therefore, the combined effects of increasing ATP demand and overexpression of the glycolytic genes in C. glutamicum are of great interest. Takeno et al.Citation46 developed a recombinant C. glutamicum strain to enhance l-lysine production by replacing wild-type NAD-dependent GAPDH with NADP-dependent GAPDH (GapN) from Streptococcus mutans, because the supply of NADPH is important for efficient l-lysine production. In this strain, NADPH is synthesized through this modified EMP pathway and l-lysine production was largely improved. There is another feature in the modified EMP pathway where no ATP is formed in the engineered pathway, whereas 2 mol of ATP is generated in the native EMP pathway from 1 mol of glucose degradation. Since this recombinant strain dose not grow on glucose as the sole carbon source because of NADPH accumulation,Citation47 it is not possible to investigate the effect of reduced ATP formation on glycolytic flux under culture conditions. However, the glycolytic flux can be determined under growth-arrested conditions by using the cells cultivated in appropriate conditions, which is yet another advantage of using C. glutamicum in glycolytic engineering research.
Another interesting approach for engineering of the glycolytic pathway was explored by Benisch and Boles,Citation48 who investigated the possibility of introducing the ED pathway to S. cerevisiae, which normally metabolizes glucose via the EMP pathway. The ED pathway generates half the amount of ATP per glucose compared to the EMP pathway, which results in reduced biomass formation. In Z. mobilis, where the ED pathway is responsible for glucose catabolism, the yield of ethanol is high due to low biomass formation.Citation33 The specific productivity of ethanol is also higher in Z. mobilis than in S. cerevisiae, because Z. mobilis requires a high flux to obtain sufficient ATP to support its growth. Unfortunately, the ED pathway did not function in S. cerevisiae due to the very low enzyme activity of bacterial 6-phosphogluconate dehydratase (PGDH), one of the key enzymes in the ED pathway. The authors speculated that inefficient loading of the iron-sulfur cluster to bacterial PGDH protein might have caused the low enzyme activity, since expression of the PGDH protein was detected in Western blot analysis after codon optimization. Development of a novel method for functional expression of the bacterial enzyme is anticipated.
Increasing concerns regarding global climate change necessitates a decrease in consumption of fossil resources. Consequently, much attention has been focused on using alternative biorefinery strategies. In this strategy, a range of bulk chemicals and fuels are produced from renewable resources, especially lignocellulosic biomass. Industrial production of cellulosic ethanol from lignocellulosic biomass started in 2014 in the United States,Citation49 but further improvement of biocatalysts in yield, titer and productivity would help expand the use of biorefineries. Thus, development of technologies to enhance glycolytic flux will be one of key challenges in this research field.
Disclosure of Potential Conflicts of Interest
No potential conflicts of interest were disclosed.
Funding
This work was partially supported by New Energy and Industrial Technology Development Organization (NEDO) of Japan and the Ministry of Economy, Trade and Industry (Japan).
References
- Borodina I, Nielsen J. Advances in metabolic engineering of yeast Saccharomyces cerevisiae for production of chemicals. Biotechnol J 2014; 9:609-20; PMID:24677744; http://dx.doi.org/10.1002/biot.201300445
- Otte KB, Hauer B. Enzyme engineering in the context of novel pathways and products. Curr Opin Biotechnol 2015; 35C:16-22; http://dx.doi.org/10.1016/j.copbio.2014.12.011
- Uhlenbusch I, Sahm H, Sprenger GA. Expression of an L-alanine dehydrogenase gene in Zymomonas mobilis and excretion of L-alanine. Appl Environ Microbiol 1991; 57:1360-6; PMID:1854197
- Colón GE, Nguyen TT, Jetten MSM, Sinskey AJ, Stephanopoulos G. Production of isoleucine by overexpression of ilvA in a Corynebacterium lactofermentum threonine producer. Appl Microbiol Biotechnol 1995; 43:482-8; http://dx.doi.org/10.1007/BF00218453
- Platteeuw C, Hugenholtz J, Starrenburg M, van Alen-Boerrigter I, de Vos WM. Metabolic engineering of Lactococcus lactis: influence of the overproduction of α-acetolactate synthase in strains deficient in lactate dehydrogenase as a function of culture conditions. Appl Environ Microbiol 1995; 61:3967-71; PMID:8526510
- Bakker BM, Michels PAM, Opperdoes FR, Westerhoff HV. What controls glycolysis in bloodstream form Trypanosoma brucei? J Biol Chem 1999; 274:14551-9; PMID:10329645; http://dx.doi.org/10.1074/jbc.274.21.14551
- Hauf J, Zimmermann FK, Müller S. Simultaneous genomic overexpression of seven glycolytic enzymes in the yeast Saccharomyces cerevisiae. Enzyme Microb Technol 2000; 26:688-98; PMID:10862874; http://dx.doi.org/10.1016/S0141-0229(00)00160-5
- Snoep JL, Yomano LP, Westerhoff HV, Ingram LO. Protein burden in Zymomonas mobilis: negative flux and growth control due to overproduction of glycolytic enzymes. Microbiology 1995; 141:2329-37; http://dx.doi.org/10.1099/13500872-141-9-2329
- Peter Smits H, Hauf J, Müller S, Hobley TJ, Zimmermann FK, Hahn-Hägerdal B, Nielsen J, Olsson L. Simultaneous overexpression of enzymes of the lower part of glycolysis can enhance the fermentative capacity of Saccharomyces cerevisiae. Yeast 2000; 16:1325-34; PMID:11015729; http://dx.doi.org/10.1002/1097-0061(200010)16:14<1325::AID-YEA627>3.0.CO;2-E
- Schaaff I, Heinisch J, Zimmermann FK. Overproduction of glycolytic enzymes in yeast. Yeast 1989; 5:285-90; PMID:2528863; http://dx.doi.org/10.1002/yea.320050408
- Ikeda M, Takeno S. Amino acid production by Corynebacterium glutamicum. In: Yukawa H, Inui M, eds. Corynebacterium glutamicum: Springer Berlin Heidelberg, 2013:107-47
- Inui M, Murakami S, Okino S, Kawaguchi H, Vertès AA, Yukawa H. Metabolic analysis of Corynebacterium glutamicum during lactate and succinate productions under oxygen deprivation conditions. J Mol Microbiol Biotechnol 2004; 7:182-96; PMID:15383716; http://dx.doi.org/10.1159/000079827
- Okino S, Inui M, Yukawa H. Production of organic acids by Corynebacterium glutamicum under oxygen deprivation. Appl Microbiol Biotechnol 2005; 68:475-80; PMID:15672268; http://dx.doi.org/10.1007/s00253-005-1900-y
- Okino S, Suda M, Fujikura K, Inui M, Yukawa H. Production of D-lactic acid by Corynebacterium glutamicum under oxygen deprivation. Appl Microbiol Biotechnol 2008; 78:449-54; PMID:18188553; http://dx.doi.org/10.1007/s00253-007-1336-7
- Okino S, Noburyu R, Suda M, Jojima T, Inui M, Yukawa H. An efficient succinic acid production process in a metabolically engineered Corynebacterium glutamicum strain. Appl Microbiol Biotechnol 2008; 81:459-64; PMID:18777022; http://dx.doi.org/10.1007/s00253-008-1668-y
- Jojima T, Fujii M, Mori E, Inui M, Yukawa H. Engineering of sugar metabolism of Corynebacterium glutamicum for production of amino acid L-alanine under oxygen deprivation. Appl Microbiol Biotechnol 2010; 87:159-65; PMID:20217078; http://dx.doi.org/10.1007/s00253-010-2493-7
- Yamamoto S, Gunji W, Suzuki H, Toda H, Suda M, Jojima T, Inui M, Yukawa H. Overexpression of genes encoding glycolytic enzymes in Corynebacterium glutamicum enhances glucose metabolism and alanine production under oxygen deprivation conditions. Appl Environ Microbiol 2012; 78:4447-57; PMID:22504802; http://dx.doi.org/10.1128/AEM.07998-11
- Hasegawa S, Uematsu K, Natsuma Y, Suda M, Hiraga K, Jojima T, Inui M, Yukawa H. Improvement of the redox balance increases L-valine production by Corynebacterium glutamicum under oxygen deprivation. Appl Environ Microbiol 2012; 78:865-75; PMID:22138982; http://dx.doi.org/10.1128/AEM.07056-11
- Hasegawa S, Suda M, Uematsu K, Natsuma Y, Hiraga K, Jojima T, Inui M, Yukawa H. Engineering of Corynebacterium glutamicum for high-yield L-valine production under oxygen deprivation conditions. Appl Environ Microbiol 2013; 79:1250-7; PMID:23241971; http://dx.doi.org/10.1128/AEM.02806-12
- Inui M, Kawaguchi H, Murakami S, Vertès AA, Yukawa H. Metabolic engineering of Corynebacterium glutamicum for fuel ethanol production under oxygen-deprivation conditions. J Mol Microbiol Biotechnol 2004; 8:243-54; PMID:16179801; http://dx.doi.org/10.1159/000086705
- Jojima T, Noburyu R, Sasaki M, Tajima T, Suda M, Yukawa H, Inui M. Metabolic engineering for improved production of ethanol by Corynebacterium glutamicum. Appl Microbiol Biotechnol 2015; 99:1165-72; PMID:25421564; http://dx.doi.org/10.1007/s00253-014-6223-4
- Yamamoto S, Suda M, Niimi S, Inui M, Yukawa H. Strain optimization for efficient isobutanol production using Corynebacterium glutamicum under oxygen deprivation. Biotechnol Bioeng 2013; 110:2938-48; PMID:23737329; http://dx.doi.org/10.1002/bit.24961
- Jojima T, Inui M, Yukawa H. Biorefinery applications of Corynebacterium glutamicum. In: Yukawa H, Inui M, eds. Corynebacterium glutamicum: Springer Berlin Heidelberg, 2013:149-72
- Tsuge Y, Yamamoto S, Suda M, Inui M, Yukawa H. Reactions upstream of glycerate-1,3-bisphosphate drive Corynebacterium glutamicum D-lactate productivity under oxygen deprivation. Appl Microbiol Biotechnol 2013; 97:6693-703; PMID:23712891; http://dx.doi.org/10.1007/s00253-013-4986-7
- Tsuge Y, Yamamoto S, Kato N, Suda M, Vertès AA, Yukawa H, Inui M. Overexpression of the phosphofructokinase encoding gene is crucial for achieving high production of D-lactate in Corynebacterium glutamicum under oxygen deprivation. Appl Microbiol Biotechnol 2015; 99:4679-89; PMID:25820644; http://dx.doi.org/10.1007/s00253-015-6546-9
- Dominguez H, Nezondet C, Lindley ND, Cocaign M. Modified carbon flux during oxygen limited growth of Corynebacterium glutamicum and the consequences for amino acid overproduction. Biotech Lett 1993; 15:449-54; http://dx.doi.org/10.1007/BF00129316
- Jojima T, Igari T, Gunji W, Suda M, Inui M, Yukawa H. Identification of a HAD superfamily phosphatase, HdpA, involved in 1,3-dihydroxyacetone production during sugar catabolism in Corynebacterium glutamicum. FEBS lett 2012; 586:4228-32; PMID:23108048; http://dx.doi.org/10.1016/j.febslet.2012.10.028
- Jojima T, Igari T, Moteki Y, Suda M, Yukawa H, Inui M. Promiscuous activity of (S,S)-butanediol dehydrogenase is responsible for glycerol production from 1,3-dihydroxyacetone in Corynebacterium glutamicum under oxygen-deprived conditions. Appl Microbiol Biotechnol 2015; 99:1427-33; PMID:25363556; http://dx.doi.org/10.1007/s00253-014-6170-0
- Papagianni M, Avramidis N. Lactococcus lactis as a cell factory: a twofold increase in phosphofructokinase activity results in a proportional increase in specific rates of glucose uptake and lactate formation. Enzyme Microb Technol 2011; 49:197-202; PMID:22112409; http://dx.doi.org/10.1016/j.enzmictec.2011.05.002
- Koebmann B, Solem C, Jensen PR. Control analysis as a tool to understand the formation of the las operon in Lactococcus lactis. FEBS J 2005; 272:2292-303; PMID:15853813; http://dx.doi.org/10.1111/j.1742-4658.2005.04656.x
- Emmerling M, Bailey JE, Sauer U. Altered regulation of pyruvate kinase or co-overexpression of phosphofructokinase increases glycolytic fluxes in resting Escherichia coli. Biotechnol Bioeng 2000; 67:623-7; PMID:10649237; http://dx.doi.org/10.1002/(SICI)1097-0290(20000305)67:5<623::AID-BIT13>3.0.CO;2-W
- Davies SE, Brindle KM. Effects of overexpression of phosphofructokinase on glycolysis in the yeast Saccharomyces cerevisiae. Biochemistry 1992; 31:4729-35; PMID:1533788; http://dx.doi.org/10.1021/bi00134a028
- Rogers PL, Lee KJ, Skotnicki ML, Tribe DE. Ethanol production by Zymomonas mobilis. Microbial Reactions: Springer Berlin Heidelberg, 1982:37-84
- Snoep JL, Arfman N, Yomano LP, Westerhoff HV, Conway T, Ingram LO. Control of glycolytic flux in Zymomonas mobilis by glucose 6-phosphate dehydrogenase activity. Biotechnol Bioeng 1996; 51:190-7; PMID: 18624328; http://dx.doi.org/10.1002/(SICI)1097-0290(19960720)51:2<190::AID-BIT8>3.0.CO;2-E
- Huerta-Beristain G, Utrilla J, Hernández-Chávez G, Bolívar F, Gosset G, Martinez A. Specific ethanol production rate in ethanologenic Escherichia coli strain KO11 is limited by pyruvate decarboxylase. J Mol Microbiol Biotechnol 2008; 15:55-64; PMID: 18349551; http://dx.doi.org/10.1159/000111993
- Solem C, Koebmann BJ, Jensen PR. Glyceraldehyde-3-phosphate dehydrogenase has no control over glycolytic flux in Lactococcus lactis MG1363. J Bacteriol 2003; 185:1564-71; PMID:12591873; http://dx.doi.org/10.1128/JB.185.5.1564-1571.2003
- Koebmann B, Solem C, Jensen PR. Control analysis of the importance of phosphoglycerate enolase for metabolic fluxes in Lactococcus lactis subsp. lactis IL1403. Syst Biol (Stevenage) 2006; 153:346-9; PMID:16986314; http://dx.doi.org/10.1049/ip-syb:20060022
- Solem C, Koebmann B, Jensen PR. Control analysis of the role of triosephosphate isomerase in glucose metabolism in Lactococcus lactis. IET Syst Biol 2008; 2:64-72; PMID:18397117; http://dx.doi.org/10.1049/iet-syb:20070002
- Solem C, Petranovic D, Koebmann B, Mijakovic I, Jensen PR. Phosphoglycerate mutase is a highly efficient enzyme without flux control in Lactococcus lactis. J Mol Microbiol Biotechnol 2010; 18:174-80; PMID:20530968; http://dx.doi.org/10.1159/000315458
- Litsanov B, Brocker M, Bott M. Toward homosuccinate fermentation: metabolic engineering of Corynebacterium glutamicum for anaerobic production of succinate from glucose and formate. Appl Environ Microbiol 2012; 78:3325-37; PMID:22389371; http://dx.doi.org/10.1128/AEM.07790-11
- Andersen HW, Solem C, Hammer K, Jensen PR. Twofold reduction of phosphofructokinase activity in Lactococcus lactis results in strong decreases in growth rate and in glycolytic flux. J Bacteriol 2001; 183:3458-67; PMID:11344154; http://dx.doi.org/10.1128/JB.183.11.3458-3467.2001
- Smallbone K, Messiha HL, Carroll KM, Winder CL, Malys N, Dunn WB, Murabito E, Swainston N, Dada JO, Khan F, et al. A model of yeast glycolysis based on a consistent kinetic characterisation of all its enzymes. FEBS lett 2013; 587:2832-41; PMID:23831062; http://dx.doi.org/10.1016/j.febslet.2013.06.043
- Bro C, Knudsen S, Regenberg B, Olsson L, Nielsen J. Improvement of galactose uptake in Saccharomyces cerevisiae through overexpression of phosphoglucomutase: example of transcript analysis as a tool in inverse metabolic engineering. Appl Environ Microbiol 2005; 71:6465-72; PMID:16269670; http://dx.doi.org/10.1128/AEM.71.11.6465-6472.2005
- Koebmann BJ, Westerhoff HV, Snoep JL, Nilsson D, Jensen PR. The glycolytic flux in Escherichia coli is controlled by the demand for ATP. J Bacteriol 2002; 184:3909-16; PMID:12081962; http://dx.doi.org/10.1128/JB.184.14.3909-3916.2002
- Nielsen DR, Leonard E, Yoon SH, Tseng HC, Yuan C, Prather KL. Engineering alternative butanol production platforms in heterologous bacteria. Metab Eng 2009; 11:262-73; PMID:19464384; http://dx.doi.org/10.1016/j.ymben.2009.05.003
- Takeno S, Murata R, Kobayashi R, Mitsuhashi S, Ikeda M. Engineering of Corynebacterium glutamicum with an NADPH-generating glycolytic pathway for L-lysine production. Appl Environ Microbiol 2010; 76:7154-60; PMID:20851994; http://dx.doi.org/10.1128/AEM.01464-10
- Komati Reddy G, Lindner SN, Wendisch VF. Metabolic engineering of an ATP-neutral Embden-Meyerhof-Parnas pathway in Corynebacterium glutamicum: growth restoration by an adaptive point mutation in NADH dehydrogenase. Appl Environ Microbiol 2015; 81:1996-2005; PMID:25576602; http://dx.doi.org/10.1128/AEM.03116-14
- Benisch F, Boles E. The bacterial Entner-Doudoroff pathway does not replace glycolysis in Saccharomyces cerevisiae due to the lack of activity of iron-sulfur cluster enzyme 6-phosphogluconate dehydratase. J Biotechnol 2014; 171:45-55; PMID:24333129; http://dx.doi.org/10.1016/j.jbiotec.2013.11.025
- Abengoa. Press release. 2014:http://static.abengoabiotech.com/wp-content/uploads/2014/09/Abengoa-Hugoton-plant-grand-opening-press-release_final_10.17.14.pdf