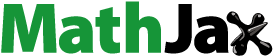
ABSTRACT
Wastewater is one of the most common by-products of almost every industrial process. Treatment of wastewater alone, before disposal, necessitates an excess of energy. Environmental concerns over the use of fossil fuels as a source of energy have prompted a surge in demand for alternative energy sources and the development of sophisticated procedures to extract energy from unconventional sources. Treatment of municipal and industrial wastewater alone accounts for about 3% of global electricity use while the amount of energy embedded in the waste is at least 2–4 times greater than the energy required to treat the same effluent. The microbial electrolysis cell (MEC) is one of the most efficient technologies for waste-to-product conversion that uses electrochemically active bacteria to convert organic matter into hydrogen or a variety of by-products without polluting the environment. This paper highlights existing obstacles and future potential in the integration of Microbial Electrolysis Cell with other processes like anaerobic digestion coupled system, anaerobic membrane bioreactor and thermoelectric micro converter.
Graphical abstract_R1
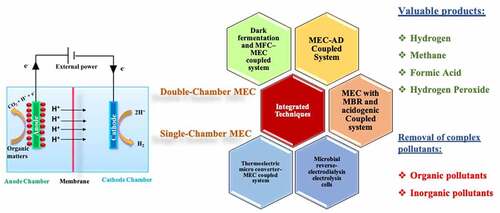
1. Introduction
With an ever-increasing population of the world, meeting the energy requirement of such a vast population has posed to be the single most critical challenge to mankind [Citation1,Citation2]. The requirement for energy has continued to go up exponentially as the population has grown over the years [Citation3]. However, our reliance on fossil fuels to generate the majority of our energy has not altered, and we continue to utilize fossil fuels such as coal, oil, and others to meet our needs [Citation4] which eventually results in the release of pollutants into the environment, necessitating further remediation [Citation5,Citation6, 63]. A lot of energy is used to run a number of industries around the globe, leaving behind by-products like wastewater [Citation7]. The different wastes generated in such processes require treatment before disposal which in turn requires a lot of energy. Thus, the production and treatment energy nexus continues [Citation8]. Wastewater is one of the most abundant by-products of practically all industries. Treatment of wastewater alone, before its disposal, requires a surplus amount of energy [Citation9]. Apart from industries, a lot of municipal wastewater is generated on a daily basis which also requires energy for its treatment. About 3% of the global electricity consumption is accounted for the treatment of municipal wastewater [Citation10]. The environmental concerns arising from the use of fossil fuels as the source of energy have also led to the increased demand for alternative sources of energy and the refined processes to harness the energy from such unconventional sources [Citation11–13]. The energy embedded in the wastewater is at least 2–4 times more than the amount of energy required for the treatment of the same wastewater. Therefore, attempts must be directed toward developing and refining such technologies that can produce energy from sources otherwise deemed waste [Citation14]. Additionally, other value-added products like chemicals, metals, clean water, etc. can be extracted from wastewater stream to maximize productivity and efficiency of the facility [Citation15]. The composition of wastewater greatly depends upon the source of generation [Citation16]. Wastewater is generally a mixture of various organic and inorganic components which can generate by-products like H2 during treatment. The different sources of wastewater can be domestic wastewater, landfill wastewater, industrial wastewater, refinery wastewater, livestock, and dairy wastewater to name a few [Citation17]. The microbes and treatment technology used greatly depend on the substrate present in the wastewater. Similarly, end product derived from treatment of wastewater also depends upon the substrate. Ditzig et al. demonstrated the production of hydrogen using the domestic wastewater as a substrate using the MECs along with the treatment of wastewater which resulted in the reduction of BOD and COD [Citation18].
Among the most efficient technology for the process of waste to product conversion is the Microbial Electrolysis Cell [Citation19,Citation20]. The MEC setup uses the exoelectronic microbes to convert the biodegradable matter like the organic matter present in the waste stream into electric current and protons [Citation21,Citation22]. The microbes are present at the anode and act upon the biodegradable waste to generate electricity and protons. The electrons are then transferred to the cathode where they reduce the protons for H2 production [Citation23,Citation24]. The type of microorganism used in the MEC depends on the substrate. For Aeromonas hydrophila, Thermincola sp, Geothrix fermentans and Gluconobacter oxydans substrate is acetate; for S. putrefaciens and S, oneidensis substrate is acetate lactate while for K. pneumoniae, Rhodoferax ferrireducens and E cloacae substrate is acetate glucose [Citation25].
The microbial electrolysis is an endothermic process and therefore for the production of H2 at the cathode, a small voltage is applied between the two electrodes to forcefully initiate the current generation. However, the applied voltage is only about 0.2–0.8 V and can be supplied by low-grade microbial fuel cells or small solar panels [Citation26]. The production of H2 using MECs has not only been hailed for commercialization because of better H2 production performance in comparison to the other H2 technologies like fermentation but also because of its higher performance efficiency than other microbial electrochemical systems like MFCs etc [Citation26].
This review aims to explore MEC applications in the following instances: an overview of Microbial Electrolysis cells for energy generation and recycling, such as hydrogen, methane, and formic acid; contaminant removal, particularly complex organic and inorganic pollutants; and resource recovery. In this review new MEC technology concepts are discussed, such as coupling with other technologies for value-added applications such as MEC-anaerobic digestion, MEC with anaerobic Membrane Bioreactor (MBR) and acidogenic, MEC-Thermoelectric micro converter, Dark fermentation, and Microbial Fuel Cell (MFC)–MEC, and MEC with Microbial reverse-electrodialysis electrolysis cell systems. Finally, challenges, prospects, and the life cycle assessment of the process are discussed.
2. Types of the microbial electrolysis system
2.1 Double-chamber MECs system
The use of a double chamber in microbial electrolysis cells, with a membrane acting as a separator, may reduce organic material and bacterial crossover from the anodic chamber to the cathodic chamber, and it also contributes to the reduction of contaminants like CO2, CH4, and H2S [Citation27]. Using a membrane also works as a separator to prevent any short-circuit. This is significant since a short length between the electrodes is needed to lower the internal strength. The double chamber draft was initially used as conceptual evidence of microbial electrolysis cells by two groups at approximately the same time frame. The transport rate of an H+ (proton) and/or OH− (hydroxyl) all over the membrane is generally restricted in the neutral state, resulting in a pH fall all over the membrane [Citation28]. The pH variation throughout the membrane may reduce the rate of H2 generation by inhibiting Electrochemically Active Bacteria (EAB) activity and increasing input strength. It was demonstrated that the double-chamber microbial electrolysis cell was not appropriate for recovering H2 from unbuffered wastewater as a result of the large pH drop. In double-chamber microbial electrolysis cells for less buffered effluent treatment, a new operation mode involving occasional polarity inversion was employed [Citation29]. shows the diagrammatic representation of a double-chambered MEC.
Different forms of double-chamber microbial electrolysis cells have been created, namely cruse-type, barrels shaped, circular like a disk, and concentric tube-shaped, by reducing the distance between the electrodes and using a porous geotextile membrane as the separator, a flat-type microbial electrolysis cell was lately developed to reduce input resistance [Citation30]. shows schematics of different types of Double Chamber MEC.
2.2 Single-chamber MECs system
By eliminating membranes from two-chamber systems and dipping both the anode and the cathode into a similar solution of one chamber, a one-chamber microbial electrolysis cell was created. The usage of single-chamber microbial electrolysis cell can help to decrease membrane resistance-related losses [Citation31]. It is simple to manufacture and autoclave, and it eliminates the problems associated with membranes, like fouling, deterioration, and massive price. The one-chamber microbial electrolysis cells have been created in a variety of designs, with the main structure constructed of diverse materials like a glass cruse and plastic block. Limited microbial electrolysis cells made from glass serum cruses can be used for the more-throughput bio-electrochemical investigation to choose the Electrochemically Active Bacteria (EAB) or possible cathode substances [Citation28]. demonstrates the diagrammatic representation of Single Chamber MEC.
The use of produced H2 by methanogenic bacteria and the re-oxidation of H2 by Electrochemically Active Bacteria are the most difficult challenges in one-chamber microbial electrolysis cells. UV irradiation was used to impede methanogenesis [Citation32]. Lately, the active gas harvesting approach was used to eliminate H2 consumption by using a vacuum and gas-permeable hydrophobic membrane to remove H2 quickly [Citation29; Citation33]. There are numerous advantages to using gas-permeable membranes. By using bubble-less hydrogen diffusion, they may improve hydrogen transfer, increase hydrogen utilization efficiency, and reduce explosion hazards. Gas-permeable membranes can be combined with either suspended or connected growth systems. Through the membrane walls, hydrogen diffuses into the bulk liquid as it travels through the lumen [Citation34].
3. Advantages and disadvantages of MEC technology
The MEC technology has proved to be an excellent process for resource recovery from the wastewater stream generated from various industries and posses several advantages over the traditional treatment methods. Some of the advantages of MEC Technology are like a) The MECs can be operated using a variety of substrates and the yields of H2 in most MECs are significantly higher, particularly in comparison to the fermentation process. Several acetates based MECs have produced up to 90% hydrogen yields, showcasing their potential in hydrogen production [Citation35]. b) The MECs can be used in combination with the traditional anaerobic digesters to increase the production of CH4 in the anaerobic digester [Citation36]. Insertion of MEC electrode into the anaerobic digester has proved to increase the production of CH4 from 60% to up to 98% and increased the carbon recovery to up to 55–56% [Citation37]. c) The MECs can be integrated into the biorefinery setup to produce hydrogen and recover chemicals. Placement of the MECs just after the pre-treatment can help utilize substrates to produce hydrogen and other value-added products and increase the overall efficiency of the fermentation process. In a similar fashion, MECs can also be coupled with the fermentation process for a higher yield of hydrogen and products [Citation38]. d) The potential of the MECs is also been evaluated for their role in the production of organic chemicals using the mechanism of microbial electrosynthesis. This can also be an alternate source for chemical generation [Citation39]. Apart from the above-mentioned advantages, other advantages include reducing the use of fossil fuels which ultimately reduces greenhouse emissions, promotes the use of renewable substrates for energy generation, reducing the burden on the environment by processing organic wastes and upholding ecological recycling, etc [Citation40].
However, there are some disadvantages of MEC Technology like a) Over a period of time, the yield of H2 decreases due to the several undesired electrons sinks in various metabolisms [Citation41]. b) The setting up of a MEC reactor is determined by the configuration of the reactor, the materials used in its making, and the type of substrate that will be used in the reactor system. These configurations are set theoretically but the actual setting may vary from the theoretically set conditions and can alter the results [Citation42]. c) For an efficient MEC, understanding the microbes and their relationship amongst themselves is essential to ensure the competition amongst the microbial species does not affect substrate utilization and product formation. Therefore, a thorough understanding of the microbes and their associated behavior is critical [Citation43]. d) The use of MECs as a treatment for industrial waste can significantly reduce the organic constituents of the waste stream, however, it faces difficulty in meeting the standards set for the effluent discharge. Therefore, it is essential to refine this process to maximize its efficiency [Citation44].
4. Coupling MEC with other technologies for value-added applications
4.1. MEC-anaerobic digestion coupled system
Anaerobic digestion occurs as a result of anaerobic microorganism metabolisms that degrade biodegradable materials and create biogas or CH4 gas [Citation31]. This is a technique that has been utilized commercially for concurrent CH4 production and waste/effluent remediation [64]. Electrochemical techniques and anaerobic digestion can be merged for improved productivity, with stillage from a certain process utilized as feedstock in the earlier and energy retrieved from the earlier utilized in the latter [Citation45]. In another study, an up-flow anaerobic sludge blanket reactor was compared to an up-flow anaerobic bio-electrochemical reactor for CH4 production from acidic distillery effluent. The up-flow anaerobic bio-electrochemical reactor was set to 300 mVolt, and both the up-flow anaerobic sludge blanket reactor and the up-flow anaerobic bio-electrochemical reactor were run in a constant state. The combination, i.e., up-flow anaerobic bio-electrochemical reactor, resulted in a considerably greater methane yield of 407 milliliters per gram CODr at 4.0-gram COD/L.day than up-flow anaerobic sludge blanket reactor (282 milliliters per gram CODr) [Citation46]. By integrating a microbial electrolysis cell technology into the reactor, De Vrieze et al. attempted to assess the rational structure underlying the enhanced operation of anaerobic digestion. They found that adding electrodes and balancing them at 0.75 and 1.205 volt enhanced the stability of the anaerobic digestion treating treacle. Methane generation in the control reactors decreased to 50% of the starting rate (on day 91), whereas it stayed constant in the microbial electrolysis cell-anaerobic digestion reactors, implying a stabilizing impact. Surprisingly, when the electrodes from these reactors were placed in the control reactors, the CH4 output jumped three to fourfold. This demonstrated that the electrochemically active biofilm generated on the surface of the electrode, instead of the electric current, should have improved the constancy of anaerobic digestion. Integrating microbial electrolysis cells and anaerobic digestion is a great illustration of how microbial electrolysis cells may be utilized in a modular fashion to improve the productivity of current technology [Citation47].
The underlying premise for organic substrate breakdown is identical in the anaerobic digestion and microbial electrolysis cell procedures. The ultimate end products differ because the ultimate electron acceptor is varied. In microbial electrolysis cells, CH4 can be created electrochemically as well as through fermentation processes. It has been proposed that combining these two procedures can help overcome the limits of separate methods. Cerrillo et al. observed that the microbial electrolysis cell system retrieved limiting products of the anaerobic digestion such as NH3, hence increasing the production of CH4 [Citation48–50]. Since few traditional anaerobic digestion techniques have been marketed, the development of integrated anaerobic digestion – microbial electrolysis cell systems appears to be feasible in the coming years.
4.2. MEC with anaerobic membrane bioreactor (MBR) (MBR and acidogenic)
Membrane bioreactors are effluent remediation systems that combine a semipermeable membrane with a suspended growth bioreactor. The following are some examples of how membrane filtering can be included in bio-electrochemical systems: 1) Between the electrodes, as a separator, 2) In the anode/cathode section, there is an inner filtration element, or 3) Prior to or after the bio-electrochemical system, there is an outer remediation technique. More effective treatment has the benefits of integrated systems including increased energy efficiency, lower investment, less fouling, and/or long-term desalination [Citation51]. Katuri et al. created the anaerobic electrochemical membrane bioreactor. A unique anaerobic remediation technology; a mix of microbial electrolysis cell and membrane filtration using nickel-based hollow-fiber membranes that are electrically conductive and porous [Citation52]. The anaerobic electrochemical membrane bioreactor was used to treat low-organic-strength effluents and solutions, as well as to retrieve biogas. The nickel-based hollow-fiber membrane fulfilled 2 purposes: as a cathode electrode for producing hydrogen and as a membrane for filtering the purified water. At a 700-megavolt applied voltage, greater than 95% of the chemical oxygen demand (starting COD: 320 mg/L) was removed, and up to 71% of the substrate energy (methane-rich biogas, 83%) was retrieved. In addition, the anaerobic electrochemical membrane bioreactor had little membrane fouling than the control reactor, which was related to the generation of hydrogen bubbles, a lower cathode voltage, and a localized higher pH at the cathode surface. Although there are numerous advantages to integrating a membrane bioreactor with a microbial electrolysis cell, more information regarding energy production and consumption in the connected system is required.
4.2.1 Acidogenic bioreactors
Acidogenic bioreactors can be paired with microbial electrolysis cells in the same way as anaerobic digestion and microbial electrolysis cells may be mixed. Babu et al. tested biohydrogen generation in a single chamber microbial electrolysis cell using CH3COO- (acetate), C3H7COO- (butyrate), and C3H5O2- (propionate) as substrates at varied voltages [Citation53]. At 600 megavolts, the highest hydrogen production rate of 2.42 millimole/hour was reported, with around 53% of synthetic acids removed [Citation54]. The same researcher’s group also paired an acidogenic bioreactor with microbial electrolysis cells to boost product retrieval and hydrogen production. microbial electrolysis cell was run at 3000 milligram/liter volatile fatty acid concentrations under various poised potentials, with the highest hydrogen production rate of 0.53 millimole/hour and 49.8% volatile fatty acid consumption observed at 600 megavolts. A unique bio-electro hydrolysis system based on self-inducing electrogenic activity was developed as a pre-treatment tool to boost hydrogen generation efficiency via the remediation of food waste in another research. Hydrolysis (1st stage) was preceded by acidogenic fermentation for hydrogen generation (2nd stage) in a two-stage coupled or hybrid system. Bio-electro hydrolysis produced more hydrogen (29.12 milliliter/hour) as a result of pre-treatment than the control (26.75 milliliter/hour). Furthermore, substrate breakdown was increased with the bio-electro hydrolysis-pre-treated substrate (a 52.42% reduction in chemical oxygen demand) as compared to the control (chemical oxygen demand removal of 43.68%) [Citation55].
Citation56,additionally developed a one-chambered microbial electrolysis cell with an acid-pre-treated biocatalyst for electro fermentation of wastewaters for further Hydrogen generation while simultaneously treating the wastewater. At 200 mVolt and 600 mVolt applied potentials, the effect of volatile fatty acid concentration (4000 milligrams/liter and 8000 milligrams/liter) on biohydrogen generation with simultaneous treatment was investigated. At 600 mVolt, the highest hydrogen production rate was 0.057 mVolt/hour, with a volatile fatty acid utilization of 68% [Citation56]. As a result, by coupling microbial electrolysis cells with acidogenic reactors, wastewater from these reactors might be transformed into usable chemicals like hydrogen.
4.3. Thermoelectric micro converter-MEC coupled system
Industrial procedures, such as those in the automobiles and steel sectors, produce waste heat as a by-product, which thermoelectric converters can use as a source of energy, and this energy is known as thermoelectricity. Thermoelectricity changers work by using a temperature gradient in the medium, which can be liquid or other solid phases. Industrial processes produce a lot of heat, which can generate a temperature gradient that can help with thermoelectricity production. Cooler temperatures, on which there is little research, can also be used to capture thermoelectricity. It is more eco-friendly to retrieve waste heat from cold temperatures. The operation of microbial electrolysis cells for the creation of hydrogen necessitates only a little quantity of electrical energy. Thermoelectricity is a natural non-conventional resource [Citation57]. As a result, combining microbial electrolysis cells with thermoelectricity can improve the long-term viability of the Hydrogen generation procedure. Chen et al. investigated the impacts of various temperature ranges on Hydrogen synthesis from CH3COO− as a carbon source in a thermoelectric micro converter-microbial electrolysis cell linked system. The thermoelectric micro converters produced electric potential and were discovered to affect the systems’ hydrogen production rate. The voltage ranged between 170 mVolt and 830 mVolt depending on the temperature (between 35°C to 55°C). At 55°C on the hot side, the highest hydrogen generation of 0.16 m3 /day and output of 2.7 mol/mol CH3COO- were reported, with an average voltage of 700 mVolt and current density ranging from 0.28 to 1.10 ampere/meter square [Citation29,Citation57].
4.4 Dark fermentation and MFC–MEC coupled system
A dark fermentation reactor, 1 to 3 microbial fuel cells, and one microbial electrolysis cell made up the combined H2 generation system. Cellulose was continually fed to the fermentation reactor, and the wastewater was accumulated and used to feed the microbial fuel cells and microbial electrolysis cells. Using fermentation wastewater as a feed, two microbial fuel cells (each 25 milliliters) linked in series to a microbial electrolysis cell (72 milliliters) achieved a highest of 0.43 Volts, resulting in a hydrogen production rate from the microbial electrolysis cell of 0.48 m3 hydrogen/m3 /day and a yield of 33.2 millimole hydrogen/gram chemical oxygen demand eliminated in the microbial electrolysis cell [Citation25; Citation29].
Microbial electrolysis cells and dark fermentation can be combined to increase total H2 production from a particular substrate [Citation58Citation59Citation60]. Because the dark fermentation procedure has a thermodynamic limit (4 mol hydrogen/mol glucose), the remaining energy from the substrate can be utilized in microbial electrolysis cells to retrieve more H2. Acidogenic bacteria transform combined organic substrates to hydrogen and volatile fatty acids as a by-product of the dark fermentation procedures. These volatile fatty acids can also be used as electrogenic bacteria substrates in microbial electrolysis cells. This coupling boosts gross H2 yields while also allowing for high substrate transformation efficiencies. When combined organic wastes (like lignocellulosic wastes) are employed as substrates, the 2-stage coupling is advantageous. Even though such substrates can be utilized natively in microbial electrolysis cells, their generation rates and procedure efficiency are insufficient when compared to volatile fatty acids. The division of energy retrieve into two processes allows for more efficient regulation of each procedure. Nevertheless, in order to attain greater efficiency, the scale of microbial electrolysis cells used must correspond to the level of dark fermentation. Separating cell biomass and adjusting the potential of hydrogen during the transition from dark fermentation to microbial electrolysis cells is still a challenge for the combination of these systems. In addition, for constant H2 generation from the 2-stage procedure, appropriate structures are required [Citation61Citation62].
Biological hydrogen generation from soluble organic components in effluents opens up the possibility of dark fermentation to make use of these previously unexplored resources. Furthermore, several thermodynamic hurdles make obtaining optimal outcomes via dark fermentation procedures difficult. Because of these thermodynamic constraints, many by-products such as CH3COO− and C4H7O2 are generated instead of hydrogen, necessitating the addition of external energy to make the process thermodynamically possible. The highest of four moles of hydrogen and two moles of CH3COO− are produced by dark fermentation of one mole of glucose. CH3COO− can also be transformed to Hydrogen via a bio catalyzed electrolysis process. A possible output of 12 moles hydrogen/mole glucose might be achieved by combining the two methods.
4.5. Microbial reverse-electrodialysis electrolysis cells (MRECs)
Reverse electrodialysis uses a succession of cation exchange membrane and anion exchange membrane to produce electricity from the salinity gradient that occurs between ocean water and pure water. Each membrane produces voltage as a result of the chemical potential contrast between pure and salt water, and the overall potential of the system is the total potential contrast from all membranes [Citation63,Citation64]. Reverse electrodialysis systems typically employ a large number of stacked cells to achieve a high level of energy recovery. The procedure results in significant capital costs due to the vast number of membranes used, as well as increased energy disadvantages from pumping water via a wide variety of cell types. When microbial electrolysis cells are combined with the reverse electrodialysis system, a microbial reverse-electrodialysis electrolysis cell is formed, in which large overpotentials can be mitigated by the oxidation of organic material by anodic biocatalyst, while the voltage drop of microbial fuel cells can be enhanced because of the salinity-driven potential of the reverse electrodialysis stack. Microbial reverse-electrodialysis electrolysis cells were used by Cusick et al. to extract salinity-gradient energy from thermolytic NH4HCO3 solutions that produced minimum waste heat (greater than 40°C). Collecting salinity gradient energy from certain thermolytic solutions makes this procedure less reliant on the supply of saltwater and pure water. The restriction of the microbial reverse-electrodialysis electrolysis cell stack configuration with ammonium bicarbonate is N2 crossing from the stack into the anode chamber, which results in NH3 contamination of the anodic solution and thus saline water reduction. Future microbial reverse-electrodialysis electrolysis cells could benefit from bipolar membranes or a minimum-salt solution in the membrane stack closest to the anode to reduce the above-mentioned losses. The highest energy retrieval with CH3COO− was found to be 30.5% with a power density of 5.6 Watt/m2 in research, which was 5 times higher than without the treatment stack. Furthermore, the reduction of power in the microbial reverse-electrodialysis electrolysis cell caused by the extra chamber near the anode might be mitigated by adding two additional membranes to boost the stack voltage. Zhu et al. developed a microbial reverse-electrodialysis electrolysis cell variant dubbed the microbial reverse-electrodialysis chemical-generation cell (MRCC). The energy generated by organic material (CH3COO−) and salt gradients were used by microbial reverse-electrodialysis chemical-generation cells to generate acid and alkali (saline water and river water imitative with various concentrations of NaCl). There was no need for an additional power supply because this system generated enough electricity (908 mWatt/m2) on its own. 1.35 millimole acid (pH 1.65 ± 0.04) and 0.59 millimole alkali (pH 11.98 ± 0.010) were produced during the fed-batch cycle performance [Citation25,Citation65].
Citation66,created a one-of-a-kind approach for H2 generation by integrating a tiny reverse electrodialysis stack (5 membrane couples) with a microbial electrolysis cell, which they dubbed microbial reverse electrodialysis electrolysis cell [Citation66]. Additional power supplies are not required in microbial reverse electrodialysis electrolysis cells since the energy for hydrogen generation comes from microbial oxidation of organic material in the anode and the saline differential between river water and seawater. During every fed-batch cycle, the microbial reverse electrodialysis electrolysis cell, which was built with 5 pairs of saltwater and river water cells, generated between 21 and 0.026 liters of gas. For an anode and cathode vessel, a cubic Lexan block with a barrels-shaped chamber (0.03 liter, 7 cm2 in cross-section) was utilized, with a glass pipe (0.02 L) affixed to the head of the cathode chamber to gather hydrogen. A microbial reverse electrodialysis electrolysis cell was formed by sandwiching 5 pairs of saltwater and river water cells between an anode-bearing exo-electrogenic bacteria and a cathode. At a saline ratio of 50, exoelectrogens provided an electrical potential from CH3COO− oxidation and lowered the anode overpotential, while the backward electrodialysis stack contributed 0.5 to 0.6 Volt. For sea and river water flow rates scale from 0.1 to 0.8 milliliter per minute, the hydrogen production rate raised from 0.8 to 1.6 m3 – hydrogen/m3 -anolyte per day. The ratio of electrons utilized for hydrogen evolution to electrons liberated by substrate oxidation, known as hydrogen recovery, ranged from 72% to 86%. Energy efficiency ranged from 58% to 64%, based on changes in salt concentrations and the depletion of organic material [Citation29]. shows the integration of Microbial Electrolysis Cell with other technologies for energy production.
Table 1. Integration of Microbial Electrolysis Cell with other technologies for energy production
5. Applications of the microbial electrolysis cell
5.1. Obtaining value-added products
5.1.1. Hydrogen production
A viable treatment solution for improved H2 generation from food waste was determined to be a one-chamber microbial electrolysis cell with negative pressure control [Citation67]. To obtain efficient H2 retrieve Food Waste was used as a substrate in a combined reactor that included one-chamber microbial electrolysis cell remediation and anaerobic digestion [Citation68]. Throughout continuous anaerobic digestion-microbial electrolysis cell operation, some investigations used a combined reactor to integrate single-chamber H2 production (511.02-milliliter hydrogen gram 1 VS), which was greater than that attained by anaerobic digestion (49.39-milliliter Hydrogen gram 1 VS). In anaerobic digestion-microbial electrolysis cells, H2 recovery was 96% and electrical energy recovery was 238.7%, accordingly [Citation69]. Key components of Food Waste [lipids, VFAs, carbohydrates, and protein] were studied to determine use of organic material to find the mechanism of H2 generation rise. The clearance efficiencies of proteins and carbohydrates in the dissolved phase in anaerobic digestion-microbial electrolysis cells were raised by four times and 2.3 times, significantly, as compared to anaerobic digestion treatment. Volatile fatty acids elimination by anaerobic digestion-microbial electrolysis cell was raised by 4.7 times, indicating that the anaerobic digestion reactor in combination with microbial electrolysis cell technologies increased the usage of the primary organic substances and hence improved H2 generation. As a result, study illustrates feasibility of minimizing Food Waste amounts while still producing bio-hydrogen. Microbial electrolysis cell technique is regarded as a resource-efficient solution for food Waste treatment with variety of engineering applications [Citation69–72].
5.1.2. Methane production
Anaerobic digestion in combination with a microbial electrolysis cell is anticipated to speed up CH4 synthesis from biomass hydrolyzate in microbial electrolysis cell-anaerobic digestion feeding with raw waste activated sludge and heat pre-treated waste activated sludge, accordingly, the methanogenesis efficiency and responsiveness of operational microbes [Citation1]. The study described a low-cost adaptive technique for increasing CH4 productivity and output by using waste-activated sludge as a substrate for microbial electrolysis cell-anaerobic digestion. The CH4 production and productivity were increased by 9.5 and 7.8 times, respectively, when the applied voltage was 0.8 Volt greater than the standard open circuit (stage I), and by 6.3 and 6.2 times, respectively, when the voltage was returned to 0 Volt [Citation73]. When raw waste-activated sludge was used in microbial electrolysis cell-anaerobic digestion, the hydrolysis-fermentation and acidogenesis were significantly boosted, leading to a greater synergy of acetogenic bacteria and hydrogenotrophic methanogens, as compared to the methods for enhancing methanogenesis with heat pre-treated waste activated sludge as substrate. As a result, microbial electrolysis cells could be employed as a viable option to boost operational microorganisms in order to produce high and steady CH4 generation in traditional anaerobic digestion while also lowering the greater operating costs associated with pre-treatment and voltage [Citation16,Citation74–76]
5.1.3. Formic acid production
Formic acid (HCOOH) is a colorless, caustic acid that dissolves in water and a variety of other polar solvents. It is primarily utilized in the textiles, pharmaceuticals, and food industries, with the tanning and leather industries accounting for the majority of its usage in 2003 [Citation77]. Meanwhile, its use as a supplement and protector in livestock feed has lately surpassed this, accounting for roughly 20% of worldwide HCOOH usage in 2019 [Citation78]. Along with its ever-expanding spectrum of applications, the need for the chemical is expected to rise by about 6% in 2019. Because of its characteristics and ease of dehydrogenation, HCOOH can be utilized to store H2. HCOOH is typically produced through the oxidation of hydrocarbons (HCs), the hydrolysis of HCONH2, the synthesis of HCOO− and the hydrolysis of HCOOCH3. CH4 and CH3OH can be used in the oxidation of hydrocarbons (HCs) to make HCOOH.
Hydrocarbon oxidation,
Formate-based production,
Methyl formate hydrolysis,
In a two-step procedure, CH3OH is oxidized to CH2O, which is then oxidized to HCOOH [see EquationEq. (1)(1)
(1) and (Equation2
(2)
(2) )]. However, employing heterogeneous catalysts, CH4 is oxidized to yield HCOOH. Although outputs are modest, employing CH4 is favorable since oxidation happens at cooler temperatures (at 60°C). until the end of the 19th century, CH2O hydrolysis was a common method of producing HCOOH throughout Europe. The use of NH3 and H2SO4, as well as the production of (NH4)2 SO4, rendered the technique less cost-effective. HCOONa [see EquationEq. (3)
(3)
(3) in the following section] and Ca (HCO2)2 [see EquationEq. (4)
(4)
(4) in the following section] are commonly used in formate generation, with H2SO4 or H3PO4 used for acidolysis. When comparing the various methods HCOOH can be made traditionally, hydrolysis of HCOOCH3 is presently the most used method. Around 90% of all HCOOH installation manufacturing plants are located along this path. It is produced in two-stage procedures in which 95% CO and 30% CH3OH are combined to make HCOOCH3, which is subsequently hydrolyzed to give HCOOCH [see EquationEq. (5)
(5)
(5) and (Equation6
(6)
(6) )] [Citation78–80]. demonstrates the formic acid generation using biotic and abiotic electrocatalysis
5.1.4. Hydrogen peroxide production
Microbial electrolysis cells can also create hydrogen peroxide (H2O2), an essential industrial chemical. The viability of producing hydrogen peroxide by combining microbial oxidation of organic materials in the anode with oxygen reduction in the cathode of microbial electrolysis cells has recently been established. This system was able of creating hydrogen peroxide at a rate of 1.17 millimole/L/hour in the aerated cathode with an applied voltage of 0.5 Volt, leading to a massive efficiency of 83% based on CH3COO− oxidation. Hydrogen peroxide synthesis in microbial electrolysis cells uses far less energy than in typical electrochemical methods, with 0.93 kWh/kg hydrogen peroxide reported in the research [Citation81]. More work should be placed toward improving hydrogen peroxide concentration for this innovation to mature. The highest hydrogen peroxide concentration currently achievable in microbial electrolysis cells is 0.13 wt.%, which seems to be an order of magnitude below the expected level for actual industrial ramifications [Citation47,Citation82,Citation83]. shows value-added products obtained by employing microbial electrolysis system.
Table 2. Value-added products obtained by employing microbial electrolysis system
5.2. Removal of complex pollutants with simultaneous resource recovery
5.2.1. Organic pollutants
Most organic pollutants, like nitrobenzene, can be eliminated in microbial fuel cells, but in microbial electrolysis cells with a little quantity of energy supply, the elimination can be greatly boosted [X. Citation70, Citation84–89]. To decrease nitrobenzene, without membrane, an up-flow microbial electrolysis cell-type reactor was created. With an outer voltage source of 0.5 Volt, up to 98% of nitrobenzene was eliminated in the cathode region, leading to the highest extraction rate of 3.5 mol/m3 /day. C6H5NH2 was the primary product of nitrobenzene destruction, with a generation rate of 3.06 mol/m3 /day. This procedure required under 0.075 kWh per mol nitrobenzene in terms of total energy [Citation90].
Chlorophenols (CPs) are hazardous, bio-refractory, and tough to break down in the native environment type of chlorinated contaminants. The possibility of ClC6H4OH (4-chlorophenol) removal in double-chamber microbial fuel cells and microbial electrolysis cells was examined by Wen et al. With only a little quantity of electricity produced, the 4-chlorophenol (ClC6H4OH) reduction procedure was possible in the double-chamber microbial fuel cell. The dichlorination accuracy of 4-chlorophenol at the cathode, on the other hand, was just 50.3%. When the reactor was performed in microbial electrolysis cell mode with 0.7 Volt voltage input, it increased to 92.5%. With an energy expenditure of 0.549-kilowatt h/mol 4-chlorophenol, the highest dichlorination rate achieved 0.38 mol per m3 /day. The energy consumption of microbial electrolysis cells for dichlorination was significantly lesser than that of standard electrochemical techniques, which needed roughly 1.17-kilowatt h/mol 4-chlorophenol. BTHs (benzothiazole derivatives) are a class of xenobiotic heterocyclic compounds that are poisonous and tough to decompose in nature. In microbial electrolysis cells, Liu et al. looked at the viability of removing Benzothiazole derivatives. In a sulfate-reducing bacteria (SRB)-enhanced microbial electrolysis cell system, Miran et al. examined the chlorinated phenol contaminant (ClC6H4OH (4-CP)) remediation. The results demonstrated that the sulfur-reducing bacteria-enriched microbial electrolysis cell effectively eliminated 4-chlorophenol and allowed for further dichlorination of dechlorinated compounds [Citation88,Citation91,Citation92].
5.2.2. Inorganic pollutants
Organic decomposition in the microbial electrolysis cell has been observed to be significantly improved by bio electrochemical augmentation of sulfate reduction [Citation93]. In the anode chamber, organic substrates were oxidized, while in the biocathode, sulfate served as an electron acceptor. According to Li et al., microbial electrolysis cells can increase anaerobic acidogenesis by processing sulfate-containing effluent, and microbial electrolysis cell-based acidogenesis can achieve better sulfate removal even at greater sulfate loadings [Citation94,Citation95]. Furthermore, Dong et al. employed a one-chamber microbial electrolysis cell with 600 milligram L-1 potassium sulfide concentrations in which the sulfide removal (K2S) rate achieved 80.7% and the main bacteria discovered was Geobacter (up to 7.35%) [Citation96]. Wang et al. introduced electron transfer efficiency via neutral red that worked as the electron transfer facilitator to increase the electrocatalytic activity of microbial electrolysis cells on sulfate elimination, and the sulfate reduction in this reactor achieved 79% [Citation97]. Exploring the ideal working conditions in real effluents is one of the difficulties of microbial electrolysis cells for sulfate elimination.
Sulfate (SO42-), perchlorate (ClO4−), and nitrate (NO3−) are among the inorganic contaminants that can be eliminated at the cathode of microbial electrolysis cells [Citation98,Citation99]. Sulfate reduction as an electron acceptor has previously been observed in a microbial electrolysis cell using a bacteria-catalyzed cathode [Citation100]. Sulfate reduction was not seen in the absence of voltage, but it improved when the voltage was introduced, reaching over 50 gm SO42-/m3 /day at 1.4 Volt. A minimum voltage of 0.7 Volt is required for such a procedure [Citation101]. Because of the increase in the potential of hydrogen in the cathode, the final product of sulfate decrease was sulfide, which was retained in the ionic form. Organic material oxidation and sulfate (SO42- reduction procedures are physically isolated in this method, preventing methanogenic bacteria from competing for electrons [Citation102]. Because no additional organic material is required, this approach can be used to remediate sulfate-polluted groundwater without affecting the biostability of potable water. The significant pH differential between the anode and cathode, as well as the less conductivity of groundwater, are the method’s principal drawbacks. In this case, treating industrial effluents with greater conductivity could result in improved remedial efficiency [Citation101,Citation103]. Due to the hazardous effects and challenging breakdown features of heavy metals, remediation of heavy metals polluted effluent has received a lot of attention lately. Microbial electrolysis cells are a cost-effective and reliable technology for treating effluent-containing heavy metals as nickel ions. Microbial electrolysis cells had a three-fold better Nickel (II) ion extraction efficiency than traditional electrolysis cells and microbial fuel cells. The applied voltage and the starting nickel concentrations in the microbial electrolysis cells are 2 important parameters in Nickel ion elimination. According to X-ray diffraction quantifications, metal nickel was detected as an outcome of nickel ion reduction on the cathode electrode. For the remediation of nickel ion-containing effluents, microbial electrolysis cells could be a viable alternative to traditional electrolysis. Numerous problems must be overcome in order to make this technique widely accessible. The system’s lengthy durability must be investigated, as the production of metal nickel on the surface of the cathode may impact the cathode reaction activity, which in turn may affect system efficiency [Citation104].
6. Life cycle assessment of microbial electrolysis cells
Life cycle assessment (LCA) is an instrument to assess the potential impacts on the environment and human health that a product can have. This method follows a cradle to grave approach and assesses not only the impacts of the product but also the impact of the acquisition of raw material, manufacturing process of the product, the transportation of the product, and the disposal of the waste generated during the manufacturing and ultimately the disposal of the product at the end [Citation105]. The LCA assesses the global warming potential, eutrophication potential, ecotoxicity, acidification potential, and the potential to harm the human health, of the manufacturing process. The LCA can help identify the stages of the process that are more potent for causing environmental damage and efforts can be made to reduce them. The LCA is an important tool for manufacturers and decision-makers to make environmentally sound decisions concerning the manufacturing processes [Citation106].
Since the use of MECs for the treatment of wastewater is a relatively newer technology, LCA is an important tool to prevent any unplanned outcomes that may rise from the new technologies. The LCA of MECs should include the evaluation of energy, financial flow, and their potential to emit greenhouse gas emissions. The main end product of the MEC is mostly hydrogen. The storage and subsequent transport of the hydrogen must also factor in as an important parameter for the LCA. This is important because it is extremely difficult to store or transport hydrogen at atmospheric pressure and hence a large amount of energy is required to compress it along with a storage facility specific to the storage of hydrogen [Citation107].
7. Role of microbial electrolysis cells toward circular bioeconomy
The world is encountering a new wave of urbanization, which has had serious consequences on natural resources and the environment [Citation108–111]. Concerns about rising energy demand and the generation of massive amounts of waste are pervasive [Citation112,Citation113]. For example, sewage produced at a rate of 40 billion liters per day results in more than 10 million tonnes of extra sludge and more than 20 million tonnes of CO2 emissions when incinerated. In this regard, humanity has only scratched the surface of the potential of biogenic waste [Citation114]. These old ways of dealing with waste not only lack system integration but also cause negative impacts on the environment on a regional and global scale [Citation115].
The circular economy concept aims to keep the value of goods, materials, and resources in the economy for as long as humanly possible while reducing waste generation [Citation116–119]. According to the ‘Ellen MacArthur Foundation’, the circular economy is a restorative, regenerative paradigm in which ‘nothing is lost and everything feeds a new cycle.’ The cascading use of biomass (Biorefinery) substantially overlaps with the circular economy concept and is largely a part of it [Citation120]. The primary goal of the bioeconomy and circular economy is to achieve better resource efficiency, resulting in a more sustainable and resource-efficient planet that relies primarily on recyclable items, hence lowering the carbon footprint [Citation121–123].
Although much work and study have been done in recent years to develop green technologies that would aid in the achievement of a circular economy, there is still much work to be done [Citation124,Citation125]. As a result, greater investment in research and development is needed to develop more efficient circular technologies [Citation126, Citation127, Renuka et al., 202; Citation128].
8. Technical challenges and prospects of microbial electrolysis cells
Microbial electrolysis cells (MECs) have the promise to be a long-term sustainable wastewater treatment technology. However, it encounters bottlenecks during scale-up. As a result, fresh scale-up experiences are needed to learn about crucial difficulties in MEC designs. The following are some of the primary obstacles that MECs encounter in scaling up:
8.1 Industrial feasibility
Despite MECs’ wide range of applications and promising future, industrial feasibility remains a major barrier, and system upscaling is necessary to evaluate this technology [66]. Recently, the scaling-up of MECs from bench to pilot-scale for production of hydrogen was described. Even though the electric energy recovery was greater than 70%, the Coulomb efficiency and the rate of hydrogen were much lower than the maximum value reported in lab-scale research, emphasizing the need for future tuning. Additionally, several MEC applications, like pollutant removal, chemical synthesis, and recovery of metal, have yet to be scaled up from a lab scale [Citation129,Citation130].
8.2 Economic challenges
Scaling up raises two important economic challenges: the materials of the electrode and reactor design. Efforts should be aimed toward the development of low-cost reactors capable of competing with a variety of different energy-generating and wastewater-treatment technologies. This, in turn, will have the additional benefit of supporting the phenomena of cost reduction with increasing manufacturing units, thus enhancing the practicability of this technology and favoring the development of more efficient MEC reactors. Cost-effective anode materials capable of efficient electron transfer from bacteria to the electrode in Microbial Fuel Cells, such as carbon fibre brush or activated carbon, might be a suitable alternative for anodes of MECs. Similarly, as a cathode catalyst, biocathode may be a promising alternative to noble metals. This is due to its low cost, excellent stability, and ecologically sound qualities. However, the effectiveness of the biocathode in pilot-scale activity remains unknown [Citation43,Citation131].
8.3 Process control and monitoring
Process control and monitoring is another significant area that has received little attention but it does have the ability to propel this technique forward on its path to practical use [Citation132]. To attain this goal, a collaborative effort of engineers, microbiologists, electrochemists, and others would be required to understand the interactions between the many parameters and factors that may influence MEC function [Citation133].
9. Conclusions
Microbial Electrolysis Cells show great potential in not only reducing the pollution quotient in the wastewater stream of the industry but also helping in aiding in the resource recovery from the industrial wastewater. This system not only helps in reducing biodegradable matter in the stream but also helps in generating electricity and hydrogen which is used as a clean fuel due to its nonpolluting properties. Due to its increased demand globally, the use of MEC has proved to be an efficient source of hydrogen generation. This system is energy positive and carbon negative and its integration with traditional technologies leads to higher output and refined processes. However, there are certain shortcomings in this process as well like the competition amongst the microbes, structural shortcomings, etc. and an extensive study and research needs to be directed in this regard to increase the efficiency of the process and maximize the yield produced in the process. Efforts to enhance reactor design and substrate selection can be done to maximize the efficiency of the setup. The MEC technology can prove to be a great asset for energy generation and resource recovery and can significantly help in reducing the dependence on conventional sources of energy like fossil fuels which are also polluting in nature. This technology can therefore pave a new way for energy generation and help meet the global energy requirement.
Acknowledgments
YK and VD are grateful to authorities of GPCB for allowing them to undergo internship with ‘A scheme on Project, Thesis or Internship at GPCB’. The authors would like to thank Gujarat Pollution Control Board for encouragement and support during manuscript preparation.
Disclosure statement
No potential conflict of interest was reported by the author(s).
Additional information
Funding
References
- Shah AV, Singh A, Mohanty SS, et al. Organic solid waste: biorefinery approach as a sustainable strategy in circular bioeconomy. Bioresour Technol. 2022;349:126835.
- Vyas S, Prajapati P, Shah AV, et al. Municipal solid waste management: dynamics, risk assessment, ecological influence, advancements, constraints and perspectives. Sci Total Environ. 2022a;814:152802.
- Vyas S, Prajapati P, Shah AV, et al. Opportunities and knowledge gaps in biochemical interventions for mining of resources from solid waste: a special focus on anaerobic digestion. Fuel. 2022b;311:122625.
- Devda V, Chaudhary K, Varjani S, et al. Recovery of resources from industrial wastewater employing electrochemical technologies: status, advancements and perspectives. Bioengineered. 2021;12:4697–4718.
- Do MH, Ngo HH, Guo W, et al. Microbial fuel cell-based biosensor for online monitoring wastewater quality: a critical review. Sci Total Environ. 2020;712:135612.
- Varjani SJ, Upasani VN. A new look on factors affecting microbial degradation of petroleum hydrocarbon pollutants. Int Biodeterior Biodegrad. 2017;120:71–83.
- Varjani S, Rakholiya P, Ng HY, et al. Microbial degradation of dyes: an overview. Bioresour Technol. 2020a;314:123728.
- Di Fraia S, Massarotti N, Vanoli L. A novel energy assessment of urban wastewater treatment plants. Energy Convers Manag. 2018;163:304–313.
- Chattopadhyay I, Banu RJ, Usman TMM, et al. Exploring the role of microbial biofilm for industrial effluents treatment. Bioengineered. 2022;2022250. Available form: https://doi.org/10.1080/21655979.2022.2044250
- Grobelak A, Grosser A, Kacprzak M, et al., Sewage sludge processing and management in small and medium-sized municipal wastewater treatment plant-new technical solution. 2019;J Environ Manage. 234:90–96.
- Asongu SA, Agboola MO, Alola AA, et al. The criticality of growth, urbanization, electricity and fossil fuel consumption to environment sustainability in Africa. Sci Total Environ. 2020;712:136376.
- Patel GB, Rakholiya P, Shindhal T, et al. Lipolytic nocardiopsis for reduction of pollution load in textile industry effluent and swiss model for structural study of lipase. Bioresour Technol. 2021;341:125673.
- Varjani SJ. Microbial degradation of petroleum hydrocarbons. Bioresour Technol. 2017;223:277–286.
- Thangamani R, Vidhya L, Varjani S. Electrochemical technologies for wastewater treatment and resource reclamation. In: Microbe mediated remediation of environmental contaminants. Elsevier; 2021. p. 381–389.
- Liu Y, Deng YY, Zhang Q, et al. Overview of recent developments of resource recovery from wastewater via electrochemistry-based technologies. Sci Total Environ. 2021;757:143901.
- Varjani S, Rakholiya P, Shindhal T, et al. Trends in dye industry effluent treatment and recovery of value added products. J Water Process Eng. 2021;39:101734.
- Kataki S, Chatterjee S, Vairale MG, et al., Constructed wetland, an eco-technology for wastewater treatment: a review on types of wastewater treated and components of the technology (macrophyte, biolfilm and substrate). 2021;J Environ Manage. 283:111986.
- Ditzig J, Liu H, Logan BE. Production of hydrogen from domestic wastewater using a bioelectrochemically assisted microbial reactor (BEAMR). Int J Hydrogen Energy. 2007;32:2296–2304.
- Janani R, Baskar G, Sivakumar K, et al. Advancements in heavy metals removal from effluents employing nano-adsorbents: way towards cleaner production. Environ Res. 2022;203:111815.
- Mohan SV, Sravan JS, Butti SK, et al. Microbial Electrochemical Technology. In: Microbial electrochemical technology: emerging and sustainable platform. Elsevier, 2019. 3–18.
- Ahmad A, Chowdhary P, Khan N, et al. Effect of sewage sludge biochar on the soil nutrient, microbial abundance, and plant biomass: a sustainable approach towards mitigation of solid waste. Chemosphere. 2022;287:132112.
- Jain A, He Z. “NEW” resource recovery from wastewater using bioelectrochemical systems: moving forward with functions. 2018;Front Environ Sci Eng. 12:1–13.
- Adesra A, Srivastava VK, Varjani S. Valorization of dairy wastes: integrative approaches for value added products. Indian J Microbiol. 61:270–278. 2021;https://doi.org/10.1007/s12088-021-00943-5
- Huang Q, Liu Y, Dhar BR. A critical review of microbial electrolysis cells coupled with anaerobic digester for enhanced biomethane recovery from high-strength feedstocks. Crit Rev Environ Sci Technol. 2020c: 1–40
- Kadier A, Jain P, Lai B, et al. Biorefinery perspectives of microbial electrolysis cells (MECs) for hydrogen and valuable chemicals production through wastewater treatment. Biofuel Res J. 2020a;7:1128–1142.
- Rousseau R, Etcheverry L, Roubaud E, et al. Microbial electrolysis cell (MEC): strengths, weaknesses and research needs from electrochemical engineering standpoint. Appl Energy. 2020b;257. Doi: 10.1016/j.apenergy.2019.113938
- Jiang Y, Liang P, Zhang C, et al. Periodic polarity reversal for stabilizing the pH in two-chamber microbial electrolysis cells. Appl Energy. 2016;165:670–675.
- Kadier A, Jiang Y, Lai B, et al. Biohydrogen production in microbial electrolysis cells from renewable resources. (CRC Press, Taylor & Francis), 2019.10.1201/9781351228138-12.
- Kadier A, Simayi Y, Abdeshahian P, et al. A comprehensive review of microbial electrolysis cells (MEC) reactor designs and configurations for sustainable hydrogen gas production. Alexandria Eng J. 2016b;55:427–443.
- Escapa A, Mateos R, Martínez EJ, et al. Microbial electrolysis cells: an emerging technology for wastewater treatment and energy recovery. From laboratory to pilot plant and beyond. Renewable Sustainable Energy Rev. 2016;55:942–956.
- Waqas M, Rehan M, Aburiazaiza AS, et al. Wastewater biorefinery based on the microbial electrolysis cell: opportunities and challenges. Elsevier B.V. 2018; 10.1016/B978-0-444-64017-8.00017-8
- Cui W, Liu G, Zeng C, et al. Improved hydrogen production in the single-chamber microbial electrolysis cell with inhibition of methanogenesis underalkaline conditions. RSC Adv. 2019;9:30207–30215.
- Lu L, Hou D, Wang X, et al. Active H2 harvesting prevents methanogenesis in microbial electrolysis cells. Environ Sci Technol Lett. 2016;3:286–290.
- Lu C, Gu P, He P, et al. Characteristics of hydrogenotrophic denitrification in a combined system of gas-permeable membrane and a biofilm reactor. J Hazard Mater. 2009;168:1581–1589.
- Wang L, Trujillo S, Liu H. Selective inhibition of methanogenesis by acetylene in single chamber microbial electrolysis cells. Bioresour Technol. 2019;274:557–560.
- Segundo-Aguilar A, González-Gutiérrez LV, Payá VC, et al., Energy and economic advantages of simultaneous hydrogen and biogas production in microbial electrolysis cells as a function of the applied voltage and biomass content. 2021;Sustain. Energy Fuels. 5:2003–2017.
- Kadier A, Parkhey P, Adekunle A, et al. Microbial electrochemical technologies. In Sonia M. Tiquia-Arashiro: Microbial electrolysis cell (mec): a versatile technology for hydrogen, value-added chemicals production and wastewater treatment. CRC Press, Taylor & Francis, 2020c. 113–127.
- Zhen G, Lu X, Kumar G, et al. Microbial electrolysis cell platform for simultaneous waste biorefinery and clean electrofuels generation: current situation, challenges and future perspectives. Prog. Energy Combust. Sci. 2017;63:119–145.
- Zou S, Qin M, Moreau Y, et al. Nutrient-energy-water recovery from synthetic sidestream centrate using a microbial electrolysis cell - forward osmosis hybrid system. J Clean Prod. 2017;154:16–25.
- Varanasi JL, Veerubhotla R, Pandit S, et al. Biohydrogen production using microbial electrolysis cell: recent advances and future prospects. Microb. Electrochem. Technol. 2019: 843–869
- San-Martín MI, Sotres A, Alonso RM, et al. Assessing anodic microbial populations and membrane ageing in a pilot microbial electrolysis cell. Int J Hydrogen Energy. 2019;44:17304–17315.
- Miller A, Singh L, Wang L, et al. Linking internal resistance with design and operation decisions in microbial electrolysis cells. Environ Int. 2019;126:611–618.
- Kadier A, Kalil MS, Chandrasekhar K, et al. Surpassing the current limitations of high purity H2 production in microbial electrolysis cell (MECs): strategies for inhibiting growth of methanogens. Bioelectrochemistry. 2018;119:211–219.
- Leicester D, Amezaga J, Heidrich E. Is bioelectrochemical energy production from wastewater a reality? identifying and standardising the progress made in scaling up microbial electrolysis cells. Renewable Sustainable Energy Rev. 2020;133:110279.
- Sadhukhan J, Lloyd JR, Scott K, et al. A critical review of integration analysis of microbial electrosynthesis (MES) systems with waste biorefineries for the production of biofuel and chemical from reuse of CO2. Renewable Sustainable Energy Rev. 2016;56:116–132.
- Feng Q, Song Y-C, Yoo K, et al., Performance of upflow anaerobic bioelectrochemical reactor compared to the sludge blanket reactor for acidic distillery wastewater treatment. 2016;J Korean Soc Environ Eng. 38:279–290.
- Aryal N, Kvist T, Ammam F, et al. An overview of microbial biogas enrichment. Bioresour Technol. 2018;264:359–369.
- Cerrillo M, Viñas M, Bonmatí A. Overcoming organic and nitrogen overload in thermophilic anaerobic digestion of pig slurry by coupling a microbial electrolysis cell. Bioresour Technol. 2016;216:362–372.
- Cerrillo M, Viñas M, Bonmatí A. Unravelling the active microbial community in a thermophilic anaerobic digester-microbial electrolysis cell coupled system under different conditions. Water Res. 2017;110:192–201.
- Zhi Z, Pan Y, Lu X, et al. Electrically regulating co-fermentation of sewage sludge and food waste towards promoting biomethane production and mass reduction. Bioresour Technol. 2019;279:218–227.
- Yuan H, He Z. Integrating membrane filtration into bioelectrochemical systems as next generation energy-efficient wastewater treatment technologies for water reclamation: a review. Bioresour Technol. 2015;195:202–209.
- Katuri KP, Werner CM, Jimenez-Sandoval RJ, et al. A novel anaerobic electrochemical membrane bioreactor (AnEMBR) with conductive hollow-fiber membrane for treatment of low-organic strength solutions. Environ Sci Technol. 2014;48:12833–12841.
- Babu ML, Subhash GV, Sarma PN, et al. Bio-electrolytic conversion of acidogenic effluents to biohydrogen: an integration strategy for higher substrate conversion and product recovery. Bioresour Technol. 2013b;133:322–331.
- Babu ML, Sarma PN, Mohan SV. Microbial electrolysis of synthetic acids for biohydrogen production: influence of biocatalyst pretreatment and pH with the function of applied potential. J Microb Biochem Technol S. 2013a;6:2.
- Chandrasekhar K, Mohan SV. Bio-electrohydrolysis as a pretreatment strategy to catabolize complex food waste in closed circuitry: function of electron flux to enhance acidogenic biohydrogen production. Int J Hydrogen Energy. 2014;39:11411–11422.
- Modestra JA, Babu ML, Mohan SV. Electro-fermentation of real-field acidogenic spent wash effluents for additional biohydrogen production with simultaneous treatment in a microbial electrolysis cell. Sep Purif Technol. 2015;150:308–315.
- Chen Y, Chen M, Shen N, et al. H2 production by the thermoelectric microconverter coupled with microbial electrolysis cell. Int J Hydrogen Energy. 2016;41:22760–22768.
- Wang A, Sun D, Cao G, et al. Integrated hydrogen production process from cellulose by combining dark fermentation, microbial fuel cells, and a microbial electrolysis cell. Bioresour Technol. 2011;102:4137–4143.
- Varjani SJ, Upasani VN. Influence of abiotic factors, natural attenuation, bioaugmentation and nutrient supplementation on bioremediation of petroleum crude contaminated agricultural soil. 2019;J Envirov Manage. 245:358–366.
- Wang XT, Zhao L, Chen C, et al. Microbial electrolysis cells (MEC) accelerated methane production from the enhanced hydrolysis and acidogenesis of raw waste activated sludge. Chem Eng J. 2021b;413:127472.
- Varanasi JL, Veerubhotla R, Pandit S, et al. Biohydrogen production using microbial electrolysis cell: recent advances and future prospects. Elsevier B.V. 2018a; DOI:10.1016/B978-0-444-64052-9.00035-2
- Zhang Y, Angelidaki I. Microbial electrolysis cells turning to be versatile technology: recent advances and future challenges. Water Res. 2014c;56:11–25.
- Zhang Y, Angelidaki I. Microbial electrolysis cells turning to be versatile technology: recent advances and future challenges. Water Res. 2014a;56:11–25.
- Zhang Y, Angelidaki I. Counteracting ammonia inhibition during anaerobic digestion by recovery using submersible microbial desalination cell. Biotechnol Bioeng. 2015;112:1478–1482.
- Zhang Y, Angelidaki I. Microbial electrolysis cells turning to be versatile technology: recent advances and future challenges. Water Res. 2014b;56:11–25.
- Kim Y, Logan BE. Hydrogen production from inexhaustible supplies of fresh and salt water using microbial reverse-electrodialysis electrolysis cells. Proc Natl Acad Sci. 2011;108:16176–16181.
- Harirchi S, Wainaina S, Sar T, et al. Microbiological insights into anaerobic digestion for biogas, hydrogen or volatile fatty acids (vfas): a review. Bioengineered. 2022;13(3):6521–6557. https://doi.org/10.1080/21655979.2022.2035986
- Feng H, Huang L, Wang M, et al. An effective method for hydrogen production in a single-chamber microbial electrolysis by negative pressure control. Int J Hydrogen Energy. 2018;43:17556–17561.
- Huang J, Feng H, Huang L, et al. Continuous hydrogen production from food waste by anaerobic digestion (AD) coupled single-chamber microbial electrolysis cell (MEC) under negative pressure. Waste Manag. 2020a;103:61–66.
- Al Afif R, Amon T. Mesophilic anaerobic co-digestion of cow manure with three-phase olive mill solid waste. energy sources, part a recover. Util. Environ. Eff. 2019;41:1800–1808.
- Velásquez F, Espitia J, Mendieta O, et al., Non-centrifugal cane sugar processing: a review on recent advances and the influence of process variables on qualities attributes of final products. 2019;J Food Eng. 255:32–40.
- Yun Y-M, Lee M-K, Im S-W, et al. Biohydrogen production from food waste: current status, limitations, and future perspectives. Bioresour Technol. 2018;248:79–87.
- Wang XT, Zhao L, Chen C, et al. Microbial electrolysis cells (MEC) accelerated methane production from the enhanced hydrolysis and acidogenesis of raw waste activated sludge. Chem Eng J. 2021a;413:127472.
- Liu C, Sun D, Zhao Z, et al. Methanothrix enhances biogas upgrading in microbial electrolysis cell via direct electron transfer. Bioresour Technol. 2019;291:121877.
- Yu Z, Leng X, Zhao S, et al. A review on the applications of microbial electrolysis cells in anaerobic digestion. Bioresour Technol. 2018;255:340–348.
- Zakaria BS, Dhar BR. Progress towards catalyzing electro-methanogenesis in anaerobic digestion process: fundamentals, process optimization, design and scale-up considerations. Bioresour Technol. 2019;289:121738.
- Bulushev DA, Ross JRH. Towards sustainable production of formic acid. ChemSusChem. 2018;11:821–836.
- Okoroafor T, Haile S, Velasquez-Orta S. Life cycle assessment of microbial electrosynthesis for commercial product generation. 2021;J Hazardous, Toxic, Radioact Waste. 25:4020062.
- Saavalainen P, Turpeinen E, Omodara L, et al. Developing and testing a tool for sustainability assessment in an early process design phase–Case study of formic acid production by conventional and carbon dioxide-based routes. J Clean Prod. 2017;168:1636–1651.
- Srikanth S, Alvarez‐Gallego Y, Vanbroekhoven K, et al. Enzymatic electrosynthesis of formic acid through carbon dioxide reduction in a bioelectrochemical system: effect of immobilization and carbonic anhydrase addition. ChemPhysChem. 2017;18:3174–3181.
- You S, Wang J, Ren N, et al. Sustainable conversion of glucose into hydrogen peroxide in a solid polymer electrolyte microbial fuel cell. ChemSusChem Chem. Sustain. Energy Mater. 2010;3:334–338.
- Kadier A, Kalil MS, Abdeshahian P, et al. Recent advances and emerging challenges in microbial electrolysis cells (MECs) for microbial production of hydrogen and value-added chemicals. Renewable Sustainable Energy Rev. 2016a;61:501–525.
- Ki D, Popat SC, Rittmann BE, et al. H2O2 production in microbial electrochemical cells fed with primary sludge. Environ Sci Technol. 2017;51:6139–6145.
- Cheng D, Ngo HH, Guo W, et al. Performance of microbial fuel cell for treating swine wastewater containing sulfonamide antibiotics. Bioresour Technol. 2020;311:123588.
- Hu K, Xu L, Chen W, et al. Degradation of organics extracted from dewatered sludge by alkaline pretreatment in microbial electrolysis cell. Environ Sci Pollut Res. 2018;25:8715–8724.
- Khan MJ, Singh N, Mishra S, et al. Impact of light on microalgal photosynthetic microbial fuel cells and removal of pollutants by nanoadsorbent biopolymers: updates, challenges and innovations. Chemosphere. 2022;288:132589. Doi.
- Liu X, Ding J, Ren N, et al. The detoxification and degradation of benzothiazole from the wastewater in microbial electrolysis cells. Int J Environ Res Public Health. 2016;13:1259.
- Miran W, Nawaz M, Jang J, et al. Chlorinated phenol treatment and in situ hydrogen peroxide production in a sulfate-reducing bacteria enriched bioelectrochemical system. Water Res. 2017;117:198–206.
- Varjani SJ, Rana DP, Jain AK, et al. Synergistic exsitu biodegradation of crude oil by halotolerant bacterial consortium of indigenous strains isolated from on shore sites of Gujarat, India. Int Biodeterior Biodegrad. 2015;103:116–124.
- Wang A-J, Cui D, Cheng H-Y, et al. A membrane-free, continuously feeding, single chamber up-flow biocatalyzed electrolysis reactor for nitrobenzene reduction. J Hazard Mater. 2012;199-200:401–409.
- Hua T, Li S, Li F, et al. Microbial electrolysis cell as an emerging versatile technology: a review on its potential application, advance and challenge. J Chem Technol Biotechnol. 2019;94:1697–1711.
- Xu X, Shao J, Li M, et al. Reductive Transformation of p-chloronitrobenzene in the upflow anaerobic sludge blanket reactor coupled with microbial electrolysis cell: performance and microbial community. Bioresour Technol. 2016;218:1037–1045.
- Coma M, Puig S, Pous N, et al. Biocatalysed sulphate removal in a BES cathode. Bioresour Technol. 2013;130:218–223.
- Li Y, Zhao Z, Yang Y, et al. Bioelectrochemical enhancement of organic matter mineralization and sulfate reduction during acidogenesis. J Chem Technol Biotechnol. 2018;93:675–682.
- Vinayak V, Khan MJ, Varjani S, et al. Microbial fuel cells for remediation of environmental pollutants and value addition: special focus on coupling diatom microbial fuel cells with photocatalytic and photoelectric fuel cells. J Biotechnol. 2021;338:5–19.
- Dong ZS, Zhao Y, Fan L, et al. Simultaneous sulfide removal and hydrogen production in a microbial electrolysis cell. Int J Electrochem Sci. 2017;12:10553–10566.
- Wang K, Cao Z, Chang J, et al. Promoted bioelectrocatalytic activity of microbial electrolysis cell (MEC) in sulfate removal through the synergy between neutral red and graphite felt. Chem Eng J. 2017;327:183–192.
- Cecconet D, Devecseri M, Callegari A, et al. Effects of process operating conditions on the autotrophic denitrification of nitrate-contaminated groundwater using bioelectrochemical systems. Sci Total Environ. 2018;613-614:663–671.
- Tugaoen HO, Herckes P, Hristovski K, et al. Influence of ultraviolet wavelengths on kinetics and selectivity for N-gases during TiO2 photocatalytic reduction of nitrate. Appl Catal B Environ. 2018;220:597–606.
- Hussain A, Manuel M, Tartakovsky B. A comparison of simultaneous organic carbon and nitrogen removal in microbial fuel cells and microbial electrolysis cells. 2016;J Environ Manage. 173:23–33.
- Kelly PT, He Z. Nutrients removal and recovery in bioelectrochemical systems: a review. Bioresour Technol. 2014;153:351–360.
- Kuntke P, Sleutels T, Arredondo MR, et al. (Bio) electrochemical ammonia recovery: progress and perspectives. Appl Microbiol Biotechnol. 2018;102:3865–3878.
- Zhan G, Zhang L, Li D, et al. Autotrophic nitrogen removal from ammonium at low applied voltage in a single-compartment microbial electrolysis cell. Bioresour Technol. 2012;116:271–277.
- Qin B, Luo H, Liu G, et al. Nickel ion removal from wastewater using the microbial electrolysis cell. Bioresour Technol. 2012;121:458–461.
- Giwa A. Comparative cradle-to-grave life cycle assessment of biogas production from marine algae and cattle manure biorefineries. Bioresour Technol. 2017;244:1470–1479.
- Ghosh P, Sengupta S, Singh L, et al. Life cycle assessment of waste-to-bioenergy processes: a review. Bioreactors. 2020;105–122. doi:10.1016/B978-0-12-821264-6.00008-5.
- Savla N, Pandit S, Verma JP, et al., Techno-economical evaluation and life cycle assessment of microbial electrochemical systems: a review. 2021;Curr. Res. Green Sustain. Chem. 4:100111.
- Fang C, Zhou C, Gu C, et al., A proposal for the theoretical analysis of the interactive coupled effects between urbanization and the eco-environment in mega-urban agglomerations. 2017;J Geogr Sci. 27:1431–1449.
- Liu N, Liu C, Xia Y, et al. Examining the coordination between urbanization and eco-environment using coupling and spatial analyses: a case study in China. Ecol Indic. 2018;93:1163–1175.
- Sheng P, Zhai M, Zhang Y, et al. The effects of urbanization on household wastewater emissions in China: efficient-and inefficient-emissions. Environ Pollut. 2020;267:115350.
- Shindhal T, Rakholiya P, Varjani S, et al. A critical review on advances in the practices and perspectives for the treatment of dye industry wastewater. Bioengineered. 2020;12(1):70–87.
- Li K, Fang L, He L. How population and energy price affect China’s environmental pollution? Energy Policy. 2019;129:386–396.
- Mason K, Duggan J, Howley E. Forecasting energy demand, wind generation and carbon dioxide emissions in Ireland using evolutionary neural networks. Energy. 2018;155:705–720.
- Mohanty SS, Koul Y, Varjani S, et al. A critical review on various feedstocks as sustainable substrates for biosurfactants production: a way towards cleaner production. Microb Cell Fact. 2021;20:1–13.
- Ye Y, Ngo HH, Guo W, et al. A critical review on ammonium recovery from wastewater for sustainable wastewater management. Bioresour Technol. 2018;268:749–758.
- Kirchherr J, Reike D, Hekkert M. Conceptualizing the circular economy: an analysis of 114 definitions. Resour Conserv Recycl. 2017;127:221–232.
- Korhonen J, Honkasalo A, Seppälä J. Circular economy: the concept and its limitations. 2018;Ecol. Econ. 143:37–46.
- Mishra B, Varjani S, Agarwal DC, et al., Engineering biocatalytic material for the remediation of pollutants: a comprehensive review. Env Technol Innov. 20, 101063 ( 2020. 2020;:.
- Prieto-Sandoval V, Jaca C, Ormazabal M. Towards a consensus on the circular economy. J Clean Prod. 2018;179:605–615.
- Linder M. Ripe for disruption: reimagining the role of green chemistry in a circular economy. 2017;Green Chem. Lett. Rev. 10:428–435.
- Kristensen HS, Mosgaard MA. A review of micro level indicators for a circular economy–moving away from the three dimensions of sustainability? J Clean Prod. 2020;243:118531.
- Moraga G, Huysveld S, Mathieux F, et al. Circular economy indicators: what do they measure? Resour Conserv Recycl. 2019;146:452–461.
- Morseletto P. Targets for a circular economy. Resour Conserv Recycl. 2020;153:104553.
- Sharma P, Gaur VK, Gupta S, et al. Trends in mitigation of industrial waste: global health hazards, environmental implications and waste derived economy for environmental sustainability. Sci Total Environ. 2022;811:152357.
- Varjani SJ, Upasani VN. Bioaugmentation of pseudomonas aeruginosa NCIM 5514 - A novel oily waste degrader for treatment of petroleum hydrocarbons. Bioresour Technol. 2021;319:124240.
- Chiranjeevi P, Dahiya S, Kumar N. Waste derived bioeconomy in India: a perspective. N Biotechnol. 2018;40:60–69.
- Iyyappan J, Bharathiraja B, Varjani S, et al. Anaerobic biobutanol production from black strap molasses using Clostridium acetobutylicum MTCC11274: media engineering and kinetic analysis. Bioresour Technol. 2022;346:126405.
- Kostas ET, Adams JMM, Ruiz HA, et al. Macroalgal biorefinery concepts for the circular bioeconomy: a review on biotechnological developments and future perspectives. Renewable Sustainable Energy Rev. 2021;151:111553.
- Katuri KP, Ali M, Saikaly PE. The role of microbial electrolysis cell in urban wastewater treatment: integration options, challenges, and prospects. Curr Opin Biotechnol. 2019;57:101–110.
- Shah AV, Srivastava VK, Mohanty SS, et al. Municipal solid waste as a sustainable resource for energy production: state-of-the-art review. J Environ. Chem. Eng. 9:105717. 2021;https://doi.org/10.1016/j.jece.2021.105717
- Mishra B, Varjani S, Iragavarapu GP, et al. Microbial fingerprinting of potential biodegrading organisms. Curr. Pollut. Rep. 2019; 1–17. DOI:10.1007/s40726-019-00116-5).
- Varjani SJ, Pandey A, Upasani VN. Oilfield waste treatment using novel hydrocarbon utilizing bacterial consortium - A microcosm approach. Sci Total Environ. 2020b;745:141043.
- Rousseau R, Etcheverry L, Roubaud E, et al. Microbial electrolysis cell (MEC): strengths, weaknesses and research needs from electrochemical engineering standpoint. Appl Energy. 2020a;257:113938.