ABSTRACT
We developed a eutectic high-entropy alloy (EHEA) with appreciable high-temperature strength-plasticity balance. Structural characterizations of the as-cast sample revealed a lamellar structure with austenite (face-centered-cubic, FCC) and Laves phases. Upon annealing at 873 K, precipitation of nanoscale (Ni, Al, and Zr)-rich L12 phase from the FCC phase took place, which eventually transformed into a martensitic (hexagonal, ε) phase with rearrangement of atomic species. Compressive properties revealed a brittle behavior at temperatures below 873 K, but changed to a ductile behavior with considerable strain hardening at 973 K, due to the formation of a high density of deformation twins observed by transmission electron microscopy.
GRAPHICAL ABSTRACT
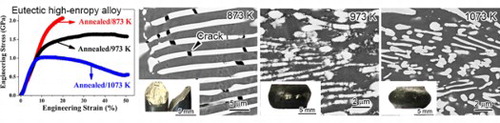
IMPACT STATEMENT
A eutectic high-entropy alloy with attractive high-temperature compressive strength-plasticity balance was realized by tuning the microstructure via heat treatment, during which nanoscale L12 phase formed within the FCC matrix phase.
Eutectic high-entropy alloys (EHEAs), which combine the alloy design concepts of high-entropy alloys and eutectic alloys [Citation1], have received considerable attention since the first successful fabrication of an EHEA by Lu et al. [Citation2,Citation3]. The reported EHEAs were shown to overcome strength-ductility trade-off of both high-entropy alloys (HEAs) and eutectic alloys [Citation4]. The essential design strategy of strong and ductile EHEAs is to grain strength from a hard phase and ductility from a soft phase, where the soft and hard phases being the product of a eutectic reaction [Citation5]. The resulting eutectic structure is expected to provide a good thermal stability and a high-temperature softening resistance [Citation6–8], making the EHEAs promising for high-temperature applications. The selection for the hard and soft phases on EHEAs has to be careful though, to achieve the desirable balance of strength and ductility. For example, the hard phase could be the Laves phase which is known to be brittle in nature at the room to intermediate temperatures [Citation9,Citation10]. EHEAs containing a significant amount of Laves phase showed only marginal compressive plasticity at temperatures below 1073 K [Citation6,Citation11]. Although considerable strength (843 MPa) and compressive plasticity (28%) could be attained at higher temperatures (>1073 K) [Citation11], the EHEAs suffered from softening and limited strain hardening due to the lack of strain hardening sources, which makes them less attractive as structural materials. Hence, developing strong EHEAs with retained plasticity and strain hardening capability at high temperatures is of great interest for many industrial applications [Citation2,Citation11]. Moreover, despite that a brittle-to-ductile transition with increasing deformation temperatures has been reported for many EHEAs [Citation7,Citation8,Citation11], the underneath fracture mechanism has not been studied in detail. This motivated us to investigate the high-temperature deformation behavior of a non-equimolar EHEA with a nominal composition of Co27.6Cr22.6Fe19.6Ni23.4Ta3.4Zr2.5Al0.9 (in at. %). The EHEA was designed by the ‘simple mixture method’ as per the M-Ta (where M = Co, Cr, Fe, and Ni) binary phase diagrams [Citation12]. The detailed compressive behavior at temperatures from 873 to 1273 K was investigated, and post-deformation microstructure were examined.
The EHEA samples were prepared by arc-melting high purity constituent elements (better than 99.99%, wt. %). The ingots were remelted at least three times to improve chemical inhomogeneity and subsequently solidified in a water-cooled copper mold. The resultant ingots were homogenized at 1373 K for 5 h in a vacuum and quenched in ice water. The measured chemical composition from the as-cast ingot using inductively coupled plasma-optical emission spectrometry (ICP-OES) is Co29.0Cr21.6Fe19.5Ni23.3Ta3.5Zr2.4Al0.7 (at. %), which is close to the nominal one. The homogenized samples were annealed at 873 K for 0–100 h and quenched in ice water. For comparison, the as-homogenized sample and as-homogenized sample annealed for 60 h were chosen for the evaluation of mechanical properties and microstructure characterization. Hereafter, the as-homogenized sample and sample annealed for 60 h are denoted as ‘as-homogenized’ and ‘annealed’ samples, respectively. Cylindrical samples with a dimension of 6 mm in diameter and 9 mm in length were compressed using a Gleeble-3800 at 873, 973, 1073, and 1273 K and at a strain rate of 1.0 × 10−3 s−1. All the samples were heated to the compression temperatures at a rate of ∼50 K/s and then kept for 300 s before compression. At least three samples were tested for each condition. Backscattered electron (BSE) scanning electron microscopy (SEM) images were acquired using a Quanta FEG 250 microscope operated at 20 kV. Electron transparent specimens for high-resolution transmission electron microscopy (HRTEM) and scanning TEM (STEM) observations were prepared by the standard twin-jet electro-polishing technique. The HRTEM images and energy dispersive spectroscopy (EDS) maps were acquired using a FEI Titan 80–300 TEM operated at 300 kV. The selected area electron diffraction (SAED) patterns were taken using a Philips CM200 TEM operated at 200 kV. X-ray diffraction experiments (XRD) were conducted using an X-ray diffraction system (X’ Pert PRO MRD) with the Cu Kα radiation.
Figure (a,b) shows normalized XRD patterns of the as-homogenized and annealed bulk samples. A face-centered-cubic (FCC, a = 3.539 Å) phase and a C14-type Laves phase with the MgZn2 prototype [Citation13] can be identified, and no more other phases are seen to exist. The major diffraction peak positions for the Laves phase in the annealed EHEA do not vary significantly, which indicates that annealing does not influence the lattice parameter. It is noted that changes in the peak intensity ratio of the Laves phase are due to crystal texture in the bulk samples and the volume fraction of the Laves phase does not change upon annealing based on SEM observations. While the diffraction pattern in the as-homogenized sample reveals no noticeable superlattice peaks, the annealed sample displays the presence of (100) superlattice reflection, which could be attributed to the formation of the L12 phase. The low magnification and enlarged BSE-SEM images of the as-homogenized and annealed EHEAs are shown in Figure (c–f), respectively. Both samples exhibit a eutectic lamellar structure, and the morphology of the as-homogenized sample has not changed much upon annealing. The lamellar structure with alternating layers is composed of the Laves phase (bright contrast) and the FCC phase (dark contrast) (Figure (c–f)). As shown in a high-angle annular dark-field (HAADF)-STEM and the corresponding elemental STEM-EDS maps (Figure (a)), the Laves phase is enriched with Ta, Co, Zr and the FCC phase contains Fe, Co, Cr, Ni, Ta, and Al. The detailed chemical compositions of the constituent phases in the annealed sample are summarized in Table .
Figure 1. (a,b) X-ray diffraction patterns of the as-homogenized and annealed EHEAs, (c, d) low magnification BSE-SEM images showing eutectic cells and (e, f) are corresponding enlarged views showing a lamellar structure.
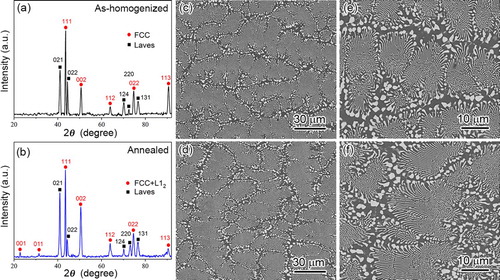
Figure 2. (a) HAADF-STEM and corresponding elemental STEM-EDS maps of Ni, Ta, Fe, Co, Cr, Al and Zr, respectively. (b, c) HRTEM images and SAED patterns (right panel) of the as-homogenized and annealed samples, respectively.
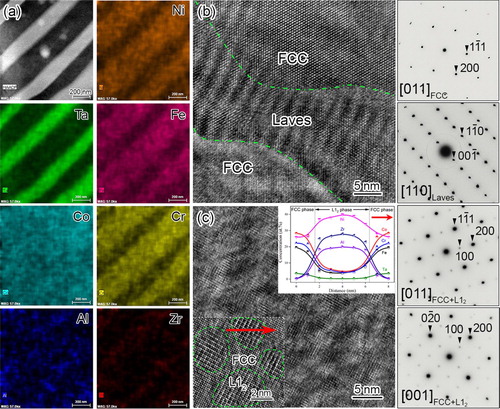
Table 1. Chemical compositions of the constituent phases in the annealed EHEA samples before and after compression at 1073 K.
Figure (b) depicts HRTEM image of the FCC and Laves phases with their SAED patterns shown in the right panel (note the modulation contrast in the Laves phase is Moiré fringes, not additional phases), the observed crystallographic orientation relationship is [011]FCC//[110]Laves and is consistent with previous reports [Citation12,Citation14]. Upon annealing at 873 K for 60 h, ordered L12 domains appear in the FCC matrix phase, as shown in the [001]FCC HRTEM image and SAED patterns (right panel) (Figure (c)), these L12 domains have a typical size of 2–5 nm. Chemical analysis reveals the L12 phase is mainly enriched in Ni, Zr, Al, and has a chemical composition (Table ) close to the stoichiometric Ni3Al compound. In addition, both the matrix and Laves phases contain a similar amount of constituent elements except for Ta, which is 19.8 ± 0.2 at.% for the Laves phase but only 3.5 ± 0.3 at. % for the matrix phase, respectively.
Temporal evolution of the Vickers hardness of the as-homogenized samples upon annealing at 873 K is plotted in Figure (a). The hardness reaches to 642.0 Hv at 60 h and saturates with prolonged annealing, the increased hardness values upon annealing could be attributed to the formation of ordered L12 phase in the matrix FCC phase because the structure, chemistry, and morphology of the Laves have not changed much upon annealing (Figures and ). Figure (b) shows compressive stress-strain curves of the as-homogenized and annealed samples at a temperature of 873–1273 K, respectively. The stress-strain curves obtained at 873 K terminated immediately after yielding for both samples. This supports a brittle nature of the EHEAs deformed at temperatures lower than 873 K. With the compression temperature increases to 973 K, the as-homogenized EHEA undergoes a softening process without any strain hardening. Further increase of compression temperature leads to a more pronounced softening with reduced 0.2 offset yield strength values. In contrast, although the annealed EHEA exhibits a similar brittle deformation mode at 873 K as compared to that of the as-homogenized case, the annealed EHEA compressed at 973 K shows a continuous strain hardening after yielding, which is in sharp contrast to the as-homogenized sample that shows only a strain-softening behavior. At the temperature higher than 973 K, the annealed EHEAs exhibit a similar softening process as compared to those of the as-homogenized samples. However, the annealed sample compressed at 1073 K shows significantly higher yield strength value than that of the as-homogenized one. The summarized 0.2 offset yield strength values vs. compression temperatures for both the as-homogenized and annealed samples are plotted in Figure (d), the annealed EHEA samples show higher 0.2 offset yield strength values compared to those of the as-homogenized ones, and the difference in strength reaches maximum at 1073 K, further increase of compression temperature leads to convergence of the yield strength values. This suggests that the annealed samples are stronger against high-temperature softening than the as-homogenized counterparts at temperatures below 1273 K.
Figure 3. (a) Temporal evolution of the Vickers hardness of the as-homogenized sample annealed at 873 K, (b, c) compressive engineering stress-strain curves of the as-homogenized and annealed EHEAs at 973–1273 K, (d) plot of temperature dependence on 0.2 offset yield strength values.
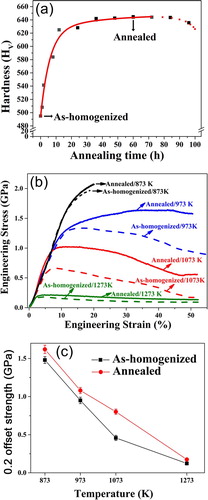
The compression yield strength (σ0.2), peak yield strength (σp) and fracture strain (δ) of the as-homogenized and annealed samples are then compared with previously studied EHEAs [Citation11,Citation15,Citation16] and summarized in Table . Both the as-homogenized and annealed samples show concurrent improvement on both yield strength and fracture strain at 873–1073 K except for the Al20Co20Cr20Fe20Ni20 EHEA that has a lower strength but larger fracture strain compared with other reported EHEAs. This reveals that our EHEA possesses an excellent compressive strength-plasticity balance.
Table 2. Compressive yield strength (σ0.2), peak yield strength (σp) and fracture strain (δ) of the as-homogenized and annealed EHEAs compared with the previously studied EHEAs [Citation11,Citation15,Citation16].
To understand the brittle-to-ductile transition observed for the EHEA samples with increasing compression temperatures, the samples compressed at 873, 973, and 1073 K were chosen for detailed microstructure analysis. For the brittlely deformed sample at 873 K, the fractured surface was carefully polished before SEM observation (Figure (a)); while for the ductilely deformed sample at 973 and 1073 K, the center part of the samples normal to the compression direction was observed (Figure (b,c)). The observation reveals that the morphology of the lamellar structure is retained in the 873 K-compressed sample and cracks within the elongated Laves phase were frequently observed. As shown in an enlarged SEM image in Figure (d), these cracks are found to initiate within the Laves phase other than at phase boundaries. This validates the assumption that brittle deformation of the as-homogenized HEAs at 873 K is due to the intrinsic brittleness of the Laves phase. In contrast, the annealed EHEAs compressed at 973 and 1073 K show a completely different morphology compared to that of the brittlely deformed case (Figure (b,c)), in which the Laves phases are observed to be refined and any noticeable cracks are not observed throughout the samples. Notably, despite similar morphology of the Laves phase is observed in the annealed samples compressed at 973 and 1073 K, the former exhibits finer Laves phase with zig–zag interfacial morphology and the latter with well-defined round shape (Figure (e,f)).
Figure 4. (a, b, c) Low magnification and (d, e, f) enlarged SEM BSE images showing post-deformation microstructures of the annealed EHEA samples compressed at 873, 973, and 1073 K, respectively.
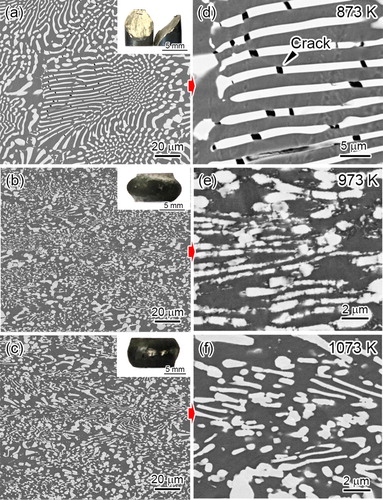
Microstructures of the EHEA samples compressed at 973 and 1073 K are further inspected in order to understand the mechanical behaviors associated with deformation, particularly the observed enhanced strain hardening and increased yield strength value of the as-annealed samples compared to those of the as-homogenized counterparts are of great interest (Figure (b,c)). In the annealed sample compressed at 973 K, a high density of deformation twins are observed by bright-field (BF) TEM observation in combination with electron diffraction experiment (inset) (Figure (a)) and high-resolution HAADF-STEM observation (Figure (b)). However, deformation twins are not observed in the as-homogenized 973 K-compressed sample, in which only dislocations are observed at the phase boundaries between the FCC (inset SAED pattern) and Laves phases (Figure S2(a,b)). Upon increasing the compression temperature to 1073 K, the compressed annealed sample shows a high density of entangled dislocations without any noticeable deformation twins, as is further confirmed by BF-TEM observation as shown in Figure (c). The corresponding SAED pattern (inset) confirms the presence of weakly ordered L12 phase along with diffused streaks running through the 111-type spots and parallel to the <011>FCC directions, implying a possible martensitic phase transformation progresses from the austenite FCC phase. The same area observed using HAADF-STEM revealed a high density of disk-shaped precipitates, which align perfectly well along with the <011> directions of the matrix phase (Figure (d)), these precipitates are observed to pin dislocations strongly at phase interfaces (Figure (c)). This suggests that the high-temperature yield strength of the 1073 K-compressed annealed sample is partly strengthened by the disk-shaped precipitates. An example of the precipitate phase is shown in Figure (e), both the matrix and precipitate phases are confirmed to share a fully coherent interface. The corresponding FFT pattern (inset) of the precipitate phase reveals one pair of diffused streaks, confirming a good match with the SAED pattern in Figure (c). An enlarged view of the precipitate phase reveals the presence of faulted 111-type planes and some of them can be viewed as a mixture of austenite (γ) and martensitic (ε) phases with severe lattice distortions (Figure (f)). Hereafter, this precipitate phase is called ε-phase. In contrast, no visible precipitate phases within the matrix can be observed in the as-homogenized sample compressed at 1073 K (Figure (b,d)), in which only weakly ordered L12 phase is confirmed from a [011] SAED pattern (Figure (e)). Similar to the post-deformation microstructure of the as-homogenized sample compressed at 973 K, the 1073 K-compressed sample exhibits dislocation-pinning at the Laves/FCC phase boundaries. Apart from the structural analyses, the chemical compositions of the precipitate, matrix and Laves phases measured by STEM-EDS (Figures S3 and S4) are summarized in Table . From the table, the disk-shaped precipitate phase appears to have a higher amount of Ta (7.8 ± 0.1 at.%) than that of the L12 phase (0.2 ± 0.1 at.%) in the as-annealed sample.
Figure 5. Post-deformation microstructures of the annealed EHEA samples compressed at 973 and 1073 K. (a, c) Bright-field (BF)-TEM image with inserted SAED patterns; (b, d) HAADF-STEM image showing a deformation twin and precipitates in the annealed samples compressed at 973 and 1073 K, respectively; (e) high resolution HAADF-STEM image showing the structures of the precipitate and matrix phases; (f) enlarged view showing faulted 111-type planes within the precipitate phase.
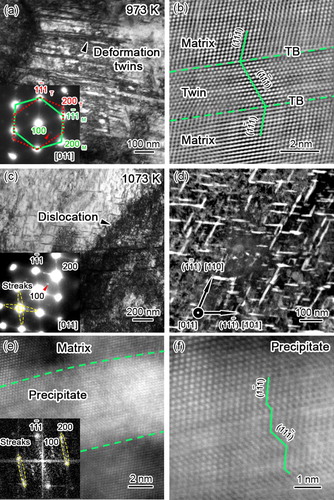
In this work, we have fabricated an EHEA with good high-temperature strength-plasticity balance; the EHEA is composed of the hard Laves and soft FCC phases with the lamellar structure. Upon annealing at 873 K, the Vickers hardness increased with increasing annealing time and saturated at 60 h with a value of 642.0 Hv. Since the structure, chemistry and morphology of the Laves phase have not changed upon annealing (Figures and ), the increased Vickers hardness could be attributed to the precipitation of L12 ordered (Ni, Zr, M)3Al (where M = Co, Cr, Fe, and Ta) phase from the FCC matrix (Figure (c)). The L12 ordering involves with local rearrangement of atomic species due to the strong affinity (or negative mixing enthalpy) between Al and the rest of constituent elements [Citation17,Citation18]. As the L12 ordering nearly completed, saturation of Vickers hardness was observed (Figure (a)). The difference in the degree of L12 ordering between the as-homogenized (Figure (b)) and annealed EHEAs (Figure (c)) contributed to the 0.2 offset yield strength differences of the EHEA samples compressed at 873 K and 973 K because the compression temperatures are close to the annealing temperature (873 K) and the strong chemical L12 ordering was preserved in the annealed sample (Figure (a)) but not in the as-homogenized counterpart (Figure S2(d)). The presence of the ordered L12 nanophase in the FCC matrix is expected to increase local lattice friction stress for dislocation motion, which in turn favors the formation of deformation twins because dislocation and twinning are two competitive modes and both are highly stress dependent [Citation19]. The twin formation also explains the enhanced strain hardening for the annealed sample compared to that of the as-homogenized one, in which L12 phase was not formed after compression (note the time for heating, keeping and compression is less than 300 s and this time is significantly shorter compared with the time required for the formation of L12 phase, which is 36 h at 873 K). The continuous strain hardening via deformation twinning at 973 K is expected to increase local stress concentration at the FCC (L12)/Laves phase interface, which promotes refinement of the Laves phase (Figure (e)).
With compression temperature further increased to 1073 K, precipitation of a high density of weakly ordered Ta-containing ε precipitates took place (Figure (c–f)) due to the enhanced atomic diffusion compared to those of the samples compressed at 873 and 973 K. However, such a high density of Ta-containing ε precipitates was not observed in the as-homogenized sample compressed at 1073 K (Figure S2(b,d)], suggesting that the time for heating and compression, was insufficient to precipitate the Ta-containing ε phase, during which rejection of Al and Zr and gaining of Ta, Co, Cr, and Fe from the L12 phase occurred (Table ). This delayed precipitation in the as-homogenized sample explains the significantly smaller yield strength value compared to that of the annealed sample as shown in Figure (b).
Supplemental Material
Download MS Word (7.8 MB)Acknowledgements
The authors would like to thank Sheng Guo for valuable discussions.
Disclosure statement
No potential conflict of interest was reported by the authors.
ORCID
Xiandong Xu http://orcid.org/0000-0002-7912-4211
Additional information
Funding
References
- Lu YP, Gao XZ, Jiang L, et al. Directly cast bulk eutectic and near-eutectic high entropy alloys with balanced strength and ductility in a wide temperature range. Acta Mater. 2017;124:143–150. doi: 10.1016/j.actamat.2016.11.016
- Lu YP, Dong Y, Guo S, et al. A promising new class of high-temperature alloys: eutectic high-entropy alloys. Sci Rep. 2014;4:6200. doi: 10.1038/srep06200
- Lu YP, Jiang H, Guo S, et al. A new strategy to design eutectic high-entropy alloys using mixing enthalpy. Intermetallics. 2017;91:124–128. doi: 10.1016/j.intermet.2017.09.001
- Jin X, Zhou Y, Zhang L, et al. A novel Fe20Co20Ni41Al19 eutectic high entropy alloy with excellent tensile properties. Mater Lett. 2018;216:144–146. doi: 10.1016/j.matlet.2018.01.017
- He F, Wang ZJ, Cheng P, et al. Designing eutectic high entropy alloys of CoCrFeNiNbx. J Alloy Compds. 2016;656:284–289. doi: 10.1016/j.jallcom.2015.09.153
- He F, Wang ZJ, Shang XL, et al. Stability of lamellar structures in CoCrFeNiNbx eutectic high entropy alloys at elevated temperatures. Mater Des. 2016;104:259–264. doi: 10.1016/j.matdes.2016.05.044
- Huo WY, Zhou H, Fang F, et al. Microstructure and mechanical properties of CoCrFeNiZrx eutectic high-entropy alloys. Mater Des. 2017;134:226–233. doi: 10.1016/j.matdes.2017.08.030
- Zhang YL, Wang X, Li J, et al. Deformation mechanism during high-temperature tensile test in an eutectic high-entropy alloy AlCoCrFeNi2.1. Mater Sci Eng A. 2018;724:148–155. doi: 10.1016/j.msea.2018.03.078
- Guénolé J, Mouhib FZ, Huber L, et al. Basal slip in Laves phases: the synchroshear dislocation. Scr Mater. 2019;166:134–138. doi: 10.1016/j.scriptamat.2019.03.016
- Ding ZY, He QF, Wang Q, et al. Superb strength and high plasticity in laves phase rich eutectic medium-entropy-alloy nanocomposites. Int J Plast. 2018;106:57–72. doi: 10.1016/j.ijplas.2018.03.001
- Jiang H, Qiao DX, Lu YP, et al. Direct solidification of bulk ultrafine-microstructure eutectic high-entropy alloys with outstanding thermal stability. Scr Mater. 2019;165:145–149. doi: 10.1016/j.scriptamat.2019.02.035
- Jiang H, Han KM, Gao XX, et al. A new strategy to design eutectic high-entropy alloys using simple mixture method. Mater Des. 2018;142:101–105. doi: 10.1016/j.matdes.2018.01.025
- Komura Y, Tokunaga K. Structural studies of stacking variants in Mg-base Friauf-Laves phases. Acta Crystallogr Sect B Struct Crystallogr Cryst Chem. 1980;36:1548–1554. doi: 10.1107/S0567740880006565
- Jiang H, Han KM, Qiao DX, et al. Effects of Ta addition on the microstructures and mechanical properties of CoCrFeNi high entropy alloy. Mater Chem Phys. 2018;210:43–48. doi: 10.1016/j.matchemphys.2017.05.056
- Lim KR, Lee KS, Lee JS, et al. Dual-phase high-entropy alloys for high-temperature structural applications. J Alloys Compd. 2017;728:1235–1238. doi: 10.1016/j.jallcom.2017.09.089
- Senkov ON, Wilks GB, Scott JM, et al. Mechanical properties of Nb25Mo25Ta25W25 and V20Nb20Mo20Ta20W20 refractory high entropy alloys. Intermetallics. 2011;19:698–706. doi: 10.1016/j.intermet.2011.01.004
- Duan YH, Huang B, Sun Y, et al. Stability, elastic properties and electronic structures of the stable Zr-Al intermetallic compounds: A first-principles investigation. J Alloy Compds. 2014;590:50–60. doi: 10.1016/j.jallcom.2013.12.079
- Troparevsky MC, Morris JR, Kent PRC, et al. Criteria for predicting the formation of single-phase high-entropy alloys. Phys Rev X. 2015;5:11041.
- Zhu YT, Liao XZ, Wu XL. Deformation twinning in nanocrystalline materials. Prog Mater Sci. 2012;57:1. doi: 10.1016/j.pmatsci.2011.05.001