Abstract
Increasing active sites and improving long-term stability are of great significance for electrocatalysts in water splitting. Herein, we report a simple and metal-economize synthesis of NiFe-LDH@Ni-MOF/NF (Nickle iron layered double hydroxide@Nickle metal-organic frameworks/Nickel foam) heterostructures. The NiFe-LDH@Ni-MOF/NF electrocatalyst possesses ultralow oxygen evolution overpotentials of 205, 231, and 248 mV at current densities of 10, 50, and 100 mA cm–2, respectively, with a small Tafel slope of 43.7 mV dec−1, benefiting from the exposure of edge defects and catalytic active sites, and reduced diffusion pathways for mass and charge transfer, making it one of the best non-noble-metal-based electrocatalysts for oxygen evolution.
GRAPHICAL ABSTRACT
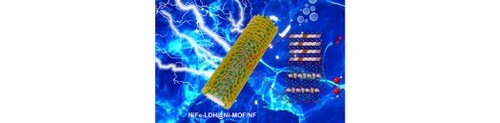
IMPACT STATEMENT
We provide a simple way for synthesizing high-activity and durable LDH/MOF electrocatalysts, without extra metal additions in Ni-MOF in situ synthesis.
1. Introduction
With the increasing global demand for energy and the severe climate change caused by the excessive use of fossil fuels, developing renewable and clean energy is urgently needed [Citation1]. Electrochemical water splitting is an eco-friendly strategy to effectively solve the global energy and environmental crisis [Citation2–4], where the main determinant is the oxygen evolution reaction (OER), because of the sluggish four proton-coupled electron transfer process and high activation barrier [Citation5–8]. Though possess outstanding OER catalytic performance, noble metal-based oxide nanomaterials are difficult for large-scale industrial applications due to high cost [Citation9,Citation10]. Numerous efforts have been made to develop low-cost, high-activity electrocatalysts for OER, such as transition metal oxides, layered double hydroxides (LDHs), and metal-organic frameworks (MOFs) [Citation11–19].
MOFs have received increasing attentions due to ultrahigh specific surface area, homogeneous active metal node distribution [Citation20], adjustable morphology, and structural stability [Citation21, Citation22]. However, the catalytic activities of MOFs are not ideal, due to the low conductivity [Citation23]. Interface engineering may be useful to improve that. Tang et al. reported fine OER performances of stable Ni-MOF in alkaline solutions [Citation24]. Wehrspohn et al. presented the better catalytic activity of NiFe-LDH@NiCoP heterostructures than individual components [Citation13]. Wang et al. constructed NiFe-LDH@Ni3N nano/microsheet arrays to facilitate rapid charge transfer at the interface and obtained enhanced OER activities [Citation25].
In this study, we attempted to utilize the advantages of MOFs to immobilize the LDH and improve OER performance. We designed and successfully synthesized a novel NiFe-LDH@Ni-MOF/NF heterostructured electrocatalyst using a simple two-step preparation process, in situ grown and electrically deposition. The NiFe-LDH@Ni-MOF/NF heterostructure not only inherits the ultra-large surface area from Ni-MOF, but also possesses an excellent phase interface and more exposed active sites. The outstanding OER performances (ultralow overpotentials of 205, 231, and 248 mV at current densities of 10, 50, and 100 mA cm−2, respectively) and stability (potential change of 10 mV after 100 hours testing) were obtained. Our work provides a simple way to synthesize efficient and durable LDH@MOF/NF heterostructured electrocatalysts for oxygen evolution.
2. Materials preparation
The preparation process of the NiFe-LDH@Ni-MOF/NF heterostructure was illustrated as Figure . Different with the conventional synthesis of MOFs, without the extra addition of metal salts, the Ni-MOFs were in situ grown on the NF substrate at a low-temperature of 160°C by a one-step solvothermal method. During the solvothermal process, the Ni2+ ions decomposed from the slow oxidization of NF substrate were immediately coordinated and assembled with the BDC molecules to form MOFs cores, which was playing a role of seed, effectively fixating and accelerating the epitaxial growth of MOF [Citation8, Citation26, Citation27]. Subsequently, after drying, the NiFe-LDH nanosheets were self-dissociation-assembly composited on the Ni-MOFs by electrodeposition. Negatively-charged carboxylate ligands were simultaneously introduced into the NiFe-LDH in form of both coordination and non-coordination, with the NiFe-LDH expected to stabilize the high valence states of the metal centers, and the Ni-MOF expected to serve as proton transfer relays in the second-coordination-sphere of the active sites[Citation28]. The sketches of crystal structure for Ni-MOF and NiFe-LDH@Ni-MOF are shown in Figure as subgraphs.
It is notable that the whole preparation process is simple and environment-friendly (without additional metal salt), making the NiFe-LDH@Ni-MOF/NF heterostructure great potential for practical industrial production. Details of chemicals and preparation procedures were presented in Supplementary Material.
3. Results and discussion
The powder XRD patterns of Ni-MOF/NF, NiFe-LDH/NF, and NiFe-LDH@Ni-MOF/NF are shown as Figure (a). For NiFe-LDH, the strong diffraction peaks pertaining to the (003) and (012) planes can be clearly observed at 11.5° and 34.8°, respectively, consisting with the JCPDS No. 51-0463 [Citation29, Citation30]. The diffraction peaks of Ni-MOF related to the (200), (001), (-201), and (400) planes were observed at 8.9°, 14.2°, 15.9°, and 17.5°, respectively (JCPDS No. 985792, space group of C2/m) [Citation31, Citation32]. The characteristic peaks of both NiFe-LDH and Ni-MOF can be found in the XRD pattern of NiFe-LDH@Ni-MOF.
Figure 2. (a) XRD patterns and (b) Raman spectra of NiFe-LDH/NF, Ni-MOF/NF and NiFe-LDH@Ni-MOF/NF. The interference peaks belong to the NF substrate.
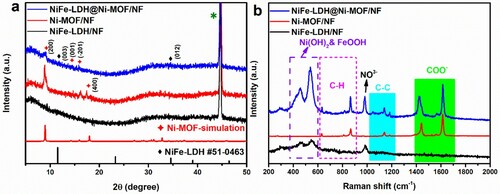
The Raman spectra of NiFe-LDH/NF, Ni-MOF/NF, and NiFe-LDH@Ni-MOF/NF before water oxidation are shown in Figure (b). The Raman spectrum of Ni-MOF/NF was affected by the vibrations of BDC. The peaks at 1612 and 1429 cm−1 can be assigned to COO- groups [Citation28]. The peaks at 1139 and 1183 cm−1 were probably owing to the C–C stretching mode [Citation33]. Three bands around 864, 810, and 629 cm−1 were corresponded to the C–H stretching regions of the benzene ring in Ni-MOF/NF [Citation26]. For NiFe-LDH, the Raman peak at 980 cm−1 was derived from NO3− in the precursor solution. The main peaks located at 455 and 530 cm−1 can be derived from the Ni(OH)2 and FeOOH species, respectively [Citation28,Citation34,Citation35]. The Raman peaks of NiFe-LDH@Ni-MOF/NF were found to contain the peaks of Ni-MOF/NF and NiFe-LDH/NF.
Additionally, FTIR spectra of NiFe-LDH@Ni-MOF/NF and Ni-MOF are shown in Figure S1. The FTIR band around 553 cm−1 was attributed to the Ni-O bonds between the Ni atoms and carboxylic group of terephthalic acid [Citation36]. The band around 655 cm−1 indicated the lattice vibrations of the layer Ni(Fe)-OH in NiFe-LDH [Citation37]. The peaks at 1384 and 1581 cm−1 were attributed to the stretching modes of the symmetric and asymmetric vibrations of the coordinated COO- groups [Citation26, Citation38], respectively. The bending modes of free water molecules and the stretching modes of the hydroxyl groups were also observed at 3400–3600 and 1636 cm−1, respectively [Citation34].
The combined analyses of the XRD, Raman, and FTIR spectra prove the coexistence of NiFe-LDH and Ni-MOF, which indicates successful synthesis of the NiFe-LDH@Ni-MOF/NF heterostructure.
The low and high magnification SEM images of the NiFe-LDH@Ni-MOF/NF are shown as Figure (a–c), the NiFe-LDH@Ni-MOF heterostructures were found uniformly distributed on the NF. Furthermore, the NiFe-LDH nanosheets were also uniformly distributed on Ni-MOF, with the layer thicknesses estimated to be 10–20 nm, proved by the TEM characterization (Figure (d)). Meanwhile, the Ni-MOFs were in the form of interconnected flat and small nanosheets on the NF (Figure S2). Finely resolved lattice fringes with d-spacings of 0.25 and 0.21 nm were observed in the high-resolution TEM (HRTEM) image of NiFe-LDH@Ni-MOF, which were assigned to the (012) and (200) crystal planes of NiFe-LDH and Ni-MOF (Figure (e)), respectively [Citation24, Citation26, Citation34]. The selected area electron diffraction (SAED) pattern (Figure (f)) exhibited three bright rings related to the (018) and (012) crystal planes of NiFe-LDH, and the (200) crystal plane of Ni-MOF. The HAADF-STEM image and elemental mapping (Figure (g) and Figure S3) indicated the uniform distribution of Ni, Fe, C, N, and O elements in NiFe-LDH@Ni-MOF. Similar conclusions could be obtained from the EDX elemental mapping (Figure S4).
Figure 3. SEM image of NiFe-LDH@Ni-MOF/NF at (a) low and (b, c) high magnification. (d) TEM image of NiFe-LDH@Ni-MOF/NF. (e) HRTEM image of NiFe-LDH@Ni-MOF, the insets are the corresponding lattice fringe of the (012) crystal face of NiFe-LDH and the (200) crystal face of Ni-MOF, respectively. (f) SAED pattern of NiFe-LDH@Ni-MOF. (g) HAADF-STEM image and elemental mappings of NiFe-LDH@Ni-MOF.
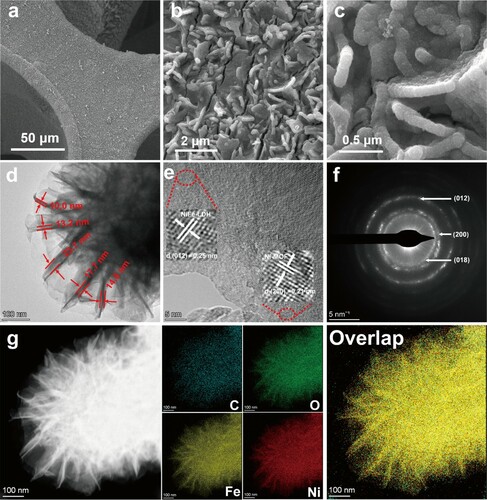
High-resolution X-ray photoelectron spectroscopy (XPS) were carried out to analyze surface chemical compositions and in situ bonding states of the sample. The C 1s spectrum (Figure (a)) can be divided into three bonding states, benzoic rings of the organic ligand linkers at binding energy 284.8 eV (C–C bonds), C–O bonds at binding energy 286.2 eV, and carboxylate groups (O=C–O bonds) of organic ligand linkers at binding energy 288.6 eV [Citation39–41]. The O 1s spectrum (Figure (b)) was fitted by five intense binding energy peaks located at 529.8, 531.0, 531.6, 532.6, and 533.6 eV, attributed to the oxygen atoms of the Ni–O bonds, Ni(Fe)–OH bonds, carboxylate groups of the organic ligands (O=C–O bonds and C–O bonds), and absorbed water [Citation28, Citation42–44]. The XPS spectra of both Fe 2p and Ni 2p for NiFe-LDH@Ni-MOF/NF (Figure (c, d)) present 2p1/2 and 2p3/2 components due to spin-orbit splitting. The Fe 2p spectrum shown in Figure (c), the binding energy peaks located at 712.3 eV and 725.9 eV were attributed to Fe 2p3/2 and Fe 2p1/2 [Citation34, Citation45]. In the high-resolution XPS spectra of Ni 2p (Figure (d)), the binding energy peaks at 855.9 eV (2p3/2) and 873.4 eV (2p1/2) could be ascribed to the Ni–O bonds, while the peaks at 857.4 eV (2p3/2) and 875.1 eV (2p1/2) could be ascribed to the Ni–OH bonds [Citation46–49]. The full XPS spectra of NiFe-LDH@Ni-MOF/NF and Ni-MOF/NF were shown in Figure S5(a). After electrodeposition, the peak of Ni 2p shifted to a lower energy (∼0.5 eV) (Figure S5(b)), indicating the electronic structure changed, due to the introduction of Ni and Fe ions in Ni-MOF. All these results clearly confirmed the successful preparation of NiFe-LDH@Ni-MOF/NF, even after 100 hours stability test, all peaks remained presence (Figure S6).
Figure 4. High-resolution XPS spectra of (a) C 1s, (b) O 1s, (c) Fe 2p (d) Ni 2p for NiFe-LDH@Ni-MOF/NF.
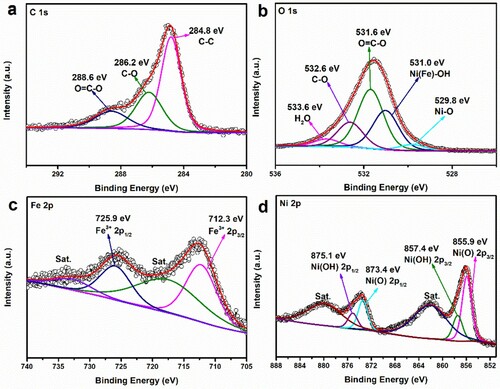
The electrocatalytic OER performances were evaluated in an alkaline solution (1 M KOH) using a standard three-electrode system. The polarization curves and the corresponding Tafel slopes of NiFe-LDH@Ni-MOF/NF, NiFe-LDH/NF, Ni-MOF/NF, NF, and commercial IrO2 are shown in Figure (a–c). Comparing with other above systems, the CV curve of NiFe-LDH@Ni-MOF/NF shows intensified redox peaks between 1.25 and 1.45 V (Figure (a)). It was found the redox peak shifted anodically upon the introduction of Ni and Fe atoms into the Ni-MOF (Figure S10(a)), indicating the emergence of the strong synergistic electronic interaction between Ni and Fe atoms, thereby improving the OER performance [Citation50]. NiFe-LDH@Ni-MOF/NF requires overpotentials (η10, η50, η100) of 205, 231, and 248 mV only, for achieving current densities of 10, 50, and 100 mA cm−2, which are far better than those of NiFe-LDH/NF (η10 = 242 mV, η50 = 271 mV, η100 = 290 mV), Ni-MOF/NF (η10 = 305 mV, η50 =250 mV, i100 = 378 mV), NF (η10 = 424 mV), and commercial IrO2 (η10 = 325 mV, η50 = 426 mV) (Figure (b)). NiFe-LDH@Ni-MOF/NF was found to achieve a current density of 704 mA cm−2 at 1.6 V vs. RHE, which was 30.6 times that of commercial IrO2 (704 vs. 23 mA cm−2). The superior OER performance of the NiFe-LDH@Ni-MOF/NF was further confirmed by extremely low Tafel slope of 43.7 mV dec−1, which was also less than those of NiFe-LDH/NF (65.3 mV dec−1), Ni-MOF/NF (88.2 mV dec−1), NF (151.7 mV dec−1), and commercial IrO2 (97.6 mV dec−1), revealing a more favorable electrocatalytic kinetics for the OER (Figure (c)).
Figure 5. OER performances in 1 M KOH aqueous. (a) Linear sweep voltammetry (LSV) curves of NF, IrO2/NF, Ni-MOF/NF, NiFe-LDH/NF, and NiFe-LDH@Ni-MOF/NF at scan rate 5 mV s−1. (b) Comparison for overpotentials of NF, IrO2/NF, Ni-MOF/NF, NiFe-LDH/NF, and NiFe-LDH@Ni-MOF/NF (c) Corresponding Tafel slopes. (d) Electrochemical double-layer capacitance (Cdl) calculations. (e) Nyquist plots of the different catalyst electrodes, the inset shows enlarged electrochemical impedance spectra (EIS) curves. (f) Chronopotentiometry curves of NiFe-LDH@Ni-MOF/NF at a constant current density of 10 mA cm−2 (without iR compensation).
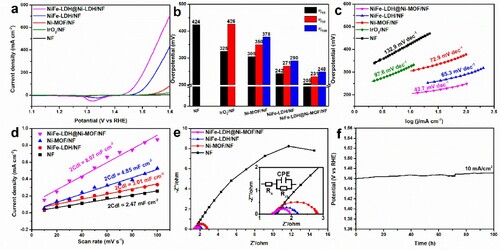
To further study the inherent activity of the NiFe-LDH@Ni-MOF/NF for OER, the electrochemical double-layer capacitance (Cdl) was measured, shown in Figure (d). The NiFe-LDH@Ni-MOF/NF present a higher Cdl value (8.07 mF cm−2) than that of NiFe-LDH/NF (3.01 mF cm−2), Ni-MOF/NF (4.85 mF cm−2), and NF (2.47 mF cm−2). The electrochemically active surface area (ECSA) of NiFe-LDH@Ni-MOF/NF is the largest, because of the linear proportional relationship between Cdl value and ECSA. This observation reveals that the NiFe-LDH@Ni-MOF/NF has inherited the high special surface area from NiFe-LDH and Ni-MOF, and therefore can provide abundant reaction sites for surface reactions, which is one of the factors for remarkably high OER performance. The CV curves measured at different scan rates (10–100 mV s−1) were shown in Figure S7.
In addition, the representative Nyquist plots (Figure (e)) of electrical impedance spectroscopy (EIS) show that the NiFe-LDH@Ni-MOF/NF possesses the smallest charge transfer resistance (Rct) (Table S1), indicating the lowest interfacial barrier for charge and mass transfer kinetics, which is beneficial for OER. The effect of organic ligands concentration was also studied, where 150 mg BDC (denoted as NiFe-LDH@Ni-MOF-150) was the best concentration (Figure S8).
Long-term stability is an important factor in electrocatalysts evaluation. The NiFe-LDH@Ni-MOF/NF electrode was subjected to a series of chronopotentiometry experiments, involving multiple current steps in 1.0 M KOH for 1 h. As the current density increased from 10 to 200 mA cm−2, the corresponding potential required was plotted, shown as Figure S9(a). The overpotential was observed to increase and remained stable, with increasing current density. Furthermore, as shown in Figure (f), the excellent electrochemical stability of NiFe-LDH@Ni-MOF/NF was confirmed by 100 hours stability test at a constant current density of 10 mA cm−2, the potential change was only about 10 mV. After 100 hours of continuous electrolysis, NiFe-LDH@Ni-MOF/NF exhibited only a 3.3% positive shift (7 mV) in the overpotential (Figure S10(b)). As shown in Figure S9(b), the stability of NiFe-LDH@Ni-MOF/NF is better than Ni-MOF/NF and NiFe-LDH/NF. Compared with the recently reported OER electrocatalyst (Figure S10(c) and Table S2), the OER performance of NiFe-LDH@Ni-MOF/NF was highly competitive.
The morphology and composition of the NiFe-LDH@Ni-MOF/NF after 100 hours stability test were analyzed by SEM, Raman, XRD, and XPS, shown as Figures S11–S14. No obvious morphological change was observed for NiFe-LDH@Ni-MOF/NF from SEM images (Figure S11). The main Raman peaks at 475 and 552 cm−1 may be derived from the OER active phase, γ-NiOOH, consisting with the literature [Citation34, Citation35]. The other peaks observed were as the same as those before stability test, but the intensities were reduced (Figure S12). The crystalline phase of NiFe-LDH@Ni-MOF/NF is retained from XRD pattern (Figure S13). The C 1s binding energies were not changed before and after stability test. The signal is attributed to K on the surface of the sample, which may be derived from the residual KOH from the electrolyte (Figure S14(a)) [Citation28]. The O 1s binding energies before and after the stability test were consistent; but the relative content of the metal–hydroxyl species increased after stability tests (Figure S14(b)). As shown in Figure S14(c), the Fe 2p spectrum was consistent with the fresh sample. The binding energies of Ni 2p were the same before and after test, while the relative intensity increased greatly after the stability test (Figure S14(d)). The ratio of the integrated area associated with the Ni-OH/NiO peaks increased from 5:15 to 6:15 after stability test. The analysis of XPS spectra of the sample from NiFe-LDH@Ni-MOF/NF could give an indication that the carboxylate groups can partially de-coordinate from the metal centers of as prepared NiFe-LDH@Ni-MOF/NF.
The excellent OER performance and stability of the NiFe-LDH@Ni-MOF/NF may attribute to the combination of the following factors: (i) the advance of the direct-growth preparation process of NiFe-LDH@Ni-MOF/NF leads to a strong adhesion and ensuring good electrical contact and structural stability; (ii) the hierarchical structure from NiFe-LDH and Ni-MOF leads to abundant pores and a large active surface area, which could promote the exposure of edge defects and catalytic active sites, reduce the diffusion pathways for mass and charge transfer, thereby enhance the catalytic activity [Citation51, Citation52]; (iii) the grafting of NiFe-LDH on Ni-MOF adjusts the electronic structure, leading to the promotion of the synergistic effects between metals. Benefiting from the above-mentioned advantages, the layered NiFe-LDH@Ni-MOF/NF presents satisfactory long-term stability and catalytic activity as an electrocatalyst for water oxidation.
4. Conclusion
In summary, we successfully synthesized the layered NiFe-LDH@Ni-MOF/NF heterostructure electrocatalyst with a facile and simple two-step process. The catalyst possesses a small Tafel slope (43.7 mV dec−1) and low overpotential (η10 = 205 mV), indicating the excellent OER performance, better than those of most reported OER catalysts. In addition, the NiFe-LDH@Ni-MOF/NF has a fairly high durability. The enhanced electrocatalytic performance is likely derived from its hierarchical porosity, large surface area, abundant active sites, intrinsic activity, synergistic tuning of the electronic structure, charge transport characteristics, and the favorable oxidation behavior. We believe this study can provide a simple way for the synthesis of high-activity, and durable LDH/MOF electrocatalysts.
Supplemental Material
Download MS Word (6.8 MB)Disclosure statement
No potential conflict of interest was reported by the author(s).
Additional information
Funding
References
- Coridan RH, Nielander AC, Francis SA, et al. Methods for comparing the performance of energy-conversion systems for use in solar fuels and solar electricity generation. Energy Environ Sci. 2015;8:2886–2901.
- Ma Y-Y, Wu C-X, Feng X-J, et al. Highly efficient hydrogen evolution from seawater by a low-cost and stable CoMoP@C electrocatalyst superior to Pt/C. Energy Environ Sci. 2017;10:788–798.
- Liu J, Zhu D, Guo C, et al. Design strategies toward advanced MOF-derived electrocatalysts for energy-conversion reactions. Adv Energy Mater. 2017;7:1700518.
- Zhao X, Pattengale B, Fan D, et al. Mixed-node metal–organic frameworks as efficient electrocatalysts for oxygen evolution reaction. ACS Energy Lett. 2018;3:2520–2526.
- Garcia AC, Koper MTM. Effect of saturating the electrolyte with oxygen on the activity for the oxygen evolution reaction. ACS Catal. 2018;8:9359–9363.
- Nong HN, Reier T, Oh H-S, et al. A unique oxygen ligand environment facilitates water oxidation in hole-doped IrNiOx core–shell electrocatalysts. Nature Catal. 2018;1:841–851.
- Cai Z, Bu X, Wang P, et al. Recent advances in layered double hydroxide electrocatalysts for the oxygen evolution reaction. J Mater Chem A. 2019;7:5069–5089.
- Huang L, Gao G, Zhang H, et al. Self-dissociation-assembly of ultrathin metal-organic framework nanosheet arrays for efficient oxygen evolution. Nano Energy. 2020;68:104296.
- Muthurasu A, Maruthapandian V, Kim HY. Metal-organic framework derived Co3O4/MoS2 heterostructure for efficient bifunctional electrocatalysts for oxygen evolution reaction and hydrogen evolution reaction. Appl Catal B Environ. 2019;248:202–210.
- Yang G, Li Y, Lin H, et al. Constructing chemical interaction between hematite and carbon nanosheets with single active sites for efficient photo-electrochemical water oxidation. Small Methods. 2020;4:2000577.
- Yang X, Chen J, Chen Y, et al. Novel CO3O4 nanoparticles/nitrogen-doped carbon composites with extraordinary catalytic activity for oxygen evolution reaction (OER). Nano-Micro Lett. 2017;10:15.
- Dinh KN, Zheng P, Dai Z, et al. Ultrathin porous NiFeV ternary layer hydroxide nanosheets as a highly efficient bifunctional electrocatalyst for overall water splitting. Small. 2018;14:1703257.
- Zhang H, Li X, Hähnel A, et al. Bifunctional heterostructure assembly of NiFe LDH nanosheets on NiCoP nanowires for highly efficient and stable overall water splitting. Adv Funct Mater. 2018;28:1706847.
- Dou S, Wang X, Wang S. Rational design of transition metal-based materials for highly efficient electrocatalysis. Small Methods. 2019;3:1800211.
- Zhang M, Liu Y, Liu B, et al. Trimetallic NiCoFe-layered double hydroxides nanosheets efficient for oxygen evolution and highly selective oxidation of biomass-derived 5-hydroxymethylfurfural. ACS Catal. 2020;10:5179–5189.
- Lu X, Xue H, Gong H, et al. 2D layered double hydroxide nanosheets and their derivatives toward efficient oxygen evolution reaction. Nano-Micro Lett. 2020;12:86.
- Kuang M, Zhang J, Liu D, et al. Amorphous/crystalline heterostructured cobalt-vanadium-iron (oxy)hydroxides for highly efficient oxygen evolution reaction. Adv Energy Mater. 2020;10:2002215.
- Ning M, Wu L, Zhang F, et al. One-step spontaneous growth of NiFe layered double hydroxide at room temperature for seawater oxygen evolution. Mater Today Phys. 2021;19:100419.
- Yang L, Liu Z, Zhu S, et al. Ni-based layered double hydroxide catalysts for oxygen evolution reaction. Mater Today Phys. 2021;16:100292.
- Li F-L, Shao Q, Huang X, et al. Nanoscale trimetallic metal–organic frameworks enable efficient oxygen evolution electrocatalysis. Angewandte Chem Int Ed. 2018;57:1888–1892.
- Li L, He J, Wang Y, et al. Metal-organic frameworks: a promising platform for constructing non-noble electrocatalysts for the oxygen-reduction reaction. J Mater Chem A. 2019;7:1964–1988.
- Zhao S, Tan C, He C-T, et al. Structural transformation of highly active metal-organic framework electrocatalysts during the oxygen evolution reaction. Nature Energy. 2020;5:881–890.
- Xie LS, Skorupskii G, Dincă M. Electrically conductive metal–organic frameworks. Chem Rev. 2020;120:8536–8580.
- Zhao S, Wang Y, Dong J, et al. Ultrathin metal-organic framework nanosheets for electrocatalytic oxygen evolution. Nature Energy. 2016;1:16184.
- Wang B, Jiao S, Wang Z, et al. Rational design of NiFe LDH@Ni3N nano/microsheet arrays as a bifunctional electrocatalyst for overall water splitting. J Mater Chem A. 2020;8:17202–17211.
- Sun F, Wang G, Ding Y, et al. NiFe-based metal-organic framework nanosheets directly supported on nickel foam acting as robust electrodes for electrochemical oxygen evolution reaction. Adv Energy Mater. 2018;8:1800584.
- Cao C, Ma D-D, Xu Q, et al. Semisacrificial template growth of self-supporting MOF nanocomposite electrode for efficient electrocatalytic water oxidation. Adv Funct Mater. 2019;29:1807418.
- Li W, Li F, Yang H, et al. A bio-inspired coordination polymer as outstanding water oxidation catalyst via second coordination sphere engineering. Nature Commun. 2019;10:5074.
- Lu Z, Xu W, Zhu W, et al. Three-dimensional NiFe layered double hydroxide film for high-efficiency oxygen evolution reaction. Chem Commun. 2014;50:6479–6482.
- Zhang F-S, Wang J-W, Luo J, et al. Extraction of nickel from NiFe-LDH into Ni2P@NiFe hydroxide as a bifunctional electrocatalyst for efficient overall water splitting. Chem Sci. 2018;9:1375–1384.
- Mesbah A, Rabu P, Sibille R, et al. From hydrated Ni3(OH)2(C8H4O4)2(H2O)4 to anhydrous Ni2(OH)2-(C8H4O4): impact of structural transformations on magnetic properties. Inorg Chem. 2014;53:872–881.
- Kirchon A, Feng L, Drake HF, et al. From fundamentals to applications: a toolbox for robust and multifunctional MOF materials. Chem Soc Rev. 2018;47:8611–8638.
- Liu Q, Xie L, Shi X, et al. High-performance water oxidation electrocatalysis enabled by a Ni-MOF nanosheet array. Inorg Chem Front. 2018;5:1570–1574.
- Cai Z, Bu X, Wang P, et al. Simple and cost effective fabrication of 3D porous core–shell Ni nanochains@NiFe layered double hydroxide nanosheet bifunctional electrocatalysts for overall water splitting. J Mater Chem A. 2019;7:21722–21729.
- Qiu Z, Tai C-W, Niklasson GA, et al. Direct observation of active catalyst surface phases and the effect of dynamic self-optimization in NiFe-layered double hydroxides for alkaline water splitting. Energy Environ Sci. 2019;12:572–581.
- Wu Q, Yang H, Kang L, et al. Fe-based metal-organic frameworks as Fenton-like catalysts for highly efficient degradation of tetracycline hydrochloride over a wide pH range: acceleration of Fe(II)/ Fe(III) cycle under visible light irradiation. Appl Catal B Environ. 2020;263:118282.
- Sanati S, Rezvani Z. Ultrasound-assisted synthesis of NiFe- layered double hydroxides as efficient electrode materials in supercapacitors. Ultrason Sonochem. 2018;48:199–206.
- Li F-L, Wang P, Huang X, et al. Large-scale, bottom-up synthesis of binary metal-organic framework nanosheets for efficient water oxidation. Angewandte Chem Int Ed. 2019;58:7051–7056.
- Cao J, Wang C. Multifunctional surface modification of silk fabric via graphene oxide repeatedly coating and chemical reduction method. Appl Surf Sci. 2017;405:380–388.
- Yan X, Xing Z, Cao Y, et al. In-situ C-N-S-tridoped single crystal black TiO2 nanosheets with exposed {001} facets as efficient visible-light-driven photocatalysts. Appl Catal B Environ. 2017;219:572–579.
- Liang C, Qiu H, Han Y, et al. Superior electromagnetic interference shielding 3D graphene nanoplatelets/reduced graphene oxide foam/epoxy nanocomposites with high thermal conductivity. J Mater Chem C. 2019;7:2725–2733.
- Zhou D, Jia Y, Duan X, et al. Breaking the symmetry: gradient in NiFe layered double hydroxide nanoarrays for efficient oxygen evolution. Nano Energy. 2019;60:661–666.
- Dutta S, Indra A, Feng Y, et al. Promoting electrocatalytic overall water splitting with nanohybrid of transition metal nitride-oxynitride. Appl Catal B Environ. 2019;241:521–527.
- Deng X, Li J, Shan Z, et al. A N, O co-doped hierarchical carbon cathode for high-performance Zn-ion hybrid supercapacitors with enhanced pseudocapacitance. J Mater Chem A. 2020;8:11617–11625.
- Zhang R, Wang G, Wei Z, et al. A Fe-Ni5P4/Fe-Ni2P heterojunction electrocatalyst for highly efficient solar-to-hydrogen generation. J Mater Chem A. 2021;9:1221–1229.
- Xie J, Gao L, Cao S, et al. Copper-incorporated hierarchical wire-on-sheet α-Ni(OH)2 nanoarrays as robust trifunctional catalysts for synergistic hydrogen generation and urea oxidation. J Mater Chem A. 2019;7:13577–13584.
- Karmakar A, Srivastava SK. In situ fabricated nickel vanadate/N-doped reduced graphene oxide hybrid as an advanced electrocatalyst in alkaline hydrogen evolution reaction. J Mater Chem A. 2019;7:15054–15061.
- Thangasamy P, Shanmuganathan S, Subramanian V. A NiCo-MOF nanosheet array based electrocatalyst for the oxygen evolution reaction. Nanoscale Adv. 2020;2:2073–2079.
- Fan R, Zhou J, Xun W, et al. Highly efficient and stable Si photocathode with hierarchical MoS2/Ni3S2 catalyst for solar hydrogen production in alkaline media. Nano Energy. 2020;71:104631.
- Wang Y, Yan L, Dastafkan K, et al. Lattice matching growth of conductive hierarchical porous MOF/LDH heteronanotube arrays for highly efficient water oxidation. Adv Mater. 2021;33:2006351.
- Liu J, Gao Y, Tang X, et al. Metal–organic framework-derived hierarchical ultrathin CoP nanosheets for overall water splitting. J Mater Chem A. 2020;8:19254–19261.
- Saad A, Shen H, Cheng Z, et al. Mesoporous ternary nitrides of earth-abundant metals as oxygen evolution electrocatalyst. Nano-Micro Lett. 2020;12:79.