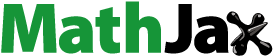
Abstract
The diffusion kinetics of the BCC-structured high-entropy alloys (HEAs) is studied experimentally for the first time. First, the literature data regarding the transition-metal based, single-phase, BCC-structured HEAs is re-evaluated using the state-of-the-art, HEAs-dedicated TCHEA4 thermodynamic database, signalizing the need for further reassessment of the literature data accumulated during the last decade. The selected Al-Cr-Fe-Mn-V system was used for the interdiffusion experiments. The concentration profiles were evaluated using the numerical combinatorial approach, revealing surprisingly fast diffusion kinetics. The comparison with the available diffusion data for conventional BCC alloys supports recent findings regarding the lack of the ‘sluggish diffusion’ in HEAs.
IMPACT STATEMENT
The first-ever experimental study of interdiffusion in BCC-structured high-entropy alloys is conducted, revealing fast diffusion kinetics and providing a further argument against the presence of sluggish diffusion effect in HEAs.
GRAPHICAL ABSTRACT
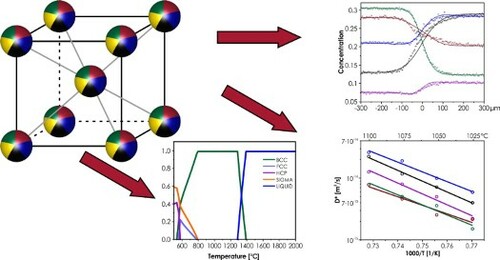
1. Introduction
The high-entropy alloys (HEAs), initially developed back in 2004 [Citation1,Citation2], have become one of the most extensively researched directions of studies in materials science, carrying a promise of obtaining next-generation, structural materials for high-temperature applications. For years believed to possess a number of unique features [Citation3,Citation4], regardless of the actual extent of high-entropy effects [Citation5,Citation6], over the years they have proved to be a viable alternative to state-of-the-art, conventional alloys, with multiple compositions displaying excellent high-temperature corrosion behavior [Citation7–9], good fatigue-resistance [Citation10], complex and tailorable deformation mechanism [Citation11], and most importantly, an excellent thermomechanical behavior [Citation5,Citation12–14]. The common denominator for these latter compositions, especially with regard to transition metal-based HEAs (TM-HEAs), is the presence of a multiphase structure [Citation5,Citation12–16], with body-centered cubic phases (BCC) being of especially crucial importance. However, despite these observations, our understanding of the high-temperature behavior of BCC-structured HEAs, especially in terms of phase stability and diffusion kinetics, remains extremely limited. While the diffusion in FCC-structured HEAs (face-centered cubic) has been relatively thoroughly investigated during last few years [Citation17–27], even allowing for negative verification of the so-called ‘sluggish diffusion effect’ theorem [Citation20,Citation28], which turned out to be associated primarily with the presence of the particular elements in the system e.g. Mn rather than a high number of elements, the diffusion in BCC structure, much more relevant from the application point of view, has never been previously studied on the experimental grounds. The main limiting factor there was likely the fact that most of the early BCC, solid solution-structured HEAs systems had been based mainly on the refractory elements (RF HEAs), characterized by prohibitively high Tamman and melting temperatures [Citation29], rendering the diffusion studies with the use of conventional techniques highly problematic. This has changed with the studies of Senkov et al. [Citation30,Citation31] and Tazuddin et al. [Citation32], who carried out extensive screening procedures with the use of CALPHAD approach (CALculated PHAse Diagrams), revealing a number of stable, non-refractory, BCC-structured HEAs. However, despite their comprehensive nature, both these studies were limited by the quality of early thermodynamic databases, which in turn led to a relatively low level of agreement between theoretical and experimental data [Citation32], bringing questions regarding the overall value of such an approach.
The presented study is intended to address the above-mentioned unknowns, both in terms of our ability to predict the formation of single-phase BCC solid solutions and their transport properties. These objectives are achieved by re-evaluating the single-phase, BCC-structured system suggested by the earlier works [Citation30–32], with the use of the most up-to-date thermodynamic databases, followed by the very first experimental evaluation of the diffusion behavior in body-centered cubic HEAs.
2. Theoretical assessment of phase stability
Firstly, the temperature-dependent phase stabilities of a total of 10 equimolar systems classified by both Senkov et al. [Citation30,Citation31] and Tazuddin et al. [Citation32] as single-phase, BCC ones, were examined with the use of Thermo-Calc software, utilizing the latest HEA-dedicated TCHEA4 database [Citation33]. The results are presented in . As can be seen, only 3 out of 10 evaluated alloys are predicted to be characterized by the single-phase, BCC structure at temperatures exceeding 1000°C, namely AlCrFeMnV, CoCrFeMnV, and AlCrFeMoV, with additional 2 systems exhibiting single-phase, solid-solution structure in a much more limited range: AlCrMnTiV and AlCrNbVW. The comparison with the available experimental data yields nearly perfect agreement, as both AlCrFeMnV and CoCrFeMnV alloys have been proved to form single-phase structures [Citation32] (also in agreement with our results, see Supplementary Figure S1), while the AlCrMnTiV alloy was found by Tazzudin et al. to exhibit the presence of BCC + HCP (hexagonal dense-packed) phases at 900°C [Citation32], almost matching the predictions from c. On the other hand, using the TCHEA4 database, one can arrive at the conclusion that the other five systems, previously predicted in literature to provide single-phase structures, are now expected to form multiphase ones. This shows how drastic progress had been made in terms of the HEAs’ thermodynamic description during the last few years, also indicating the need for a systematic re-evaluation of the previous literature data. In this case, however, the agreement with available experimental data is rather qualitative than quantitative, as for example, the AlMnNbTiV was found in [Citation32] to exhibit multiphase, mainly HCP structure at 900°C, rather than the BCC one predicted in g. Therefore, it can be stated that even with the evident, significant progress in predicting capabilities of the state-of-the-art thermodynamic databases, they still exhibit major deficiencies, which should be addressed in the future.
Figure 1. Temperature vs. phase fraction results calculated by the Thermo-Calc for equimolar alloys: (a) AlCrFeMnV; (b) CoCrFeMnV; (c) AlCrMnTiV; (d) AlFeMnNbV; (e) AlFeMnNbTi; (f) AlFeMnTiV; (g) AlMnNbTiV; (h) AlCrFeMoW; (i) AlCrNbVW; (j) AlCrFeMoV.
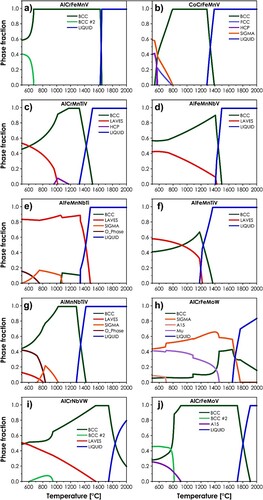
Thanks to by far the most extensive single-phase region, the Al-Cr-Fe-Mn-V system was selected for the experimental investigation of the interdiffusion behavior with the use of diffusion couple method. The design of the diffusion couples end-members was supported by further thermodynamic considerations, with the isopleth-type phase diagrams clearly indicating the possibility of obtaining single-phase materials within a wide compositional range, .
3. Materials and methods
To avoid substantial chemical differences between end-members, and therefore minimize effects connected with the composition dependence of tracer diffusion coefficients values, as well as to keep all materials within the HEAs definition, all elements were assumed to vary within a relatively narrow compositional range of 10–30 at.%: The selected end-members are presented in .
Table 1. Nominal and EDS measured compositions for the alloys used in the experiments. The melting (Tm, solidus) and Tamman (TT) temperatures were calculated with use of Thermo-Calc software.
All studied alloys were obtained from high-purity metals (Alfa Aesar, minimum purity of 99.95% except for V: 99.7%) with the use of the induction melting method, combined with casting into copper molds to form cylindrical ingots of 6 mm diameter. All ingots were remelted 3 times to assure their homogeneity and further subjected to the homogenizing annealing at 1100°C for 48 h, under vacuum conditions, followed by fast removing of the materials from the heating zone to preserve the high-temperature phase structure. Then, from each ingot, a piece was cut and polished to metallographic quality. The prepared samples were investigated using the Scanning electron microscopy (SEM) combined with the Energy-dispersive X-ray spectroscopy (EDS) (ThermoFisher Scientific Phenom XL Desktop SEM equipped with a silicon drift detector). After confirming the single-phase character, the alloys were cut with EDM (Electrical discharge machining method) into 1 mm slices, ground, and polished. The diffusion couples were prepared by assembling together two end-members in the molybdenum holders. For studying the Kirkendall effect, small particles of thorium dioxide were placed on the surface of the initial joint of alloy samples, by using their acetone suspension. Based on the calculated melting and estimated Tamman temperatures (see ), the following experimental temperatures were established: 1025, 1050, 1075, and 1100°C, with the annealing duration of 72, 48, 24, and 24 h, respectively. All experiments were carried out under vacuum conditions (10−6 Tr provided by Pfeiffer Vacuum HiPace® 80 turbomolecular pump), with fast removal of the samples from the heating zone after the experiment’s end. The annealed couples were cut into halves and polished. The prepared samples were analyzed using SEM + EDS method (apparatus: FEI VERSA 3D with EDS analyzer), allowing extracting the concentration profiles.
Firstly, the positions of Matano and Kirkendall planes were assessed, see Table S1. For the exemplary cross-sections of the diffusion couples see Figure S2. However, it turned out that the values of Kirkendall shifts did not exceed 6 µm in all cases, making the examination of interdiffusion with the application of analytical methods, such as [Citation34,Citation35], extremely problematic. Therefore, the numerical approach similar as in [Citation28] was utilized.
The theoretical model used in the analysis of the data obtained from the diffusion couples was based on the Darken formalism [Citation36,Citation37] and its extension by Manning [Citation38–40], which provides a direct connection to Onsager's theory of irreversible thermodynamics [Citation41,Citation42]. The solved set of diffusion equations in the closed, one-dimensional system (zero flux at the boundary) was as follows:
(1)
(1)
where
(2)
(2)
where x− and x+ correspond to the left and right boundary of the system, respectively, r is a total number of elements in the system,
is the volume flux of the i-th element in the laboratory reference frame, Ji is the volume flux of the i-th element in the material's reference frame, ni is the molar fraction of the i-th element,
is the drift velocity, common for all components,
is the tracer diffusion coefficient of i-th element, μi is the chemical potential of the i-th component, Lij are Onsager’s phenomenological coefficients, R is the gas constant, T is temperature, and M0 denotes the geometrical factor in Manning’s theory, equal to 5.33 for BCC structures. The thermodynamics of the system was described within the framework of the regular solid solution model:
(3)
(3)
where
is Gibbs energy of a solid solution phase,
denotes the mixing enthalpy of equimolar binary system i and j, and
is the interaction parameter. The chemical potential can be calculated according to the formula:
(4)
(4)
The interaction parameters values were determined based on the modified Miedema’s scheme [Citation43,Citation44], optimized towards the solid solution structures (Table S2) [Citation45]. The determination of the diffusion coefficients was possible thanks to the numerical procedure, conducted in a ‘global’ manner for each temperature i.e. all profiles from a given temperature were optimized simultaneously using the same set of diffusivities, allowing for averaging of the values and minimizing the error of determination. Further details regarding the diffusion model and numerical procedure can be found elsewhere [Citation28].
4. Results and discussion
The exemplary profiles, both measured and fitted, are presented in . The rest of the data can be found in Supplementary Figures S3–S6. As can be seen, the utilized procedure allows obtaining good quality of the fit, including the directions and magnitude of the up-hill effects, which further supports the correctness of the applied thermodynamic description. The determined values of tracer diffusion coefficients for each considered element and temperature are summarized in .
Figure 3. The exemplary diffusion profiles determined for the Al-Cr-Fe-Mn-V diffusion couples, both experimental and theoretical values are presented: (a) couple A-C, T = 1100°C, t = 24 h; (b) couple A-D, T = 1075°C, t = 24 h; (c) couple B-C, T = 1050°C, t = 46 h; (d) couple B-D, T = 1025°C, t = 72 h.
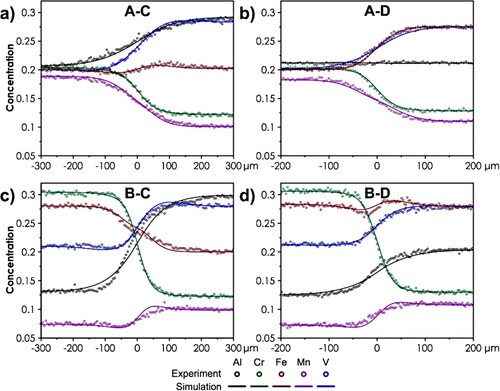
Table 2. The determined values of tracer diffusion coefficients for each experimental temperature. The values of
and Ea are also provided.
In order to check whether the obtained results can be considered unambiguous, and, consequently, if they represent the actual global minimum of the target function, the optimization procedure was carried out for a number of different sets of initial estimates, see Table S3. In all cases the determined values were independent on the algorithm’s starting point, proving the correctness of the utilized approach.
Based on the Arrhenius relation:
(5)
(5)
the pre-exponential factors
and energies of activation Ea were also determined, see . The resulting Arrhenius-type plot is presented in , together with the values of intrinsic diffusion coefficients determined for the respective elements. It is worth noting that the profiles from each temperature were optimized separately, and therefore the fact that the temperature dependence is well preserved, further confirms the physical soundness of the results.
Figure 4. Arrhenius plot for the Al-Cr-Fe-Mn-V system: (a) tracer diffusion coefficients; (b) intrinsic diffusion coefficients. The open points correspond to the values determined for each considered temperature, while the lines represent the linear fits.
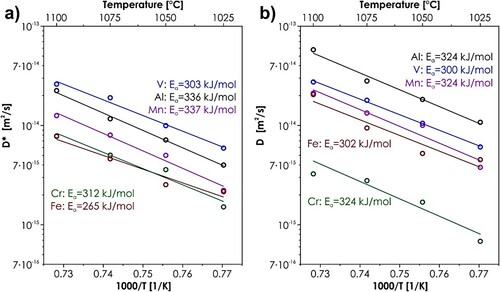
As visible, the kinetics in the Al-Cr-Fe-Mn-V can be considered relatively fast, with the values of tracer diffusivities exceeding 10−14 m2/s at 1100°C, being similar or slightly higher than the values reported for FCC-structured HEAs [Citation19,Citation20,Citation28]. What is especially worth noticing, are the differences between tracer and intrinsic diffusion coefficients, which show how prominent the effects of thermodynamics are in this system. It can be clearly seen that while for the tracer diffusivities vanadium appears to be a faster diffusing element than aluminum, the exact opposite is true for the intrinsic ones. It explains why Al behaves like a faster diffusing species on multiple diffusion profiles in , despite the apparently lower value of the tracer diffusion coefficient. Such behavior can be easily understood, when one compares the values of mixing enthalpies in the typical FCC-structured HEAs system, such as Co-Cr-Fe-Mn-Ni, with the values for Al-Cr-Fe-Mn-V (see Table S4). As visible, the average values of ΔHmix in our system are several times higher than in the reference FCC composition, as a consequence leading to much higher values of thermodynamic factors, introducing much more profound differences when converting between tracer and intrinsic diffusivities.
Considering the history of HEAs development, the question of the biggest importance in terms of diffusion in HEAs, is whether the transport of mass is actually sluggish. What should give a better overview of the actual rate of diffusion, is the comparison among the systems with the temperature scale normalized with respect to the melting point, which is more likely to show actual anomalies in the transport behavior than comparison in the absolute temperature scale [Citation28,Citation46,Citation47]. It should be noted here that between the FCC and BCC structures in HEAs, the latter ones are actually much more likely to exhibit the ‘sluggish diffusion effect’, at least from the viewpoint of the original theory used to support its existence. Such a notion stems from the fact that BCC-structured HEAs are by definition characterized by a much higher atomic size mismatch, described by the δ parameter [Citation48], which in turn should result in a much higher level of lattice distortion, originally believed by Yeh to be hindering the atomic movement in HEAs [Citation3]. Combined with the typical for HEAs, complex and random nature of the nearest-neighbor surroundings of each lattice site, which may lead to the presence of ‘atomic traps’—regions of unfavorable energy landscape [Citation46], it may seem that the BCC-structured HEAs are much closer to the originally envisioned origins of the ‘sluggish diffusion’ [Citation3] than their FCC-structured counterparts. To settle this matter, in the comparison of the Al-Cr-Fe-Mn-V system with the available data for BCC elements and conventional alloys is presented. Additionally, to provide some general idea regarding the rate of mass transport in the studied materials, the values for the FCC-structured Co-Cr-Fe-Mn-Ni reference system are also included [Citation28].
Figure 5. The values of tracer diffusion coefficients of different elements, in different BCC-structured metals and alloys [Citation34,Citation49–53]: (a) Al; (b) Cr; (c) Fe; (d) Mn; (e) V. All plots are presented in the temperature scale normalized with respect to the melting point value Tm of respective materials. The Tamman temperature, corresponding to the 2/3 of the absolute Tm value of is denoted by the vertical gray line. The open circles for the Al-Cr-Fe-Mn-V system correspond to the experimental points determined in this study, while the violet line shows the respective linear fit. Data for Co-Cr-Fe-Mn-Ni, FCC-structured system is also shown for reference [Citation28,Citation34,Citation49–53].
![Figure 5. The values of tracer diffusion coefficients of different elements, in different BCC-structured metals and alloys [Citation34,Citation49–53]: (a) Al; (b) Cr; (c) Fe; (d) Mn; (e) V. All plots are presented in the temperature scale normalized with respect to the melting point value Tm of respective materials. The Tamman temperature, corresponding to the 2/3 of the absolute Tm value of is denoted by the vertical gray line. The open circles for the Al-Cr-Fe-Mn-V system correspond to the experimental points determined in this study, while the violet line shows the respective linear fit. Data for Co-Cr-Fe-Mn-Ni, FCC-structured system is also shown for reference [Citation28,Citation34,Citation49–53].](/cms/asset/0290c336-6862-46ea-a9b9-86764ab56a4d/tmrl_a_2064728_f0005_oc.jpg)
As can be noticed, despite the presence of Mn [Citation28], the diffusion kinetics in the studied system cannot be considered anomalous - for most of the elements it is neither particularly slow nor fast when compared with more conventional systems, and in the case of V, and to a lesser extend Cr, the diffusion kinetics can be even considered to be relatively swift. As a result, it can be stated that despite a number of factors believed to be potentially favoring the occurrence of sluggish diffusion behavior, the Al-Cr-Fe-Mn-V system is yet another evidence of its non-existence in the high-entropy alloys. Of course, a thorough examination of other BCC systems will be necessary before the general conclusions can be drawn, nevertheless, the obtained results strongly support the increasingly popular notion that the diffusion in HEAs is not anomalous in any meaningful way [Citation5,Citation19,Citation20,Citation28].
5. Conclusions
To conclude, the presented study describes the thermodynamic reassessment of the phase stability in BCC-structured TM-HEA systems, and, most importantly, the very first experimental assessment of the diffusion coefficients in such materials, on the example of Al-Cr-Fe-Mn-V system. The thermodynamic analysis documents significant progress in terms of predicting capability between the state-of-the-art TCHEA 4 database and its previous generation predecessors, also signalizing a need for a re-evaluation of the literature data, to fully exploit these improvements. The diffusion kinetics of the selected Al-Cr-Fe-Mn-V system appears to follow the behavior of typical, conventional, BCC-structured systems, showing no sign of diffusion retardation, despite the presence of Mn in the system, and despite the expected higher level of lattice distortion in comparison to high-entropy FCC systems, which in theory should contribute to more sluggish kinetics. As a result, the obtained results strongly support the notion that the ‘sluggish diffusion effect’ does not occur in HEAs and cannot be considered their inherent characteristic.
Supplemental Material
Download PDF (939.6 KB)No potential conflict of interest was reported by the author(s).
Additional information
Funding
References
- Cantor B, Chang IT, Knight P, et al. Microstructural development in equiatomic multicomponent alloys. Mater Sci Eng A-Struct. 2004;375-377:213–218.
- Yeh JW, Chen SK, Lin SJ, et al. Nanostructured high-entropy alloys with multiple principal elements: novel alloy design concepts and outcomes. Adv Eng Mater. 2004;6:299–303.
- Yeh JW. Recent progress in high-entropy alloys. Ann Chim-Sci Mat. 2006;31:633–648.
- Zhang Y, Zuo TT, Tang Z, et al. Microstructures and properties of high-entropy alloys. Prog Mater Sci. 2014;61:1–93.
- Miracle DB, Senkov ON. A critical review of high entropy alloys and related concepts. Acta Mater. 2017;112:448–511.
- Pickering EJ, Jones NG. High-entropy alloys: a critical assessment of their founding principles and future prospects. Int Mater Rev. 2016;61:183–202.
- Butler TM, Alfano JP, Martens RL, et al. High-temperature oxidation behavior of Al-Co-Cr-Ni-(Fe or Si) multicomponent high-entropy alloys. JOM. 2014;16:246–259.
- Butler TM, Weaver ML. Oxidation behavior of arc melted AlCoCrFeNi multi-component high-entropy alloys. J Alloy Compd. 2016;674:229–244.
- Dąbrowa J, Cieślak G, Stygar M, et al. Influence of Cu content on high temperature oxidation behavior of AlCoCrCuxFeNi high entropy alloys (x = 0; 0.5; 1). Intermetallics. 2017;84:52–61.
- Wang Z, Wang C, Zhao Y-L, et al. High hardness and fatigue resistance of CoCrFeMnNi high entropy alloy films with ultrahigh-density nanotwins. Int J Plast. 2020;131:102726.
- Brechtl J, Chen S, Lee C, et al. A review of the serrated-flow phenomenon and its role in the deformation behavior of high-entropy alloys. Metals-Basel. 2020;10(8):1101.
- Daoud HM, Manzoni A, Wanderka N, et al. High-temperature tensile strength of Al10Co25Cr8Fe15Ni6Ti6 compositionally complex alloy (high entropy alloy). JOM. 2015;67:2271–2277.
- Lu Y, Dong Y, Guo S, et al. A promising new class of high-temperature alloys: eutectic high-entropy alloys. Sci Rep. 2014;4:6200.
- Wang Q, Ma Y, Jiang B, et al. A cuboidal B2 nanoprecipitation-enhanced body-centered-cubic alloy Al0.7CoCrFe2Ni with prominent tensile properties. Scr Mater. 2016;120:85–89.
- Manzoni AM, Singh S, Daoud HM, et al. On the path to optimizing the Al-Co-Cr-Cu-Fe-Ni-Ti high entropy alloy family for high temperature applications. Entropy . 2016;18:104.
- Tang Z, Senkov ON, Parish CM, et al. Tensile ductility of an AlCoCrFeNi multi-phase high-entropy alloy through hot isostatic pressing (HIP) and homogenization. Mater Sci Eng A-Struct. 2015;647:229–240.
- Verma V, Tripathi A, Venkateswaran T, et al. First report on entire sets of experimentally determined interdiffusion coefficients in quaternary and quinary high-entropy alloys. J Mater Res. 2020;35(2):162–171.
- Guruvidyathri K, Vaidya M, Murty BS. Challenges in design and development of high entropy alloys: a thermodynamic and kinetic perspective. Scripta Mat. 2020;188:37–43.
- Dąbrowa J, Danielewski M. State-of-the-art diffusion studies in the high entropy alloys. Metals-Basel. 2020;10(3):347.
- Divinski SV, Pokoev A, Esakkiraja N, et al. A mystery of ‘sluggish diffusion’ in high-entropy alloys: the truth or a myth? Diff Fund. 2018;17:69–104.
- Tsai MH, Tsai KY, Tsai CW, et al. Criterion for sigma phase formation in Cr- and V-containing high-entropy alloys. Mater Res Lett. 2013;1:207–212.
- Vaidya M, Pradeep KG, Murty BS, et al. Bulk tracer diffusion in CoCrFeNi and CoCrFeMnNi high entropy alloys. Acta Mater. 2018;146:211–224.
- Gaertner D, Abrahams K, Kottke J, et al. Concentration-dependent atomic mobilities in FCC CoCrFeMnNi high-entropy alloys. Acta Mater. 2019;166:357–370.
- Dąbrowa J, Kucza W, Cieślak G, et al. Interdiffusion in the FCC-structured Al-Co-Cr-Fe-Ni high entropy alloys: experimental studies and numerical simulations. J Alloy Compd. 2016;674:455–462.
- Kucza W, Dąbrowa J, Cieślak G, et al. Studies of “sluggish diffusion” effect in Co-Cr-Fe-Mn-Ni, Co-Cr-Fe-Ni and Co-Fe-Mn-Ni high entropy alloys; determination of tracer diffusivities by combinatorial approach. J Alloy Compd. 2018;731:920–928.
- Verma V, Tripathi A, Kulkarni KN. On interdiffusion in FeNiCoCrMn high entropy alloy. J Phase Equilibria Diffus. 2017;38:445–456.
- Chen W, Zhang L. High-throughput determination of interdiffusion coefficients for Co-Cr-Fe-Mn-Ni high-entropy alloys. J Phase Equilibria Diffus. 2017;38:457–465.
- Dabrowa J, Zajusz M, Kucza W, et al. Demystifying the sluggish diffusion effect in high entropy alloys. J Alloy Compd. 2019;783:193–207.
- Senkov ON, Wilks GB, Miracle DB, et al. Refractory high-entropy alloys. Intermetallics. 2010;18:1758–1765.
- Senkov ON, Miller JD, Miracle DB, et al. Accelerated exploration of multi-principal element alloys with solid solution phases. Nat Commun. 2015;6:6529.
- Senkov ON, Miller JD, Miracle DB, et al. Accelerated exploration of multi-principal element alloys for structural applications. Calphad. 2015;50:32–48.
- Tazuddin GN, Biswas K. In the quest of single phase multi-component multiprincipal high entropy alloys. J Alloy Compd. 2017;697:434–442.
- Thermo-Calc Software TCHEA: High Entropy Alloys database version 4.1. June 2020.
- Mehrer H, editor. Diffusion in solid metals and alloys. Berlin: Landolt–Börnstein; 1990.
- Zajusz M, Dąbrowa J, Danielewski M. Determination of the intrinsic diffusivities from the diffusion couple experiment in multicomponent systems. Scr Mater. 2017;138:48–51.
- Darken LS. Diffusion, mobility and their interrelation through free energy in binary systems. Trans Am Inst Min Metall Eng. 1948;175:184–201.
- Holly K, Danielewski M. Interdiffusion and free-boundary problem for R-component (≥2) one-dimensional mixtures showing constant concentration. Phys Rev B. 1994;50:13336–13346.
- Manning JR. Diffusion and the kirkendal shift in binary alloys. Acta Metall Sin. 1967;15:817–826.
- Manning JR. Cross terms in the thermodynamic diffusion equations for multicomponent alloys. Metall Trans. 1970;1:499–505.
- Manning JR. Correlation factors for diffusion in nondilute alloys. Phys Rev B. 1971;4:1111–1121.
- Onsager L. Reciprocal relations in irreversible processes I. Phys Rev. 1931;37:405–426.
- Onsager L. Reciprocal realtions in irreversible processes II. Phys Rev. 1931;38:2265–2279.
- Miedema AR. A simple model for alloys II. The influence of ionicity on the stability and other physical properties of alloys. Philips Techn Rev. 1973;33:196–202.
- Miedema AR. A simple model for alloys I. Rules for the alloying behaviour of transition metals. Philips Techn Rev. 1973;33:149–160.
- Zhang RF, Zhang SH, He ZJ, et al. Miedema calculator: a thermodynamic platform for predicting formation enthalpies of alloys within framework of Miedema’s theory. Comput Phys Commun. 2016;209:58–69.
- Tsai KY, Tsai MH, Yeh JW. Sluggish diffusion in Co–Cr–Fe–Mn–Ni high-entropy alloys. Acta Mater. 2013;61:4887–4897.
- Vaidya M, Trubel S, Murty BS, et al. Ni tracer diffusion in CoCrFeNi and CoCrFeMnNi high entropy alloys. J Alloy Compd. 2016;688:994–1001.
- Zhang Y, Zhou YJ, Lin JP, et al. Solid-solution phase formation rules for multi-component alloys. Adv Eng Mater. 2008;10:534–538.
- Lindwall G, Frisk K. Assessment and evaluation of mobilities for diffusion in the bcc Cr-V-Fe system. J Phase Equilib Diff. 2009;30(4):323–333.
- Kunitake T. The diffusion in the iron-chromium alloy. J Jpn I Met Mater. 1961;25(11):698–702.
- Yi C, Cheng L, Yunlong Z, editors. Assessment of atomic mobility for the Bcc phase of the Ti-Al-Mo system. IOP Conf. Ser.: Mater. Sci. Eng.; 2017; Changsha (China).
- Wu Q, Xu G, Chang H, et al. Assessment of diffusion mobility for bcc phase of Ti–Al–Ni ternary system. Calphad. 2020;71:102203.
- Rohrberg D, Spitzer K-H, Dörrer L, et al. Host atom diffusion in ternary Fe-Cr-Al alloys. Metal Mater Trans A. 2014;45(1):269–279.