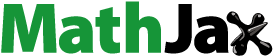
ABSTRACT
Texture adjusting approaches of divergence, inclination, and deviation were proposed to improve the tension-compression asymmetry in magnesium alloys. The dominant deformation mechanism during tensile and compressive yielding was quantified based on the Sachs assumption and primarily manifested as the extent of twinning inhibition and the transition between twinning and slip with the variation of texture adjustment. Its impacts on associated yield strength and asymmetry were estimated by the modified Hall-Petch relationship. Slight texture adjustments, particularly of basal texture divergence, can significantly improve the strength asymmetry while avoiding severe strength loss, illustrating the significance and potential of texture adjustment in engineering.
GRAPHICAL ABSTRACT
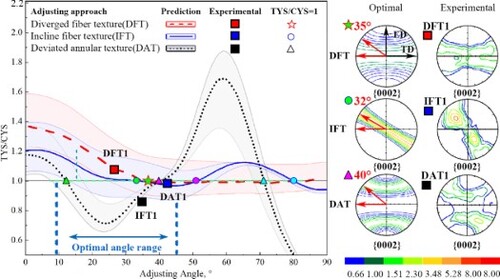
IMPACT STATEMENT
Yield strength and the contribution of deformation mechanisms were predicted using the modified Hall-Petch relationship, and texture adjustment strategies were further established to improve tension-compression asymmetry.
1. Introduction
Wrought magnesium alloys are important lightweight structural materials with low density and high specific strength [Citation1,Citation2]. However, magnesium alloys can easily form a strong basal fiber texture after extrusion. This results in a significant directional difference in its mechanical properties, especially in tensile and compressive yield strength asymmetry. This strength asymmetry undoubtedly brings hidden dangers to the design and application of the bearing structure of magnesium alloys [Citation3–5]. The root cause is that the dominant plastic deformation mechanisms of strong basal textured magnesium alloys under different loads are quite different, specifically, multi-slip yielding in tension and tensile twinning in compression [Citation6,Citation7].
The orientation of the basal plane most obviously determines the activation of the tensile-compressive-dominated plastic deformation mechanism, especially the activation of deformation twinning. In turn, this affects the strength and strength asymmetry associated with the basal texture, which has been discussed and studied by many researchers [Citation8–12]. The adjustment of the basal texture based on the plastic deformation path has been proven to be an effective way to improve the strength asymmetry. The typical adjusting approaches include: the divergence of the basal texture realized by forging-extrusion [Citation13–15]; the inclination of the basal texture obtained by equal channel extrusion and asymmetric extrusion [Citation16–19]; and the deviation of the basal texture achieved by cyclic extrusion and compression [Citation20–22]. Accordingly, the yield strength and its strength asymmetry are highly texture-dependent and can be significantly improved by grain refinement. This texture dependence is also reflected in the change in the grain sensitivity of the strength, known as the slope of the Hall-Petch relationship:
(here,
is the yield strength,
is the friction stress for dislocation gliding, and
is the average grain size), which varies in a wide range from 150 to 450 MPa·μm1/2 depending on the dominant deformation mechanism [Citation23–27]. Therefore, it is rather necessary to establish effective texture adjustment strategy for high load-bearing performance of wrought magnesium alloys.
The quantification of texture effects, typically characterized as the orientation factor , is crucial to revealing the texture dependence of strength. At present, the estimation of
for strongly textured polycrystals is mostly limited to the equivalent single crystal assumption. For example, Cáceres et al. [Citation28] estimated that the
of pure magnesium with a strong basal texture was between 2.1 and 2.5 by comparing the hardening behavior of polycrystals and equivalent single crystal. However, the more common approach is based on the Sachs assumption of monophyletic slip/twinning due to the large differences between the slip/twinning mechanisms of magnesium alloys. The
was estimated by the reciprocal of the Schmid factor m of a preferentially activated slip/twin system and showed better experimental agreement [Citation20,Citation29,Citation30]. In particular, Choo et al. effectively estimated the
and associated
values for different activated deformation mechanisms of magnesium plates with strong basal texture [Citation30]. In order to apply to the texture adjustment of magnesium alloy polycrystals, especially the weakening of the basal texture, we introduced an equivalent Schmid factor using the statistical average method and constructed a four-parameter Hall-Petch relationship. The texture dependence of the yield strength of polycrystals is well explained and predicted in upsetting-extruded ZK61 and multi-forged AZ31 [Citation31–33].
Based on the above mode, taking the divergence, inclination, and deviation of the basal texture as the adjusting approaches, we quantified the effect of the basal texture state on the dominant deformation mechanism of tensile and compressive yielding in wrought magnesium alloys and analyzed its influence on the yield strength and related tension-compression asymmetry, to eventually establish texture adjusting strategy for excellent load-bearing properties in wrought magnesium alloys.
2. Experimental details
The initial material was as-extruded Mg-2.9wt%Al-0.9wt%Zn (AZ31B) alloy rods with an ideal extruded fiber texture. Secondary extrusion at 473 K and further annealing treatments at 573 K with varying periods (O-temper) were carried out to produce varying grain sizes ranging from 5.5 μm to 30 μm (a total of 12 samples with different grain sizes). Three combined extrusion techniques-upsetting and extrusion with extrusion ratio of 4–10 [Citation32,Citation34], equal channel angular extrusion with internal channel angle of 90°–150° [Citation35], and cyclic extrusion and compression with extrusion ratio of 2–4 and cyclic passes of 2–8 [Citation20,Citation36] were employed to prepare magnesium alloy rods with diverged fiber texture (DFT), inclined fiber texture (IFT), and deviated annular texture (DAT), respectively. All the combined extrusion samples underwent extrusion at a temperature of 573 K and a velocity of 5 mm/s.
Uniaxial tension and compression tests were performed on an Instron 5967 testing machine with a starting equivalent strain rate of 6.7 × 10−4 s−1. Tensile specimens with a gauge size of Φ4 mm × 15 mm and compressive specimens of Φ8 mm × 12 mm were cut from the rods along the extrusion direction (ED).0.2% offset yield strength was determined by the offset method described in ASTM standard E8M-16a and was repeated three times in the same condition. Microstructure, including texture, was examined by electron backscattering diffraction (EBSD) using a Quanta 200FEG field emission scanning electron microscope. The size of each grain and its average value were determined through the area-based measuring method using TSL OIM Analysis software.
3. Results and discussion
Figure shows the tensile and compressive yield strengths of magnesium alloy rods with 0002 fiber texture prepared by secondary extrusion as a function of grain size along the extrusion direction. It is clear that both the yield strengths strictly showed linear Hall-Petch relationships within the scope of this study and presented an obvious strength difference with the tensile/compressive yield strength ratio (TYS/CYS) changing from 1.23 at 30 μm to 1.04 at 5.5 μm. The tensile yielding was dominated by slip with a friction stress of 119 MPa and a
value of 196 MPa μm−1/2. In contrast, compressive yielding was dominated by 10–12 twinning, which had a lower friction stress of 70 MPa and a rather higher
value of 291 MPa·μm−1/2.
Figure 1. Tensile/compressive yield strengths of magnesium alloy rods with 0002 fiber texture as a function of grain size d−1/2 along the ED.
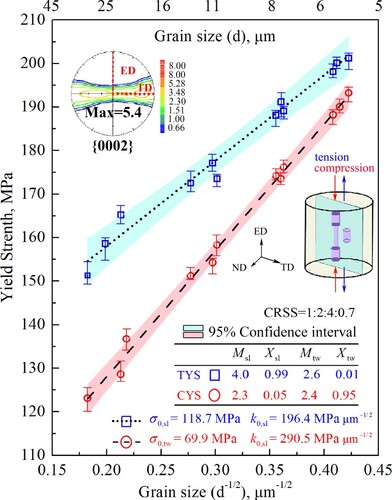
To deeply understand the strengthening mechanism behind the distinctly different Hall-Petch relationships of textured magnesium alloy polycrytals, a four-parameter equation to predict the yield strength considering four deformation modes: basal<a> slip, prismatic<a> slip, pyramidal<c+a> slip, and 10–12 twinning, was proposed referring to the Sachs model by Barnett [Citation37]. The CRSS (critical resolved shear stress) ratios of the four deformation modes: were set to 1:2:4:0.7 for the AZ31 magnesium alloy [Citation30]. The Sachs assumption, as Hosford suggested [Citation38], highlights that only one system of slip or twinning was considered to activate in each grain, particulary at yielding. The preferentially activated system in a grain is the one with the lowest CRSS/m among the four modes for uniaxial tension or compression [Citation37]. Thus, two fractions were set for the equation:
, the fraction of grains where yielded only by slip, and
, grains undergoing twinning. Based on the division, two corresponding yield stresses,
and
, were calculated following the Hall-Petch relation, respectivley.
For grains undergoing slip, the slope K can be calculated by the formula proprosed by Armstrong et al. [Citation39,Citation40] according to the dislocation pile-ups model:
(1)
(1) where M is the orientation factor reflecting texture effect,
equal to
is the CRSS for given slip mode, and r is the distance between the dislocation pile-up and the nearest dislocation sources in an adjacent grain. Thus, the Hall-Petch relationship for slips can be expressed as [Citation41–43]:
(2)
(2) where
is the square root of r and was assumed to be constant in the present, and the superscript sl was added for identification.
Further, to deal with the textured polycrystals and the different CRSSes of different slip modes, the orientation factor was equivalent calculated via a weighted average method based on quantitative statistical results as follows:
(3)
(3) where g is the grain undergoing slip, s is the slip mode,
is the CRSS of the slip mode s,
is the Schmid factor of the preferentially activated slip mode s in the grain g, wg is the corresponding area-weighted percent of the grain g, and
is the reference CRSS for equivalence, which is usually that of the basal slip
.
Different from the hardening induced by dislocation pile-ups for slip, the twinning nucleation and expansion represent the stress relaxation. This behavior tends to make the yielding mainly determined by the Schmid factor of the twinning system [Citation24,Citation44], and shows a higher K of the grain size strengthening (about 1.5∼7.0 times that of slip). Thus, for grains undergoing twinning, the slope K can be estimated as:
(4)
(4) where ktw can be treated as a constant value referring to the research of Wang et al. [Citation30], the superscript ‘tw’ was added for identification. Thus, the yield strength dominated by twinning can be directly expressed as:
(5)
(5) where
is the associated orientation factors for twinning (following the same formula as
and taking the CRSS of twinning
as a reference).
Combining the stresses generated by the two volumes at yielding, the yield strength could be predicted as:
(6)
(6) The fitting parameters of the Equation (6) for the present yield strengths were obtained as shown in Table .
Table 1. Values fitting Equation (6) for the four-parameter Hall-Petch equation.
Three typical texture states, DFT-II, IFT-III, and DAT-II, were selected to analyze the significant influence of texture types on the deformation mechanism at yielding. The corresponding proportions of preferentially activated deformation mechanisms in the polycrystals based on the Sachs assumption are shown in Figure c. These three texture adjusting approaches all clearly show effective improvement in tensile and compressive yield strength asymmetry arising from strong fiber texture and have significant differences in improvement mechanisms. (i) DFT essentially did not change the slip mechanism’s dominance in tension, and the improvement in yield strength asymmetry was attributed to an increase in slip-activated proportion in compression, resulting in a higher CYS of about 158 MPa and a better TYS/CYS of 1.11. (ii) IFT decreased slip activation in tension while increasing it in compression, resulting in the most significant improvement in TYS/CYS (around 0.98) at the expense of tensile yield strength. (iii) The effect of DAT was similar to that of IFT. The difference is that the tensile and compression yield strengths were reversed due to the overall annular deviation of the basal plane, characterized by a TYS/CYS of 0.87.
Figure 2. (a) Experimental and predicted tensile/compressive yield strengths using three texture adjusting approaches (DFT, IFT, and DAT); (b) 0002 pole figures using three texture adjusting approaches (DFT, IFT, and DAT); (c) proportions of preferentially activated deformation mechanisms corresponding to the representative textures of (b, DFT-II, IFT-III, DAT-II) estimated using the Sachs assumption.
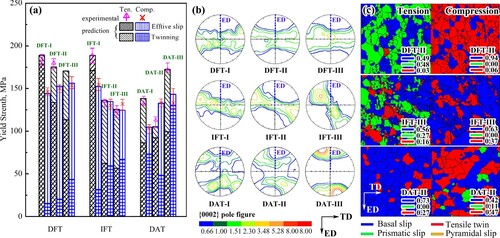
In order to further reveal the effect of texture adjustment on the deformation mechanism of magnesium alloys, referring to the textures in Figure b, we constructed three basal texture adjusting approaches with the variation of adjusting angles from 0° to 90° (Figure a–c). The quantified proportions of and
, and the orientation factors
and
were illustrated as a function of the angle (Figure d–f for tension, h-i for compression). The adjusting approach and angle have an important influence on the deformation mechanism in tensile and compressive yielding. Regardless of the adjusting approach, it is beneficial to reduce the significantly high slip
and the
of the initial fiber texture in tensile yielding, and it is more effective to suppress the twinning
in compression yielding. The extent of the influence of adjusting angle on the deformation mechanism follows: DFT < IFT < DAT. An important feature is the transition in the dominant deformation mechanism. Specifically, DFT maintained the dominant deformation mechanism during yielding similar to the initial, which is exhibited by slip in tension and twinning in compression. In contrast, the dominant deformation mechanism of IFT during compressive yielding transformed from twinning to slip after the adjusting angle of 45°. More dramatically, DAT exhibited an earlier twinning-to-slip transition at 22° during compression yielding, and even a reverse slip-to-twinning transition at 55° during tensile yielding.
Based on the obtained deformation mechanism under the influence of texture in Figure , the variations of TYS and CYS with adjusting angle were calculated using the four-parameter Hall-Petch relationship as illustrated in Figure . The color-shaded area in the figure reflected the grain size sensitivity affected by texture. The texture effect on the deformation mechanism was directly reflected in the changes of TYS and CYS. At the lower angles less than 30°, the TYS decreased significantly with the decrease of slip-activated and
under all the three texture adjusting approaches, while the CYS slightly increased or remained stable depending on the extent of twinning inhibition. The grain size sensitivity on the strength at this stage was also the most significant. With the further increase of the adjusting angle, the transition of the dominant deformation mechanism associated with the texture adjustment caused significant differences in the yield strength change, and the extent of the strength change also follows DFT < IFT < DAT. A maximum CYS of 160 MPa at an angle of 30° was achieved in DFT, which is obviously related to the high compressive
, and then both TYS and CYS showed a monotonous but gentle decrease as the angle increased. The induced strength change was merely within 20 MPa. While in IFT, the trends of TYS and CYS showed a concave shape, which first decreased rapidly and then slowly recovered with the increase in adjusting angle, and the angle of minimum yield strength was around 45° in tension and 65° in compression. This strength trend is directly related to the change of the dominant deformation mechanism, particularly the proportions of the basal slip and twinning (Figure e and h). As expected, the abrupt transitions in the dominant deformation mechanism in DAT caused a more pronounced concave changes in TYS and CYS. The minimum was only about 80 MPa for both yield strengths at about 45°, and was extremely insensitive to grain size. Another notable difference from other textures is that TYS and CYS achieved twinning-dominated local maxima at 63° and 18° due to high
, respectively. Apparently, basal texture adjustment mostly had a negative impact on the enhancement of yield strength in both tension and compression, which was attributed to either the weakening of low-
twinning or the substantial activation of low-
slip. Therefore, slight adjustment of basal texture is most favorable for achieving high yield strength in both tension and compression.
Figure 3. Adjusted basal textures constructed by three adjusting approaches with varying adjusting angle from 0° to 90°: (a) DFT, (b) IFT, and (c) DAT; The corresponding proportions X and orientation factors M of the deformation mechanism for tensile yielding (d-f) and compressive yielding (h-i). Note that IFT and DAT were constructed based on DFT with an adjusting angle of 10° to be consistent with the experimental.
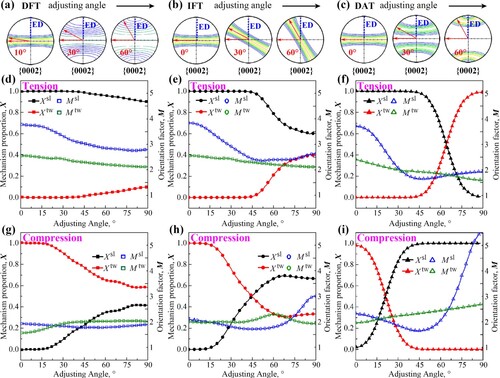
Figure 4. Variation of TYS and CYS with adjusting angle calculated using the four-parameter Hall-Petch relationship: (a) DFT, (b) IFT, and (c) DAT. A fixed grain size of 10 μm was chosen for comparison, and a grain size range of 5–45 μm was used to reflect grain size sensitivity, which was colored in blue for TYS and in red for CYS.
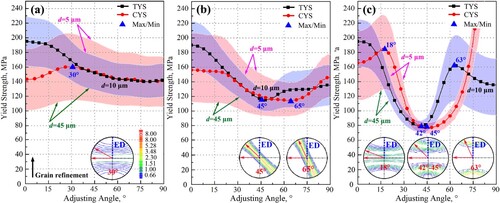
The TYS/CYS with variation of the adjusting angle was employed to investigate the texture influence on the improvement of the tension-compression asymmetry, as shown in Figure . The influence of texture adjustment on tensile and compressive yield strength is also largely reflected in the change of tension-compression asymmetry. The strength asymmetry can be greatly improved with appropriate texture adjustment while minimizing strength loss. For DFT, IFT, and DAT, the recommended adjusting angles are roughly 30°, 20°, and 15°, respectively. The higher grain size sensitivity of the adjacent angle region also means that it can be more adequately improved with the aid of grain refinement. In the subsequent angle regions, the tension-compression asymmetry of the three texture adjusting approaches showed variations in TYS/CYS corresponding to the transitions of the dominant deformation mechanism. Specifically, DFT exhibited a nearly steady TYS/CYS of about 1.0 with noticeable grain size sensitivity; IFT displayed moderate fluctuations in TYS/CYS of 0.9–1.2; and DAT presented severe fluctuations in TYS/CYS of 0.5–1.8 and multiple reversals. Fluctuations in the latter two were less sensitive to grain size.
4. Conclusions
In summary, basal texture adjustment has non-negligible impacts on tensile and compressive yield strengths as well as its asymmetry of wrought magnesium alloys, demonstrating texture adjustment’s potential and importance in engineering. The key findings drawn from this study are:
Slight texture adjustment in all three texture approaches can significantly improve the strength asymmetry while avoiding severe strength loss.
Variations in strength and its asymmetry with adjusting angle were closely related to texture effects on the deformation mechanism based on the Sachs assumption, which were mainly manifested in the extent of twinning inhibition in the low adjusting angle region and the transformation of the dominant deformation mechanism in the subsequent angle region.
The divergence of the basal texture exhibits the least strength loss and great grain size sensitivity, the deviation of the basal texture exhibits the most effective impact on tension-compression asymmetry but the most serious strength loss, and the inclination of the basal texture falls in the middle.
Declaration of competing interest
The authors declare that they have no known competing financial interests or personal relationships that could have appeared to influence the work reported in this paper.
Disclosure statement
No potential conflict of interest was reported by the author(s).
Additional information
Funding
References
- Song J, Chen J, Xiong X, et al. Research advances of magnesium and magnesium alloys worldwide in 2021. J Magnes Alloy. 2022;10(4):863–898.
- Zeng Z, Salehi M, Kopp A, et al. Recent progress and perspectives in additive manufacturing of magnesium alloys. J Magnes Alloy. 2022;10(6):1511–1541.
- Shi B, Yang C, Peng Y, et al. Anisotropy of wrought magnesium alloys: a focused overview. J Magnes Alloy. 2022;10(6):1476–1510.
- Agnew SR, Nie JF. Preface to the viewpoint set on: the current state of magnesium alloy science and technology. Scripta Mater. 2010;63(7):671–673.
- Stanford N, Sotoudeh K, Bate PS. Deformation mechanisms and plastic anisotropy in magnesium alloy AZ31. Acta Mater. 2011;59(12):4866–4874.
- Kweon S, Raja DS. Investigation of the mechanical response of single crystal magnesium considering slip and twin. Int J Plasticity. 2019;112:1–17.
- Park SH, Lee JH, Moon BG, et al. Tension–compression yield asymmetry in as-cast magnesium alloy. J Alloys Compd. 2014;617:277–280.
- Kim B, Baek S-M, Jeong HY, et al. Grain refinement and reduced yield asymmetry of extruded Mg–5Sn–1Zn alloy by Al addition. J Alloys Compd. 2016;660:304–309.
- Wei J, You J, Zhang D, et al. Reducing yield asymmetry in a wrought Mg–9Al alloy by randomized texture achieved via multi-directional forging. Mater Sci Eng A. 2020;796:140003.
- Lin J, Ren W, Wang Q, et al. Influence of grain size and texture on the yield strength of Mg alloys processed by severe plastic deformation. Adv Mater Sci Eng. 2014;2014:356572.
- Indurkar PP, Baweja S, Perez R, et al. Predicting textural variability effects in the anisotropic plasticity and stability of hexagonal metals: application to magnesium and its alloys. Int J Plasticity. 2020;132:102762.
- Mao P, Liu Z, Wang C. Texture effect on high strain rates tension and compression deformation behavior of extruded AM30 alloy. Mater Sci Eng A. 2012;539:13–21.
- Wu G, Yu J, Jia L, et al. Microstructure and texture evolution of Mg-Gd-Y-Zr alloy during reciprocating upsetting-extrusion. Materials (Basel). 2020;13(21):4932.
- Zhao X, Li S, Zheng Y, et al. The microstructure evolution, texture weakening mechanism and mechanical properties of AZ80 Mg alloy processed by repetitive upsetting-extrusion with reduced deformation temperature. J Alloys Compd. 2021;883:160871.
- Meng Y, Yu J, Zhang G, et al. Effect of circumferential strain rate on dynamic recrystallization and texture of Mg-13Gd-4Y-2Zn-0.5Zr alloy during rotary backward extrusion. J Magnes Alloy. 2020;8(4):1228–1237.
- Gautam PC, Biswas S. Effect of ECAP temperature on the microstructure, texture evolution and mechanical properties of pure magnesium. Mater Today Proc. 2021;44:2914–2918.
- Martynenko NS, Lukyanova EA, Serebryany VN, et al. Increasing strength and ductility of magnesium alloy WE43 by equal-channel angular pressing. Mater Sci Eng A. 2018;712:625–629.
- Wang Q, Song J, Jiang B, et al. An investigation on microstructure, texture and formability of AZ31 sheet processed by asymmetric porthole die extrusion. Mater Sci Eng A. 2018;720:85–97.
- Xu J, Jiang B, Kang Y, et al. Tailoring microstructure and texture of Mg–3Al–1Zn alloy sheets through curve extrusion process for achieving low planar anisotropy. J Mater Sci Technol. 2022;113:48–60.
- Chen XM, Chen WZ, Zhang LX, et al. Combined-extrusion techniques for fabricating yield-symmetric ZK61 magnesium alloys rods focusing on texture influence on their mechanical responses. J Mater Process Tech. 2021;297:117236.
- Lin J, Wang Q, Peng L, et al. Microstructure and high tensile ductility of ZK60 magnesium alloy processed by cyclic extrusion and compression. J Alloys Compd. 2009;476(1):441–445.
- Lin J-b, Wang Q-d, Chen Y-j, et al. Microstructure and texture characteristics of ZK60 Mg alloy processed by cyclic extrusion and compression. Trans Nonferrous Met Soc China. 2010;20(11):2081–2085.
- Barnett MR, Keshavarz Z, Beer AG, et al. Influence of grain size on the compressive deformation of wrought Mg–3Al–1Zn. Acta Mater. 2004;52(17):5093–5103.
- Barnett MR, Nave MD, Ghaderi A. Yield point elongation due to twinning in a magnesium alloy. Acta Mater. 2012;60(4):1433–1443.
- Figueiredo RB, Száraz Z, Trojanová Z, et al. Significance of twinning in the anisotropic behavior of a magnesium alloy processed by equal-channel angular pressing. Scripta Mater. 2010;63(5):504–507.
- Barnett MR. Twinning and the ductility of magnesium alloys: Part I: “Tension” twins. Mater Sci Eng A. 2007;464(1):1–7.
- Cepeda-Jiménez CM, Pérez-Prado MT. Microplasticity-based rationalization of the room temperature yield asymmetry in conventional polycrystalline Mg alloys. Acta Mater. 2016;108:304–316.
- Cáceres CH, Lukáč P. Strain hardening behaviour and the Taylor factor of pure magnesium. Philos Mag. 2008;88(7):977–989.
- Barnett MR. A taylor model based description of the proof stress of magnesium AZ31 during hot working. Metall Mater Trans A. 2003;34(9):1799–1806.
- Wang Y, Choo H. Influence of texture on Hall–Petch relationships in an Mg alloy. Acta Mater. 2014;81:83–97.
- Wang HX, Zhang LX, Chen WZ, et al. Improved tension/compression asymmetry achieved in high-strength magnesium alloys via compression-extrusion process. Mater Sci Eng A. 2018;736:239–247.
- Wu WJ, Chen WZ, Zhang LX, et al. Improvement of tension/compression asymmetry for high-performance ZK61 magnesium alloy rod via tailoring deformation parameters: upsetting-extrusion temperature and upsetting ratio. Mater Sci Eng A. 2021;823:141767.
- Cui C, He J, Wang W, et al. Microstructure, texture and mechanical properties of extruded AZ31 Mg alloy during small strain multi-directional forging with gradient cooling. J Alloys Compd. 2022;909:164795.
- Wang HX, Chen WZ, Zhang WC, et al. Microstructure and texture evolution during compression-extrusion process for tension/compression symmetrical ZK61 magnesium alloy rod. Mater Sci Eng A. 2021;806:140807.
- Suh J, Victoria-Hernández J, Letzig D, et al. Enhanced mechanical behavior and reduced mechanical anisotropy of AZ31 Mg alloy sheet processed by ECAP. Mater Sci Eng A. 2016;650:523–529.
- Zhang L, Zhang W, Cao B, et al. Effects of texture and grain size on the yield strength of ZK61 alloy rods processed by cyclic extrusion and compression. Materials (Basel). 2017;10(11):1234.
- Barnett MR, Keshavarz Z, Ma X. A semianalytical sachs model for the flow stress of a magnesium alloy. Metallurgical and Materials Transactions A. 2006;37(7):2283–2293.
- Responsables S. The Oxford engineering science series, The Oxford engineering science series.
- Armstrong RW. Dislocation pile-ups, material strength levels, and thermal activation. Metallurgical and Materials Transactions A. 2016;47(12):5801–5810.
- Armstrong RW. Hall-Petch description of nanopolycrystalline Cu, Ni and Al strength levels and strain rate sensitivities. Philosophical Magazine. 2016;96(29):3097–3108.
- Jain A, Duygulu O, Brown DW, etal. Grain size effects on the tensile properties and deformation mechanisms of a magnesium alloy, AZ31B, sheet. Mater. Sci. Eng. A. 2008;486(1):545–555.
- Yu HH, Xin YC, Wang MY, etal. Hall-Petch relationship in Mg alloys: A review. J Mater Sci Technol. 2018;34(2):248–256.
- Armstrong R, Codd I, Douthwaite RM, etal. The plastic deformation of polycrystalline aggregates. The Philosophical Magazine. 2006;7(73):45–58.
- Guan B, Xin Y, Huang X, etal. Quantitative prediction of texture effect on Hall-Petch slope for magnesium alloys. Acta Mater. 2019;173:142–152.